Abstract
Uca tangeri is a marine fiddler crab found commonly in the West African coast and is often exposed to Gram-negative pathogens upon injury. The aim of this study was to document the patterns of endotoxin-induced protein coagulation and phenoloxidase (PO) activity in hemolymph fractions of Uca tangeri. Hemolymph from live crabs was obtained by carapace puncture, pooled. and then separated into plasma, hemocyte Lysate (HL), hemocyte lysate supernatant (HLS) and hemocyte lysate debris (HLD). The effect of Escherichia coli (O1111:B4) endotoxin and calcium ion (Ca2+) on protein coagulation in the presence/absence of endotoxin and the endotoxin dose-dependence of coagulation and PO activity were each studied in the plasma, HL, HLS and HLD. The results showed Ca2+ was required to induce coagulation, and was endotoxin concentration-dependent in the plasma. PO activity was highest in the HLS but PO specific activity was highest in HLD. PO activity remained relatively constant with increased LPS concentration in the range studied 0–10 EU/ml. From the data we conclude that endotoxin-induced protein coagulation occurs in the plasma alone and might be mediated by trans-glutaminases, while PO activity is localized inside hemocytes and cell membranes in Uca tangeri.
Introduction
Uca tangeri (Eydoux, 1835) is a form of fiddler crab that occurs along the Iberian Peninsula and the West African coast. It is the only species of Uca in Europe (Crane Citation1975). U. tangeri is highly valued and the most consumed crab of high demand in West Africa (Jadamec et al. Citation1999; Enzenross et al. Citation2001; Ojewole and Udom Citation2005). It has been found in literature that the shell and the flesh of U. tangeri are highly protein-rich compared to other crustaceans with protein contents ranging between 17.1–21.31 g/100 g (Ackman Citation1990).
The innate immune system is the first line of inducible host defense against bacterial, fungal, and viral pathogens (Hoebe et al. Citation2004). This system is essential for the survival and perpetuation of all multicellular organisms (Hoffmann et al. Citation1999; Salzet Citation2001). Invertebrates that do not possess immunoglobulins have developed unique means to detect and respond to microbial epitopes like lipopolysaccharides (LPS), lipoteichoic acids, lipoproteins, peptidoglycan, and (1→3)-β-D-glucans (Begum et al. Citation2000; Salawu and Oloyede Citation2011). In invertebrates, toll-like receptor-mediated antimicrobial peptide production (Lemaitre et al. Citation1996; Imler and Hoff-mann Citation2000; Krutzik et al. Citation2001; Underhill and Orinsky, Citation2002), hemolymph coagulation (Iwanaga et al. Citation1978), melanin formation (Sugumaran Citation2002), and lectin-mediated complement activation (Fujita Citation2002) are prominent immune responses. In addition to these enzymatic cascades, a variety of lectins, reactive oxygen-producing cells, and phagocytic systems cooperate to help in the killing of invading pathogens (Bogdan et al. Citation2000).
The hemolymph coagulation phenomenon was first identified as a prominent defense system in the horseshoe crab (Limulus polyphemus) by Bang (Citation1956). When Gram-negative bacteria invade the hemolymph, hemocytes detect LPS molecules on their surfaces (Ariki et al. Citation2004) and then release via rapid exocytosis the contents of L- and S-granules in the hemocytes (Iwanaga Citation1993a,Citationb). The released granular components include two biosensors, factors C and G. These two factors are serine protease zymogens and are auto-catalytically activated by LPS or by (1→3)-β-D-glucan, both major components of cell walls of Gram-negative bacteria and fungi, respectively (Iwanaga and Lee Citation2005).
Iwanaga et al. (Citation1992) and in other studies (Iwanaga Citation1993a,Citationb; Muta and Iwanaga Citation1996a,Citationb) have described in detail the LPS and (1→3)-β-D-glucan-mediated clotting cascades and their molecular structures, and the functions of the five clotting factors, factor C, factor G, factor B, pro-clotting enzyme, and clottable coagulogen, which all participate in clotting cascades. These clotting cascades both involve four serine protease zymogens, factors C, B, G, pro-clotting enzyme, and coagulogen (Bergner et al. Citation1996,Citation1997) in which there is the autocatalytic activation of Factor C to its active form followed by activation/conversion of the other components of the cascade in the presence of LPS or synthetic Lipid A analogs (Iwanaga et al. Citation1992; Tan et al. Citation2000; Kawasaki et al. Citation2000; Iwanaga and Lee Citation2005). Factor G on the other hand is auto- catalytically activated to promote activation and conversion of other parts of another cascade that is stimulated by the presence of (1→3)-β-D-glucan in the absence of any other protein (Muta et al. Citation1995; Takaki et al. Citation2002).
