Abstract
Aluminum oxide nanoparticles (AlO NP) have been widely utilized in a variety of areas, including in the optical, biomedical and electronic fields and in the overall development of nanotechnologies. However, their toxicological profiles are still not fully developed. This study compared the distribution and immunotoxicity of two rod-types of AlO NP. As reported previously, the two types of AlO NP had different aspect ratios (long-type: 6.2 ± 0.6, short-type: 2.1 ± 0.4), but the size and surface charge were very similar. On Day 14 after a single intravenous (IV) injection (1.25 or 5 mg/kg), both AlO NP accumulated primarily in the liver and spleen and altered the levels of redox response-related elements. The accumulated level was higher in mice exposed to the long-type AlO NP compared to the short-type. Additionally, it was noted that the levels of IL-1β, IL-8 and MCP-1 were enhanced in the blood of mice exposed to both types of AlO NP and the percentages of neutrophils and monocytes among all white blood cells were increased only in mice injected with the long-type AlO NP (5 mg/kg). In addition, as compared to the control, co-expression of CD80 and CD86 (necessary for antigen presentation) on splenocytes together with a decreased expression of chemotaxis-related marker (CD195) was attenuated by exposure to the AlO NP, especially the long-type. Taken together, the data suggest that accumulation following a single IV injection with rod-types of AlO NP is strengthened by a high aspect ratio and, subsequently, this accumulation has the potential to influence immune functions in an exposed host.
Introduction
Nanotechnology is a fast-growing and promising innovation. Because of their small size, nanoparticles exhibit distinct physicochemical properties such as structural stability, chemical reactivity, electrical conductance, magnetism and optical effects compared to the conventional micron-sized particles (Borm et al. Citation2006; Dykman and Khlebtsov Citation2012; Cheng et al. Citation2013; Braakhuis et al. Citation2014). Aluminum oxide nanoparticles (AlO NP) are thermodynamically stable over a wide temperature range and have a corundum-like structure with oxygen atoms and Al3+ ions (Tavakoli et al. Citation2013). AlO NP are also highly effective catalysts and carry a positive charge on their surfaces at near-neutral pH; thus, they have been used as catalysts in propellants and pyro-techniques (Ghanta and Muralidharan Citation2013). Moreover, AlO NP have been widely studied over the past 10 years in a wide range of applications, including dispersion-strengthening, nanocomposites, catalyst support, transparent conductive coatings, biomaterials, food processing, drug delivery, transparent optical coatings, wear-resistant additives and material surface coating (Mukherjee et al. Citation2006; Ravishankar and Jamuna Citation2011; Willhite et al. Citation2014). At the same time, while concerns about potential risks have been raised with the increasing use of AlO NP, their toxicological profile is still unclear (Oesterling et al. Citation2008; Park, Lee, et al. Citation2015).
ROS are immunologically active and their generation facilitates transfer of NP into other tissues through damage to cell membranes (Baud and Ardaillou Citation1993; Saeidnia and Abdollahi Citation2013; Gomes et al. Citation2014; Mangge et al. Citation2014). Previous studies reported that AlO NP could induce oxidative stress via increased generation of reactive oxygen species (ROS) (Li et al. Citation2012; Prabhakar et al. Citation2012; Morsy et al. Citation2013a). Nanoparticles have also been associated with induction of autoimmune diseases, such as irritable bowel syndrome, Crohn’s disease and Type 1 diabetes (Petrarca et al. Citation2006; Borgognoni et al. Citation2015; Junnila Citation2015). In addition, accumulated data has suggested that nanoparticles that enter the body via the respiratory system, skin or gastrointestinal tract can translocate to other tissues via various transfer routes/mechanisms and that their distribution and toxicity depend on their secondary properties in biocompatible fluids and behaviors in bodily fluids as well as their primary properties (Oberdörster et al. Citation2005; Li et al. Citation2009; Roy et al. Citation2014; Bruinink et al. Citation2015). Further, reports have noted that experimental conditions, such as time, dose and exposure routes, could influence biodistribution and toxicity following nanoparticle exposure (Staskal et al. Citation2005; Morsy et al. Citation2013b; Geraets et al. Citation2014).