The pro-phenoloxidase (ProPO) system, at the origin of melanin production, has been suggested as an innate defense mechanism in invertebrates (Smith and Soderhall Citation1991). In arthropods, it has been shown that phenoloxidase (PO) is present in hemolymph in an inactive form (ProPO) that is cleaved to PO by proteolysis by an endogenous activator system or exogenous agents. The active form (Soderhall and Cerenius Citation1998) of PO is copper-dependent and catalyzes synthesis of o-diphenols from monophenols by ortho-hydroxylation; these, in turn, are dehydrogenated to o-quinones (Lee et al. Citation1997; Kong et al. Citation1998). Non-enzymatic polymeri-zation of o-diphenols leads to production of melanin, a common response of invertebrates to entry of an infectious agent. The major function of the PO cascade is deposition of pigment melanin that is derived from tyrosine-based substrates like L-3,4-dihydroxyphenyl alanine (L-DOPA) (Hellio et al. Citation2007).
Components of the PO cascade can also opsonize pathogens to enhance their phagocytic uptake; others appear to be fungistatic or cytotoxic (Tujula et al. Citation2001). The activation of the enzymatic system by bacterial or fungal components is an additional clue of the involvement of this enzyme in invertebrate immune defense mechanisms. Many components of the system have recently been isolated in invertebrates, particularly from aquatic arthropods (Aspan et al. Citation1995; Lee et al. Citation2000; Sritunyalucksana et al. Citation2002). It can therefore be seen that LPS not only causes coagulation of protein in a cascade system but also stimulates other immune responses that are enzymatic such as the activation of PO from pro-PO.
To gain further insight into this invertebrate phenomenon for resistance, this study focused on Escherichia coli endotoxin (LPS)-induced coagulation of hemolymph fractions of U. tangeri in the presence of calcium ion (Ca2+), the dependence of protein coagulation on endotoxin concentration, and the activity of PO in hemolymph and at varied endotoxin levels.
Materials and methods
Animals and reagents
Live crabs were purchased from Lekki Peninsula (Lagos, Nigeria) and maintained at ambient temperature of 25 °C. One of the crabs was sent for confirmation of identity at the Department of Zoology of University of Ilorin (Ilorn, Nigeria). The remaining crabs were euthanized upon arrival by carapace puncture (see below). Prior to this, the activeness of the crabs was determined by their ability to protect/prevent themselves from being handled by raising their major chelipeds. Any potentially ‘ill’/moribund crab was discarded from the study.
From a total of 10 crabs, hemolymph collection was carried using protocols outlined below. Eschericha coli endotoxin (Type O1111:B4) was purchased from Sigma (Shanghai, China). All other chemicals and reagents used here were of analytical grade and obtained from the chemical store of the laboratory of the Department of Biochemistry (University of Ilorin).
Extraction of hemolymph from Uca tangeri
Hemolymph from each crab was obtained by dorsi-ventral carapace puncture in an already-washed bowl on ice. The hemolymph was collected and pooled: in these types of studies of components of crustacean hemolymph, pooling of blood of the animals is a common accepted practice (Kopacek et al., Citation1993) and diluted to twice the volume collected using phosphate-buffered saline (PBS, pH 7.4). A combined total of 135 ml hemolymph was obtained from the 10 crabs; this material was diluted to 270 ml using PBS. Samples of this material were used for the entire study.
Fractionation of the hemolymph into plasma, HL, HLS and HLD
The hemolymph sample collected was centrifuged at 1000 × g for 20 min at room temperature. The resulting supernatant (plasma) was collected and stored at –20 °C. The pellet (hemocytes) was washed twice, each time with 1 ml PBS and centrifugation at 1000 × g for 10 min; each time the supernatant was discarded to remove traces of plasma. The pellet was then homogenized in PBS using an ice-cold mortar and pestle. The homogenate was then suspended in PBS to the original volume of hemolymph (now deemed Hemocyte Lysate [HL]). The HL was then centrifuged at 1000 × g for 20 min and the supernatant collected as Hemocyte Lysate Supernatant (HLS) while the remaining pellet was collected as well (now deemed Hemocyte Lysate Debris (HLD). The HLD was washed twice with the 1 ml PBS, centrifuged and then suspended in PBS to the starting volume of the hemolymph. After the various fractions were obtained, the protein contents in each were assessed by the method of Gornall et al. (Citation1949).
Determination of concentrations of some selected metal ions in the plasma
The concentration of some selected metals (i.e. Ca, Mg, K, Mn, Fe, Cu, Co, Pb) in the plasma was determined by Atomic Absorbance Spectrophotometry (AAS) and for Na and K via flame spectrometry at the Chemical Analysis Laboratory at the University of Ibadan, (Ibadan, Nigeria).