In previous studies in our laboratories, a no-observed adverse effect level (NOAEL) following oral administration of rod-type AlO NP for 13 weeks was estimated to be <6 mg/kg (Park et al. Citation2014). We also showed that the toxicity of AlO NP was affected by their aspect ratios in vivo and in vitro (Park, Shim, et al. Citation2015). The present study was undertaken to ascertain the role of aspect ratios of AlO NP in immunotoxic responses. In this study, mice were injected intravenously with one of two forms of commercially available rod-types AlO NP (at 1.25 or 5 mg/kg) and the distribution and immunotoxicity of the AlO NP were assessed 14 days later. Changes in homeostasis of redox response-related elements following accumulation of AlO NP as well as changes in cell (i.e. splenocyte) biology were also assessed to examine potential induction of systemic immunotoxic responses from the single exposure.
Materials and methods
Preparation of AlO NP
Two types of AlO NP were purchased (Cat #544833 and Cat # 718475; Sigma, St. Louis, MO) and they were suspended in deionized water (DW) at 2 mg/ml. The suspensions were sonicated in a bath-type sonicator (150 W, 40 KHz) for 3 h to disperse each AlO NP in a stable fashion (Park, Lee, et al. Citation2015). The sonicator temperature was maintained at <30°C to prevent agglomeration. The surface charge and hydrodynamic length in biocompatible fluids (DW and fetal bovine serum [FBS, HyClone, GE Healthcare Life Science, Seoul, Korea]) were characterized using a ELSZ-2 Zeta Potential and Particle Size Analyzer (Otsuka Electronics Korea Co. Ltd., Seongnam, Korea).
Animals
ICR mice (male, 27–28 g, 6-weeks-of-age) were obtained from OrientBio (Seongnam, Korea) and housed in a pathogen-free facility (23 [± 3]°C, relative humidity of 50 [± 10]%, 12-h light/dark cycle [150–300 lx] intensity), with ventilation exchanges of 10–20 times/h. All mice had ad libitum access to standard rodent chow and filtered tap water. The Institutional Animal Care and Committee (IACUC, #2013-0059) of Ajou University (Suwon, Korea) approved all aspects of these studies. All studies were performed in accordance with the ‘Guide for the Care and Use of Laboratory Animals’, an ILAR publication.
AlO NP treatment
All mice were acclimatized for 1 week before the start of the study. After acclimation, mice were randomly allocated into various treatment groups (n = 10 mice/exposure group). A single dose (either 1.25 or 5.0 mg/kg) of AlO NP (short-type or long-type depending on group) was injected via the tail vein of each mouse in the respective AlO NP groups. The doses used in this study were selected based upon a NOAEL value for 13 week-repeated oral administrations in our previous study (Park et al. Citation2014). However, as there is no proper way to simply translate oral vs intravenous toxicity for any given agent, a pilot study using these dosing regimens was performed. Those results showed there was no overt mortality or morbidity induced by the treatments used here (data not shown).
After the injection, slight pressure was applied at the puncture site by using a dry piece of gauze until bleeding stopped; the mice were monitored for 5–10 minutes to ensure hemostasis. Control mice were injected with DW (≈80–90 μl; 5 ml/kg) according to standard operating procedures of the Korea Institute of Toxicology, an institute in compliance with principles of good laboratory practices. Mice were euthanized by CO2 gas on Day 14 after the single injection according to the Organization for Economic Co-operation and Development guidelines for testing of chemicals (Method #417).
Metal ion measures in tissues
At necropsy, the brain, kidney, spleen, heart, thymus, liver, lungs and testis from each mouse was recovered and weighed. Samples of blood were also collected from the saphenous vein. The tissues were digested to determine the levels of metal ions using a mixture of HNO3 (70%, 7 ml) and H2O2 (35%, 1 ml) in a microwave digestion system (Milestone, Sorisole, Italy) under high temperature (120 °C, 8 min; 50 °C, 2 min; 180 °C, 10 min) and high pressure. Element concentrations in lysates were then measured using inductively coupled plasma mass spectrometry (ICP-MS) at the Korean Basic Science Institute (see Supplement 1).