Coagulation reactions in U. tangeri hemolymph fractions
Coagulation reactions in the hemolymph fractions were evaluated. In brief, to 0.8 ml of each fraction was added 0.1 ml of 0.1 M CaCl2 and 0.1 ml of LPS (1 EU/ml) in 1.5 ml tubes. The control tubes were prepared in parallel, but lacking either CaCl2 or LPS or both (replaced by 0.1 ml of distilled water instead). In a parallel set-up, 0.6 ml plasma was combined separately with 0.2 ml of either HL or HLS, 0.1 ml of 0.1 M CaCl2 and 0.1 ml of LPS (1 EU/ml) in the tubes. Controls were prepared lacking endotoxin and replaced by 0.1 ml distilled water. Quadruplicates of each mixture were prepared and incubated as stated earlier for the plasma and HL. All the samples were incubated in a water bath at 37 °C for 1 hr and then centrifuged at 800 × g for 10 min. The supernatants obtained were discarded and the pellets in each 1.5 ml tube dissolved in 0.5 ml normal saline solution. Protein concentration in each tube was determined by adding 0.9 ml Biuret reagent to 0.1 ml of dissolved pellet, allowing the mixtures to stand for 20 min at room temperature, and then measuring the absorbance at 550 nm in a Jenway Spectrophotometer (Staffordshire, England). Blanks containing 0.1 ml normal saline solution and 0.9 ml Biuret solution were examined in parallel.
Protein coagulation dependence on LPS concentration
The dependence of the induced protein coagulation on the concentration of endotoxin was determined in the plasma and mixture of plasma/HL. Quadruplicates of each sample containing different LPS concentration were prepared for each LPS concentration. Samples were prepared the same way as in the coagulation reaction at fixed LPS concentration for both plasma and mixture of plasma/HL; however, here, LPS concentrations were varied (0–10 EU/ml) and the control was a sample that lacked LPS but contained distilled water (i.e., 0 EU/ml).
Determination of phenoloxidase (PO) activity in hemolymph fractions
Analysis of PO activity induced by LPS in the hemolymph fractions of U. tangeri using a method based on conversion of L-3,4-dihydoxyphenyl alanine (L-DOPA) to dopachrome as described by Sung et al. (Citation1998), Tanner et al. (Citation2006), and Salawu and Oloyede (Citation2011). In brief, 0.1 ml hemolymph fraction (each, plasma, HL, HLS, or HLD) was combined with 0.2 ml of 5 mM L-DOPA, 1.7 ml bicarbonate buffer (pH 8.0), and 0.1 ml LPS solution (1 EU/ml). Controls were prepared using LPS-free water in place of LPS. All samples were prepared in quadruplicates and incubated at 37 °C throughout the period of investigation. A blank was prepared by using LPS-free water in place of L-DOPA. Absorbance was taken for each sample (at 460 nm) at time 0 (on addition of L-DOPA) and after 10 min (period in which conversion of L-DOPA to dopachrome occurs). The ΔA460 nm/10min were then determined and used to show the PO activity in each sample. Changes in PO specific activity (ΔA460 nm/10 min/mg protein) were then calculated based on the protein content of each fraction used in the experiment.
Statistical analyses
For the coagulation reactions, the results were analyzed using a one-way analysis of variance (ANOVA) followed by a paired t-test in a Prism Software package (GraphPad, San Diego, CA). Values were considered significantly different at p < 0.05.
Results
Measures of metals in the plasma revealed very high concentrations of Na and K, high levels of Ca and Mg, and lesser amounts of Fe and Cu. Other metals such as Mn, Co, Cd, and Pb were not detected in the plasma (). The mean protein measures in the plasma, HL, HLS, and HLD indicated that the plasma had more protein than any of the other fractions. The ranking of protein content was HL > HLS > HLD ().
Table 1. Concentrations of select metals in plasma of Uca tangeri.
Table 2. Protein content of hemolymph fractions.
In the investigations of the amount of protein coagulated in the presence and absence of endotoxin and/or of Ca2+ ion, with the plasma fraction there was endotoxin-induced Ca2+-dependent protein coagulation at 1 EU/ml (). When either component was not present, the level of coagulation was on par with that seen without any LPS or Ca2+. In contrast, with the HL, the same dose of 1 EU/ml alone failed to produce coagulation (; level equal to that seen if neither LPS nor Ca2+ was provided in reaction). However, now, Ca2+ alone could induce coagulation in the HL material. In fact, when Ca2+ was provided in conjunction with the LPS (1 EU/ml), the amount of coagulation observed was nearly identical to that seen with the Ca2+ alone. It is interesting to note that the ‘blank’ (no LPS or Ca2+) levels of coagulation differed greatly in the plasma vs. HL reactions.