Hematologic and biochemical analyses
Hematologic analyses of the whole blood samples were conducted using a blood auto-analyzer (HemaVet850, CDC Technologies, Dayton, OH). To assess biochemical parameters, an aliquot of each whole blood sample was centrifuged at 3000 rpm for 10 min to obtain serum and parameters, including total protein, albumin, glucose, aspartate aminotransferase (AST), alanine aminotransferase (ALT), alkaline phosphatase (ALP), creatinine, blood urea nitrogen (BUN), γ-glutamyl transferase (γGT) and lactate dehydrogenase (LDH) were then measured using a 7180 auto-analyzer (Hitachi, Tokyo, Japan).
Histopathology
Samples of tissues (brain, kidney, spleen, heart, thymus, liver and lungs) were placed in 10% neutral buffered formalin; testes were fixed in Bouin’s solution. After paraffin embedding, 3-μm sections of each were cut and then stained with hematoxylin and eosin. The presence of histopathological lesions was then evaluated in a blinded manner following the standard operating procedures of the Korea Institute of Toxicology (Daejeon, Korea).
Cytokine assay
Concentrations of pro-inflammatory cytokines/chemokines including interleukin (IL)-1β, tumor necrosis factor (TNF)-α, IL-6, chemokines macrophage inflammatory protein (MIP)-1α, monocyte chemotactic protein (MCP)-1 and IL-8, as well as of immunoglobulin (Ig)-E were determined using commercially available ELISA kits (all eBioscience [San Diego, CA] except for kit for IL-8: Cusabio [Wuhan, Hubei, China]), according to manufacturer instructions. Following the assay completion, the absorbance in each well was evaluated at 450 nm on a 96-well ELISA plate reader (Molecular Devices, Sunnyvale, CA). The concentrations of each cytokine were extrapolated from standard curves generated in parallel using kit-provided standards. The level of sensitivity for each cytokine/chemokine ELISA kit was 500 pg/ml and 1 ng/ml for the IgE kit.
Fluorescence-activated cell sorting analysis
Splenocytes were isolated from spleens of mice in RPMI media containing 2% FBS. After removing red cells by incubation in fluorescence-activated cell sorting (FACS) lysis buffer, the splenocytes were filtered through a 70 μm-pore size nylon mesh and then re-suspended in FACS buffer. After blocking with Fc-block antibody to reduce non-specific binding (eBioscience), the splenocytes were incubated all at one time with fluorescein isothiocyanate (FITC)-conjugated anti-CD80 (clone B7-1, eBioscience), peridinin chlorophyll (PerCP)-conjugated anti-CD195 (eBioscience) and allophycocyanin (APC)-conjugated anti-CD86 (clone B7-2; BioLegend, San Diego, CA) antibodies for 30 min at 4°C according to manufacturer instructions. The cells were then washed twice with FACS buffer and then analyzed in a FACS Calibur flow cytometer with CellQuest software (BD Biosciences, Franklin Lakes, NJ). A minimum of 10 000 events/sample was acquired.
Statistical analysis
Statistical analyses were performed using a Student’s t-test (GraphPad Software, San Diego, CA) and a one-way analysis of variance (ANOVA) followed by a Tukey’s post-hoc comparison. A p-value <0.05 was considered statistically significant. All data were expressed as mean ± standard deviation (SD), unless otherwise noted.
Results
Characterization of AlO NP
After suspension and sonication of both AlO NP, the dispersion stability of each was confirmed in the water suspension – even after 3 h (Supplement 2A). Both AlO NP exhibited rod-shaped morphologies with different aspect ratios (length-to-diameter) as previously reported (Park, Lee, et al. Citation2015). The aspect ratios of the AlO NP were 6.2 [± 0.6] (hereafter referred to as long-AlO NP) and 2.1 [± 0.4] (hereafter referred to as short-AlO NP). In addition, the Brunauer–Emmett–Teller (BET) specific surface areas (SSA) of the long- and short-AlO NP were 163 and 106 m2/g, respectively. Hydrodynamic diameters and surface charges of both AlO NP in DW and FBS were similar ().
Table 1. Physicochemical characteristics of aluminum oxide nanoparticles (AlO NP).
Distribution of Al and redox response-related elements
At 14 days after the single IV injection of the AlO NP, the short-type AlO NP had significantly accumulated in the liver and spleen, whereas the long-type AlO NP accumulated in the brain, heart, lung, kidney, liver and spleen (at 5 mg/kg dose) (). Further, the accumulated levels were higher in mice injected with the long-type AlO NP compared to the short-type. Moreover, levels of copper (Cu; Supplement 3A) and manganese (Mn; Supplement 3B) were significantly decreased in the brain, lung and spleen (Cu) and in the brain (Mn) of mice treated with either AlO NP. Meanwhile, zinc (Zn) levels were markedly increased in the lung and spleen of mice injected with the long-type AlO NP (Supplement 3C). As compared to the control mice values, iron (Fe) levels were notably lower in the heart, kidneys and liver of the short-type AlO NP-exposed mice and in the heart, kidney and testes of long-type AlO NP-exposed mice (Supplement 3D). Cobalt (Co) levels also were decreased in kidneys of mice administered either AlO NP (Supplement 3E).
Table 2. Al levels in tissues (ng/g), feces (ng/g) and blood (ng/μl).
Hematological and biochemical changes following accumulation of AlO NP
As shown in , the short-type AlO NP had no significant effects on any of the measured parameters, except for causing significant (dose-independent) reductions in the percentages of leukocytes among all the white blood cells (WBC). On the other hand, the lower dose (1.25 mg/kg) of the long-type NP caused significant increases in host numbers of WBC; no other measured parameter was significantly affected. With the higher 5 mg/kg dose of the long-type AlO NP, among all the endpoints evaluated, only the percentages of neutrophils and monocytes among all WBC were significantly elevated in the treated mice. Furthermore, among all the various biochemical endpoints evaluated here, no significant biochemical changes were induced by treatment with either dose/form of the AlO NP ().
Table 3. Hematological changes in mice administered AlO NP.
Table 4. Biochemical changes in mice administered AlO NP.
Histopathological changes following accumulation of AlO NP
Long-type AlO NP induced infiltration of inflammatory cells into the liver (). Short-type AlO NP did not elicit any significant changes in the liver, despite the clearly elevated Al levels found at the site. Additionally, no remarkable pathological changes were observed in the brain, kidney, spleen, heart, thymus or lung of mice injected with both AlO NP (data not shown).
Immunotoxic responses following injection of AlO NP
Serum levels of IL-8 and MCP-1 were significantly elevated in mice 14 days after being injected with either dose of the long-type AlO NP; IL-8 and MCP-1 were significantly elevated only with the higher dose (5 mg/kg) of the short-type AlO NP (). IL-1β levels were only increased significantly following injection of the long-type AlO NP at 5 mg/kg. There were no significant changes in serum TNFα, IL-6, MIP-1 or IgE levels due to the injection of either AlO NP at either test dose (data not shown).
Figure 2. Serum levels of pro-inflammatory mediators. Whole blood was collected on Day 14 after the single injection. n = 5 mice/group. *p < 0.05; **p < 0.01 vs control level.
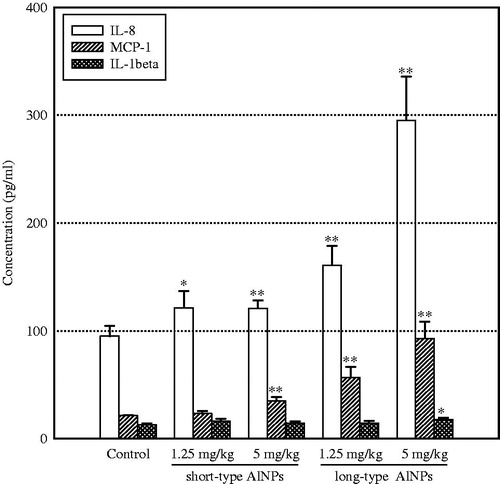
With regard to expression of CD80, CD86 and CD195, levels of each marker were significantly decreased on splenocytes from mice injected with the higher dose (5 mg/kg) of the long-type AlO NP (). Injection with 5 mg/kg of the short-type AlO NP only significantly impacted on CD86 and CD195 levels. The lower dose of either rod-type had nominal effects on expression of these markers; only CD195 levels were significantly lowered with the 1.25 mg/kg dose of the long-type AlO NP.