Figure 1. Protein coagulation in plasma from Uca tangeri – with or without endotoxin (1 EU/ml) and calcium (0.1 M).
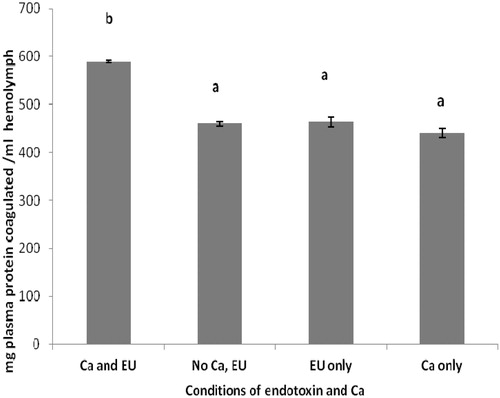
Figure 2. Protein coagulation in HL from Uca tangeri – with/without 1 EU/ml endotoxin or 0.1 M calcium. Values shown are mean ± SEM of four determinations. Bars bearing different letters are significantly different (p < 0.05).

When the plasma was combined with the HL material (), the outcomes in the presence of Ca2+ were on par with those seen with the HL alone treated with LPS and Ca2+ at the original 1 EU/ml endotoxin level. When neither LPS nor Ca2+ was present, levels of coagulation were slightly, but significantly, decreased. This suggests the plasma portion of the mixture was driving the activity seen here (i.e., compare data in vs. to see plasma fraction imparted almost 5-times the levels of coagulation in absence of LPS and Ca2+). Unlike with the HL, when the plasma was combined with the HLS material (), the outcomes in the presence of Ca2+ were significantly reduced vs. those seen with the plasma/HL system. Again, when neither LPS nor Ca2+ was present, the levels of coagulation were slightly, but significantly, decreased.
Figure 3. Endotoxin-induced protein coagulation with the plasma/HL and plasma/HLS hemo-lymph fractions. Values shown are mean ± SEM of four determinations. Bars bearing different letters are significantly different (p < 0.05).
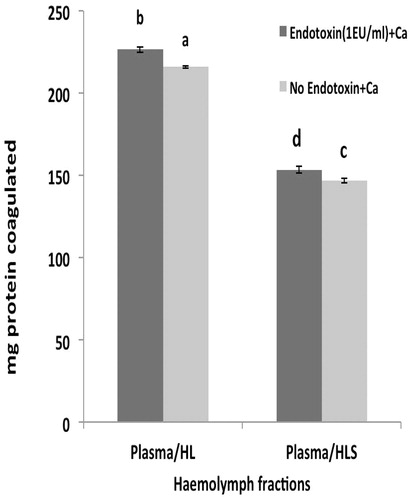
As the plasma fraction had the greatest response to the endotoxin, studies were done to ascertain the dose-relationship between coagulation and LPS level. Analyses of responses over a range of 0-10 EU/ml (in the presence of exogenous Ca2+) indicated a generalized increase – albeit not a response directly correlative to that shown in . Over the dose range, a dose-response relation of y = 16.452x +158.27, r2 = 0.647 was determined. Interestingly, as seen earlier, even in the absence of endotoxin, there was still residual coagulation activity in the preparation. Specifically, at a dose of 0 EU/ml, ≈60 mg protein was coagulated (). While there is certainly Ca present in the plasma itself, there is no source of endotoxin; thus, at this point, it is unclear what is the basis for this high background (actual data presented in Supplemental Table 1a).
When the same dose-relationship determination studies were performed with the plasma/HL mixture (), in this case the response was a generalized decrease (i.e., here, y = −2.9985x + 117.83, r2 = 0.404) as the endotoxin dose increased (actual data presented in Supplemental Table 1b). This was surprising in that there should have been carryover-Ca from the plasma to help drive the coagulation regardless of the LPS level, i.e., at a minimum, the response should have remained flat. This then suggests that with respect to the HL, the presence of LPS can become inhibitory if levels become too great. Further study is required to ascertain more clearly how LPS is acting in this manner with the HL.
Figure 6. Dose-relationship for endotoxin-induced coagulation in Uca tangeri plasma/HL hemolymph fractions mixture.