Figure 3. Changes in expression of immune response-related surface makers. Splenocytes isolated on Day 14 after the single injection were stained with conjugated monoclonal antibody for the indicated marker and then underwent FACS analysis. A total of 10 000 cells/sample was acquired. Values shown are means ± SD. n = 5 mice/group. *p < 0.05, **p < 0.01 vs control level.
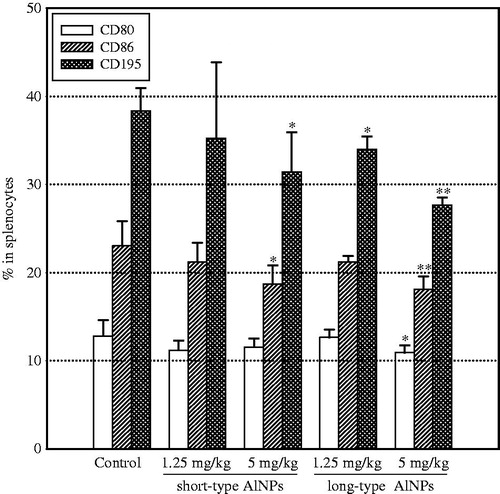
Discussion
Although a number of studies have been devoted to identifying relationships between properties of nanoparticles and their toxicity and biodistribution, the effects of parameters determining their toxicity and biodistribution are not fully understood in vivo. Nanoparticles exhibit unique physicochemical properties according to their manufacturing processes, even with the raw materials being the same. Therefore, findings obtained from the analysis of soluble chemicals cannot be extrapolated to insoluble nanoparticles, despite the fact many are composed of classic chemical elements (Willhite et al. Citation2014).
Accumulated evidence also suggests that nanoparticles elicit toxicities that differ from that of micro-sized particles, because of their smaller size and larger surface area. Similarly, some research has shown that manufactured nanoparticles induce notably different toxicity from that caused by naturally-occurring particles, due in part to their high reactivity and greater ability to cross biological barriers (Li et al. Citation2008). In addition, properties of manufactured nanoparticles (primary properties) can be altered in biological conditions, including proteins, pH and ions (secondary properties), and interaction between nanoparticles and biological systems can be influenced by both primary and secondary properties (Nel et al. Citation2009; Johnston et al. Citation2012; Saeidnia et al. 2013; Vereda et al. Citation2015). Furthermore, experimental conditions, such as time, dose and exposure route influence their biodistribution and toxicity (Staskal et al. Citation2005; Morsy et al. Citation2013b; Geraets et al. Citation2014). For example, because NP are easily affected in low pH conditions, their presence in a site like the gastrointestinal system (re: stomach and gastric acid) could ultimately impact on ultimate toxicity in situ following exposure by the oral route.
Nanoparticles may be distributed to the colon, lungs, bone marrow, liver, spleen and the lymphatic system after injection and removed from the systemic circulation predominantly by the actions of hepatic and splenic macrophages (Moghimi et al. Citation2005; Hagens et al. Citation2007). In this process, the particles can be trapped by macrophages and so remain for a long time in the spleen and liver (Cho et al. Citation2009). In our previous study, long-type AlO NP distributed to the liver, kidney, heart, lung and thymus on Day 90 after a repeated oral dosing (Park, Shim, et al. Citation2015) and – when instilled intratracheally – these long-type AlO NP remained in the lungs to a greater extent and induced more severe lesions compared to the effects from the short-type AlO NP (Park, Lee, et al. Citation2015). In the current study, secondary properties of both AlO NP in DW and FBS, including size, size distribution and surface charge, were very similar and they accumulated mostly in the liver and spleen on Day 14 post-injection. However, the accumulated levels were higher in mice injected with long-type AlO NP relative to in mice that received the short-type AlO NP, especially in the liver, lung, kidneys, and spleen. This indicated that AlO NP with higher aspect ratios might accumulate to greater degrees in the body. Moreover, the liver and spleen may be the main target organs of intravenously injected AlO NP.