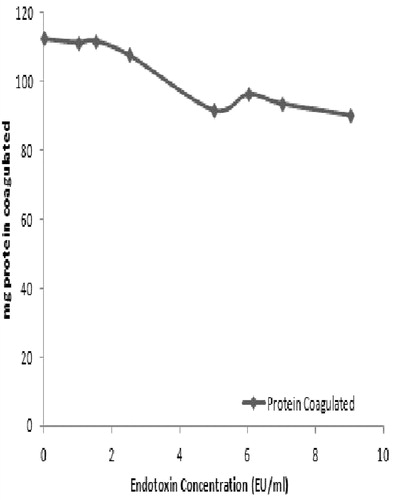
The investigation of phenoloxidase (PO) activity in each fraction showed that PO activity (as specific activity) was highest in the HLD in the presence of endotoxin (1 EU/ml) (
). Levels in all of the fractions were found to rank in the order HLD > HLS > HLD > plasma; in the presence or absence of LPS, these activity levels among the fractions significantly differed. Interestingly, only with the HLS and HLD did the presence of LPS cause signifiant increases in PO activty above background levels. This pattern of PO activities strongly implies that the PO is localized to the HLD (hemocyte cell membrane) and not in the HLS itself.Discussion
The aim of this work was to investigate the coagulation reactions and the phenoloxidase (PO) activity mediated by endotoxin (LPS) in hemolymph fractions of Uca tangeri. The research also attempted to determine the dependence the reactions on endotoxin concentration and calcium (Ca2+) in hemolymph plasma, HL, and mixtures of plasma/HL and plasma/HLS. This was intended to determine the extent to which endotoxin would induce and sustain protein coagulation and PO activity in hemolymph.
Crabs used for this work were collected from the banks of Igbokuta Sea Imota, Ikorodu. Lagos State, Nigeria. The result from the analysis of some metals in the plasma revealed a high amount of sodium and potassium, indicating high amounts of salts (salinity) in the environment of the crab (and thus a marine habitat). The absence of heavy metals such as cadmium and lead indicated that little pollution was present in the habitat of the crab (Olowoyo et al. Citation2010). This might be due to a large expanse of the marine environment that may sufficiently dilute pollutants from onshore thus preventing the accumulation of pollutants in the seawater. The Cu in the plasma could serve as components of some proteins such as phenoloxidase and hemocyanin (Frieden et al. Citation1965).
The protein concentration in each hemolymph fraction indicated that the plasma had the highest mean amount of protein (1056 mg/ml) followed by the HL, HLS with the least amount of protein in the HLD (). This showed that the hemolymph was highly rich in protein and could contribute to the overall high nutritional protein content of these crab (Ozbay and Riley Citation2002). The dependence of coagulation on a presence of Ca2+- that was observed with and without endotoxin in various fractions (plasma, HL, plasma/HL and plasma/HLS) of hemolymph – suggested that coagulation was carried out using Ca2+-dependent enzymes in the HL and plasma fractions. In a Ca2+-free scenario, there was little protein recovered as coagulates. Taken together, the results showed Ca2+ aided protein coagulation responses induced by endotoxin, suggesting coagulation was Ca2+-dependent process and likely to involve specific Ca2+-dependent transgluta-minases (Soderhall and Cerenius Citation1992). On the other hand, the HL studies indicated a signifi-cant amount of coagulation occurred in the absence of endotoxin but only if Ca2+ was present. This signified the coagulation process in Uca tangeri hemocytes was Ca2+-dependent/endotoxin-independent.
A dependence of coagulation on Ca2+ was also noted in mixtures containing Uca tangeri plasma/HL and plasma/HLS - in the presence and absence of endotoxin. Thus, we concluded that Ca2+ played a major role in hemolymph coagulation and that the process was subject to modulation by endotoxin. Like this Uca tangeri plasma endotoxin-induced Ca2+-dependent protein coagulation, a similar effect was reported in Sudanonautes africanus freshwater crabs (Salawu and Oloyede Citation2011). However, contrary to the findings with S. africanus, the cellular components of Uca tangeri could coagulate upon signaling by Ca2+, independent of endotoxin. Why these two species should differ in this manner remains to be determined.
Phenoloxidase (PO) activity in the hemolymph fractions of Uca tangeri was significantly higher in the HLS than in any of the other fractions evaluated. This result suggested a role for hemocytes in limiting the growth/survival of Gram-negative bacteria upon entry into hemolymph. Specific activity of PO in each fraction reflected the fact the enzyme should be more concentrated in the hemocyte membranes (and thus in the HLD) than in any other hemolymph fraction. This would be in keeping with a need for PO to be more highly concentrated at the cell surface compared to other proteins that may exist on the cell surface (Tanner et al. Citation2006). Because one product of PO activity (dopachrome) and the actual end product melanin have been reported to be toxic to invading pathogens, localization of PO to the cell surface provides an optimal opportunity for fending off Gram-negative bacteria and other pathogens that are routinely found in the waters of major towns/cities in tropical climates where this crab resides.
While there were noted differences in coagulation process specifics for the Uca tangeri vs. that in another freshwater crab, in many ways the biology of the PO in this crab was more like that observed in several other crustaceans. For example, Terwilliger and colleagues (Terwillinger and Ryan, Citation2006; Terwillinger et al., Citation2006) noted during assessments of PO and hemocyanin (a PPO phylogenetically related to PO) isolated from hemolymph of the brachyuran crab Cancer magister that both PO and hemocyanin in hemocytes converted diphenols to O-quinones. Similarly, PO activity in hemolymph of spiny lobsters (Panulirus argus) was due to a presence of PPO in hemocytes [and hemocyanin] in the plasma (Perdomo-Morales et al., Citation2007).
It is not a coincidence that the product of PO activity, melanin, plays many roles in invertebrates apart from contributing to pigmentation. Several studies have shown that injury or the presence of nematodes/parasitoids results in melanin deposition (melanization) around the damaged tissue or invading object (Nappi and Christensen, Citation2005). In arthropods, melanization starts when the foreign target is located by hemocytes via a signaling cascade involving LPS, or even peptidoglycans, glucans, and their recognition proteins (Strand, Citation2008). Hemocytes surround the foreign body and release chemoattractants to recruit plasmatocytes (Lavine and Strand, Citation2002) that, in turn, form a multi-cellular plasmatocyte wall (Wood et al., Citation2006). The internal layers of the plasmatocyte wall thicken and darken due to melanin production; ultimately, the melanin is deposited onto the target and this capsule then prevents the growth/reproduction/survival of the pathogen (Gillespie et al., Citation1997). Because this endproduct of PO activity is toxic to invading pathogens, localization of PO to the cell surface provides an optimal opportunity for fending off Gram-negative bacteria and other pathogens that are routinely found in the waters of major towns/cities in tropical climates where this crab resides.
Conclusion
The results here indicated that endotoxin-induced protein coagulation in Uca tangeri is localized in the plasma and is concentration-dependent. Further, the study showed endotoxin triggered phenoloxidase (PO) activity primarily in the HLD and HLS fractions. This primary localization of PO activity to the hemocytes is in keeping with the expected role of melanins in contributing to the defense of Uca tangeri against potential invading Gram-negative bacteria due to injury or exposure in polluted environments.
Supplemental_Tables.docx
Download MS Word (30.1 KB)Supplemental_files_for_IMT_13-3-16-18_UIMT_2015-0082_FINAL_.doc
Download MS Word (46 KB)Acknowledgements
The contributions to this work of Dada Michael Friday, Ope-ewe Oludayo Oluwaseyi and other students supervised by Dr. M.O. Salawu in the 2014/2015 Session are highly appreciated.
Disclosure statement
The authors declare no conflicts of interest. The authors alone are responsible for the content of this manuscript.
References
- Ackman RG. 1990. Seafood lipids and fatty acids. Food Rev Intl. 6:617–646.
- Ariki S, Koori K, Osaki T, Motoyama K, Inamori K, Kawabata S. 2004. A serine protease zymogen functions as a pattern-recognition receptor for lipopolysaccharides. Proc Natl Acad Sci USA. 101:953–958.
- Aspan A, Sheng Huang T, Cerenius L, Soderhall K. 1995. cDNA cloning of pro-PO from the freshwater crayfish Pacifastacus leniusculus and its activation. Proc Natl Acad Sci USA. 92:939–943.
- Bang FB. 1956. A bacterial disease of Limulus polyphemus. Bull Johns Hopkins Hosp. 98:325–351.
- Begum N, Matsumoto M, Tsuji S, Toyoshima K, Seya T. 2000. The primary host defense system across humans, flies, and plants. Curr Trends Immunol. 3:59–74.
- Bergner A, Muta T, Iwanaga S, Beisel HG, Delotto R, Bode W. 1997. Horseshoe crab coagulogen is an invertebrate protein with a nerve growth factor-like domain. Biol Chem. 378:283–287.
- Bergner A, Oganessyan V, Muta T, Iwanaga S, Typke D, Huber R, Bode W. 1996. Crystral structure of a coagulogen, the clotting protein from horseshoe crab: A structural homologue of nerve growth factor. EMBO J. 15:6789–6797.
- Bogdan C, Röllinghoff M and Diefenbach A. 2000. Reactive oxygen and reactive nitrogen intermediates in innate and specific immunity. Curr Opin Immunol. 12:64–76.
- Crane JH, editors. 1975. Fiddler crabs of the world: Ocypodidae, genus Uca. Princeton, NJ: Princeton University Press.
- Enzenross R, Enzenross L, Bingel F, 2001. Occurrence of blue crab, Calinectes sapidus (Rathbun) (Crusteceae, Brachyura) on the Turkish Mediterranean adjacent to Aegean coast and its size distribution in the bay of Iskenderun. Turk J. 21:113–122
- Frieden E, Osaki S, Kobayashi H. 1965. Copper proteins and oxygen: Correlations between structure and function of the copper oxidases. J Gen Physiol. 1965:213–251.