Metallic nanoparticles are known to induce oxidative stress by increasing reactive oxygen species (ROS) levels and to alter anti-oxidant enzyme activities, such as those of Cu-, Zn- and Mn-superoxide dismutase (SOD, Shrivastava et al. Citation2014). SOD acts as an anti-oxidant by catalyzing dismutation of superoxide radicals. Most trace elements can form a divalent ion in biological systems that, in turn, competitively bind to the same sites (Bal et al. Citation2013; Cyert and Philpott Citation2013). As a result, the absorption and excretion of one trace element can affect that of another (Brzozowska Citation1989; Mondal et al. Citation2010). In our previous study, levels of the redox response-related elements were altered in tissues from mice injected with a rod-type titanium oxide nanoparticle (Park et al. Citation2014). In the current study, levels of Cu, Mn, Zn, Fe and Co were also altered to various degrees among the several tissues that were evaluated. These are essential trace elements needed to support proper growth, development and physiology. While there was no consistent trend across the changes in organ burdens of these metals, the one clear trend – especially with the higher dose of the long-type AlO NP – was for greater excretion of these metals in the feces. This is not to say that the short-type NP had no impact; with regard to Cu, Mn and Zn, both doses also seemed to cause increases (albeit non-dose-dependent and not significant in all cases) in fecal excretion of these elements. Further study is necessary to identify how homeostasis of trace elements might be impacted by exposure to/accumulation of these nanoparticles and how this too might be contributing to many of the effects of the AlO NP on immune-related endpoints observed here.
Almost all the metabolic processes in the body, including detoxification, protein synthesis and production of biochemical compounds necessary for digestion, are carried out in the liver (Zimmermann et al. 2012; Ceni et al. Citation2014). Some research has reported that blood levels of IL-8 were increased in animal models of liver damage; a similar outcome has been noted following the accumulation of nanoparticles in the liver (Feng et al. Citation2009; Gaiser et al. Citation2013; Amanzada et al. Citation2014). In addition, phagocytic cells, e.g. macrophages, neutrophils and dendritic cells (DC), can engulf and digest a foreign body and then can recruit other immune cells to the site by secreting various chemokines. IL-8 – a chemokine produced by macrophages, epithelial cells, airway smooth muscle cells and endothelial cells – has a primary function of facilitating the chemotaxis of neutrophils to inflammatory sites. MCP-1 – a chemokine secreted primarily by monocytes, macrophages and DC – also recruits monocytes, T-cells and DC. Moreover, pro-inflammatory cytokines, such as TNFα, IL-6 and IL-1β, partake in the inflammatory responses in liver injury models (Enomoto et al. Citation2007; Petrasek et al. Citation2012; Zimmermann et al. 2012) and IgE is a representative parameter antibody for immunotoxicity following exposure to nanoparticles (Di Gioacchino et al. Citation2011; Ban et al. Citation2013). In the current study, both AlO NP caused increases in levels of IL-8 and MCP-1 in the blood. Further, the levels of neutrophils and monocytes in WBC were increased by 5 mg/kg long-type AlO NP, an outcome accompanied by an increase in levels of IL-1β. The presence of IL-1β in the blood was enhanced only with the long-type AlO NP (at 5 mg/kg). Levels of TNFα, IL-6 and IgE were not affected by exposures to either form of AlO NP.
In our previous study, long-type AlO NP provoked more toxicity relative to short-type AlO NP (Park et al. Citation2014). However, the mechanisms of systemic immune responses induced by these nanoparticles are still not fully understood. In the present study, the spleen was seen to be a main target organ for accumulation of AlO NP. Growing evidence also suggest that nanoparticles can influence functions of antigen-presenting cells (APC), including B-cells, DC and macrophages (Tkach et al. Citation2013; Shurin et al. Citation2014). Specialized APC play a key role in adaptive immune responses that are initiated in the lymph nodes and spleen. Namely, APC process foreign substances and then present them to other immune cells in the context of co-stimulatory surface proteins, such as CD80, CD86, CD40 and MHC Class II (Palomaki et al. Citation2010). In addition, CD80 and CD86 are co-stimulatory molecules necessary for effective activation and sustainable proliferation of resting helper T-cells (Vasilevko et al. Citation2002). Moreover, CD195 (a C-C chemokine receptor known as CCR5) is predominantly expressed on T-cells, macrophages and DC and is a site at which MIP-1 specifically binds. The results here showed that the expression of CD195, CD80 and CD86 on splenocytes was attenuated by exposure to the AlO NP, especially the long-type form. A recent study reported that graphene oxide internalized into DC impaired the stimulatory potential of these DC by decreasing their intracellular levels of LMP7, immuno-proteasome subunits required for the processing of protein antigens (Tkach et al. Citation2013).