- Fujita T. 2002. Evolution of the lectin-complement pathway and its role in innate immunity. Nat Rev Immunol. 2:346–353.
- Gillespie JP, Kanost MR, Trenczek T. 1997. Biological mediators of insect immunity. Annu Rev Entomol. 42:611–643
- Gornall AG, Bardawill CJ, David MM. 1949. Determination of serum proteins by means of the biuret reaction. J Biol Chem. 177:751–766.
- Hellio C, Bado-Nilles A, Gagnaire B, Renault T and Thomas-Guyon H. 2007. Demonstra-tion of a true phenoloxidase activity and activation of a ProPO cascade in Pacific oyster, Crassostrea gigas (Thunberg) in vitro. Fish Shellfish Immunol. 22:433–440.
- Hoebe K, Jansen E, Beutler B. 2004. Interface between innate and adaptive immunity. Nat Immunol. 5:971–974.
- Hoffmann JA, Kafatos FC, Janeway CA Jr., Ezekowitz, RA. 1999. Phylogenetic perspectives in innate immunity. Science 284:1313–1318.
- Imler JL, Hoffmann JA. 2000. Signaling mechanisms in the antimicrobial host defense of Drosophila. Curr Opin Microbiol. 3:16–22.
- Iwanaga S, Lee. BL. 2005. Recent advances in the innate immunity of invertebrate animals. J Biochem Mol Biol. 38:128–150.
- Iwanaga S. 1993a. Primitive coagulation systems and their message to modem biology. Thromb Hemost. 70:48–55.
- Iwanaga S. 1993b. The limulus clotting reaction. Curr Opin Immunol. 5:74–82.
- Iwanaga S, Miyata T, Tokunaga F and Muta T. 1992. Molecular mechanism of hemolymph clotting system in Limulus. Thrombosis Res. 68:1–32.
- Iwanaga S, Morita T, Harada T, Niwa M, Takada K, Kimura T, Sakakibara S. 1978 Chromogenic substrates for horseshoe crab clotting enzyme. Its application for the assay of bacterial endotoxins. Hemostasis 7:183–188.
- Jadamec LS, Donaldson WE, Cullenburg P. 1999. Biological Field Techniques for Chionocetes Crabs. University of Alaska Sea Grant College Program University of Alaska, Alaska.
- Kawasaki H, Nose T, Muta T, Iwanaga S, Shimohigashi Y and Kawabata S. 2000. Head-to-tail polymerization of coagulin, a clottable protein of the horseshoe crab. J Biol Chem. 275:35297–35301.
- Kong KH, Lee JL, Park HJ, Chos SH. 1998. Purification and characterization of the tyrosinase isozymes of pine needles. Biochem Mol Biol Intl. 45:717–724.
- Kopacek P, Hall M, Söderhäll K. 1993. Characterization of clotting protein, isolated from plasma of the freshwater crayfish Pacifastacus leniuscus. Eur Biochem. 213:591–597
- Krutzik SR, Sieling PA, Modlin RL. 2001. Role of toll-like receptors in host defense against microbial infection. Curr Opin Immunol. 13:104–108.
- Lavine MD, Strand MR. 2002. Insect hemocytes and their role in immunity. Insect Biochem Mol Biol. 32:1295–1309
- Lee JL, Kong KH, Cho SH. 1997. Purification and characterization of tyrosinase from Solanum melongena. J Biochem Mol Biol. 30:150–156.
- Lee SY, Wang RG, Soderhall K. 2000. A lipopolysaccharide- and β-1,3-glucan-binding protein from hemocytes of the freshwater crayfish Pacifastacus leniusculus. J Biol Chem. 275:1337–1343.
- Lemaitre B, Nicolas E, Michaut L, Reichhart JM, Hoffmann JA. 1996. The dorsoventral regulatory gene cassette spätzle/Toll/cactus controls the potent antifungal response in Drosophila adults. Cell. 86:973–983.
- Muta T and Iwanaga S. 1996a. Clotting and immune defense in Limulidae. Prog Mol Subcell Biol. 15:154–189.
- Muta T, Iwanaga S. 1996b. The role of hemolymph coagulation in innate immunity. Curr Opin Immunol. 8:41–47.
- Muta T, Seki N, Takaki Y, Hashimoto R, Oda T, Iwanaga A, Tokunaga F, Iwanaga S. 1995. Purified horseshoe crab factor G. Re-constitution and characterization of the (1→3)-β-D-glucan-sensitive serine protease cascade. J Biol Chem. 270:892–897.
- Nappi AJ, Christensen BM. 2005. Melanogenesis and associated cytotoxic reactions: applications to insect innate immunity. Insect Biochem Mol Biol. 35:443–459
- Ojewole GS, Udom SF. 2005. Chemical evaluation of the nutrient composition of some unconventional animal protein sources. Intl J Poult Sci. 4;745–747.
- Olowoyo DN, Ajayi OO, Amoo IA, Ayeisanmi AF. 2010. Seasonal variation of metal concentrations in catfish, blue crab and crayfish from Warri Coastal Water of Delta State, Nigeria. Pakistan J Nutr. 9:1118–1121.
- Ozbay G, Riley JG. 2002. An analysis of refractometry as a method of determining blood total protein concentration in the American lobster Homarus americanus (Milne Edwards). Aqua Res. 33:557–562.
- Perdomo-Morales R, Montero-Alejo V, Perera E, Pardo-Ruiz Z, Alonso-Jimenez E. 2008. Hemocyanin-derived phenoloxidase activity in the spiny lobster Panulirus argus (Latreille, 1804). Biochim Biophys Acta. 1780:652–658
- Salawu MO, Oloyede BO. 2011. Endotoxin-induced coagulation reactions and phenol-oxidase activity modulation in Sudanonautes africanus hemolymph fractions. J Immunotoxicol. 8:324–332
- Salzet M. 2001 Vertebrate innate immunity resembles a mosaic of invertebrate immune responses. Trends Immunol. 22:285–288.
- Smith VJ, Soderhall K. 1991. Comparison of phenoloxidase activity in the blood of marine invertebrates. Dev Comp Immunol. 15:251–261.
- Soderhall K, Cerenius L. 1998. Role of pro-phenoloxidase-activating system in invertebrate immunity. Curr Opin Immunol. 10:23–28.
- Söderhäll K, Cerenius L. 1992. Crustacean immunity. Ann Rev Fish Dis. 2:3–23.
- Sritunyalucksana K, Lee SY, Soderhall K. 2002. A β-1,3-glucan binding protein from the black tiger shrimp, Penaeus monodon. Dev Comp Immunol. 26:237–245.
- Strand MR. 2008. The insect cellular immune response. Insect Sci. 15:1–14
- Sugumaran M. 2002. Comparative biochemistry of eumelanogenesis and the protective roles of phenoloxidase and melanin in insects. Pigment Cell Res. 15:2–9.
- Sung HH, Chang HJ, Her CH, Chang JC, Song YL. 1998. Phenoloxidase activity of hemocytes derived from Penaeus monodon and Macrobrachium rosenbergii. J Invertebr Pathol. 71:26–33.
- Takaki Y, Seki N, Kawabata S, Iwanaga S, Muta T. 2002. Duplicated binding sites for (1→3)-β-D-glucan in the horseshoe crab coagulation factor G: Implications for a molecular basis of the pattern recognition in innate immunity. J Biol Chem. 277:14281–14287.
- Tan NS, Ho B, Ding JL. 2000. High-affinity LPS binding domain(s) in recombinant Factor C of a horseshoe crab neutralizes LPS-induced lethality. FASEB J. 14:859–870.
- Tan NS, Ng ML, Yau YH, Chong PK, Ho B, Ding, JL. 2000. Definition of endotoxin binding sites in horseshoe crab Factor C recombinant sushi proteins and neutralization of endotoxin by sushi peptides. FASEB J. 14:1801–1813.
- Tanner CA, Burnett LE, Burnett KG. 2006. Effects of hypoxia and pH on phenoloxidase activity in the Atlantic blue crab Callinectes sapidus. Comp Biochem Physiol Part A 144:218–223.
- Terwilliger NB, Ryan MC. 2006. Functional and phylogenetic analyses of phenoloxidase from the brachyuran (Cancer magister) and branchiopod (Artemia franciscana, Triops longicaudatus) crustaceans. Biol Bull. 210:38–50
- Terwilliger NB, Ryan MC, Phillips MR. 2006. Crustacean hemocyanin gene family and microarray studies of expression change during eco-physiological stress. Integr Comp Biol. 46:991–999
- Tujula N, Radford J, Nair SV, Raftos DA. 2001. Effects of tributyltin and other metals on the phenoloxidase activating system of the tunicate, Styela plicata. Aquat Toxicol. 55:191–201.
- Underhill DM, Orinsky A. 2002. Toll-like receptors: Key mediators of microbe detection. Curr Opin Immunol. 14:103–110.
- Wood W, Faria C, Jacinto A. 2006. Distinct mechanisms regulate hemocyte chemotaxis during development and wound healing in Drosophila melanogaster. J Cell Biol. 173:405–416