At this moment, we are not sure why there were reductions in expression of markers needed for antigen-presentation due to some of the exposures. In this study, it was shown that CD86 and CD195 expression was reduced (at higher doses) with both the short- and long-type rods. This laboratory is currently trying to elucidate the mechanisms associated with effects of NP on antigen-presentation/processing using various doses of NP; it is plausible the effects on markers of expression like CD80/CD86 or on CD195 seen here could be important factors in the already reported NP-induced alterations in those processes (Tkach et al. Citation2013; Shurin et al. Citation2014). Taken in context, because humans are continuously exposed to xenobiotics and both innate and acquired immunity are initiated by antigen uptake/processing by resident/recruited immune cells, accumulation of AlO NP in the spleen could ultimately impact on the defensive abilities of a host’s immune system as a result, in part, of this accumulated AlO NP causing defects in antigen-presentation/chemotaxis of immune system cells.
Conclusion
Taken together, the results of the studies here indicated that a high aspect ratio in AlO NP leads to enhanced accumulation of the nanoparticles in tissues following a single IV injection and, subsequently, that this accumulation may influence immune function. Further study is necessary to identify how these nanoparticles alter immune regulations; in particular, antigen presentation function in the spleen.
Supplement_3.doc
Download MS Word (121.5 KB)Supplemental_Figures_for_AlO_NP_paper.pptx
Download MS Power Point (1.1 MB)Acknowledgments
This work was supported by the Basic Science Research Program through the National Research Foundation of Korea funded by the Ministry of Education, Science and Technology (2011-35B-E00011).
Disclosure statement
The authors report no conflicts of interest. The authors alone are responsible for the content and writing of the paper.
References
- Amanzada A, Moriconi F, Mansuroglu T, Cameron S, Ramadori G, Malik IA. 2014. Induction of chemokines and cytokines before neutrophils and macrophage recruitment in different regions of rat liver after TAA administration. Lab Invest. 94:235–247.
- Bal W, Sokolowska M, Kurowska E, Faller P. 2013. Binding of transition metal ions to albumin: Sites, affinities and rates. Biochim Biophys Acta. 1830:5444–5455.
- Ban M, Langonné I, Huguet N, Guichard Y, Goutet M. 2013. Iron oxide particles modulate the ovalbumin-induced TH2 immune response in mice. Toxicol Lett. 216:31–39.
- Baud L, Ardaillou R. 1993. Involvement of reactive oxygen species in kidney damage. Br Med Bull. 49:621–629.
- Borgognoni CF, Mormann M, Qu Y, Schäfer M, Langer K, Öztürk C, Wagner S, Chen C, Zhao Y, Fuchs H, et al. 2015. Reaction of human macrophages on protein corona covered TiO2 nanoparticles. Nanomedicine. 11:275–282.
- Borm PJ, Robbins D, Haubold S, Kuhlbusch T, Fissan H, Donaldson K, Schins R, Stone V, Kreyling W, Lademann J, et al. 2006. The potential risks of nanomaterials: A review carried out for ECETOC. Particle Fibre Toxicol. 3:11.
- Braakhuis HM, Park MV, Gosens I, De Jong WH, Cassee FR. 2014. Physicochemical characteristics of nanomaterials that affect pulmonary inflammation. Particle Fibre Toxicol. 11:18.
- Brzozowska A. 1989. Interaction of iron, zinc and copper in the body of animals and humans. Rocz Panstw Zakl Hig. 40:302–312.
- Bruinink A, Wang J, Wick P. 2015. Effect of particle agglomeration in nanotoxicology. Arch Toxicol. 89:659–675.
- Ceni E, Mello T, Galli A. 2014. Pathogenesis of alcoholic liver disease: Role of oxidative metabolism. World J Gastroenterol. 20:17756–17772.
- Cheng LC, Jiang X, Wang J, Chen C, Liu RS. 2013. Nano-bio effects: Interaction of nanomaterials with cells. Nanoscale. 5:3547–3569.
- Cho M, Cho WS, Choi M, Kim SJ, Han BS, Kim SH, Kim HO, Sheen YY, Jeong J. 2009. The impact of size on tissue distribution and elimination by single intravenous injection of silica nanoparticles. Toxicol Lett. 189:177–183.
- Cyert MS, Philpott CC. 2013. Regulation of cation balance in Saccharomyces cerevisiae. Genetics. 193:677–713.
- Di Gioacchino M, Petrarca C, Lazzarin F, Di Giampaolo L, Sabbioni E, Boscolo P, Mariani-Costantini R, Bernardini G. 2011. Immunotoxicity of nanoparticles. Intl J Immunopathol Pharmacol. 24:65S–71S.
- Dykman L, Khlebtsov N. 2012. Gold nanoparticles in biomedical applications: Recent advances and perspectives. Chem Soc Rev. 41:2256–2282.
- Enomoto N, Takei Y, Yamashina S, Ikejima K, Kitamura T, Sato N. 2007. Anti-inflammatory strategies in alcoholic steato-hepatitis. J. Gastroenterol Hepatol. 22 (Suppl 1):S59–61.
- Feng D, Wang Y, Xu Y, Luo Q, Lan B, Xu L. 2009. IL-10 deficiency exacerbates halothane induced liver injury by increasing IL-8 expression and neutrophil infiltration. Biochem Pharmacol. 77:277–284.
- Gaiser BK, Hirn S, Kermanizadeh A, Kanase N, Fytianos K, Wenk A, Haberl N, Brunelli A, Kreyling WG, Stone V. 2013. Effects of silver nanoparticles on the liver and hepatocytes in vitro. Toxicol Sci. 131:537–547.
- Geraets L, Oomen AG, Krystek P, Jacobsen NR, Wallin H, Laurentie M, Verharen HW, Brandon EF, de Jong WH. 2014. Tissue distribution and elimination after oral and intravenous administration of different titanium dioxide nanoparticles in rats. Particle Fibre Toxicol. 11:30.
- Ghanta SR, Muralidharan T. 2013. Chemical synthesis of aluminum nanoparticles. J Nanopart Res. 15:1715–1724.
- Gomes M, Teixeira AL, Coelho A, Araújo A, Medeiros R. 2014. Role of inflammation in lung cancer. Adv Exp Med Biol. 816:1–23.
- Hagens WI, Oomen AG, de Jong WH, Cassee FR, Sips AJ. 2007. What do we (need to) know about the kinetic properties of nanoparticles in the body? Regul Toxicol Pharmacol. 49:217–229.
- Johnston H, Brown D, Kermanizadeh A, Gubbins E, Stone V. 2012. Investigating the relationship between nanomaterial hazard and physicochemical properties: Informing the exploitation of nanomaterials within the therapeutic and diagnostic applications. J Contr Rel. 164:307–313.
- Junnila SK. 2015. Type 1 diabetes epidemic in Finland is triggered by zinc-containing amorphous silica nanoparticles. Med Hypotheses. 84:336–340.
- Li N, Xia T, Nel AE. 2008. The role of oxidative stress in ambient particulate matter-induced lung diseases and its implications in the toxicity of engineered nanoparticles. Free Radic Biol Med. 44:1689–1699.
- Li XB, Zheng H, Zhang ZR, Li M, Huang ZY, Schluesener HJ, Li YY, Xu SQet al. 2009. Glia activation induced by peripheral administration of aluminum oxide nanoparticles in rat brains. Nanomedicine. 5:473–479.
- Li Y, Yu S, Wu Q, Tang M, Pu Y, Wang D. 2012. Chronic Al2O3-nanoparticle exposure causes neurotoxic effects on locomotion behaviors by inducing severe ROS production and disruption of ROS defense mechanisms in nematode. Caenorhabditis elegans. J Hazard Mater. 219:221–230.
- Mangge H, Becker K, Fuchs D, Gostner JM. 2014. Anti-oxidants, inflammation, and cardiovascular disease. World J Cardiol. 6:462–477.
- Moghimi SM, Hunter AC, Murray JC. 2005. Nanomedicine: Current status and future prospects. FASEB J. 19:311–330.
- Mondal S, Haldar S, Saha P, Ghosh TK. 2010. Metabolism and tissue distribution of trace elements in broiler chickens’ fed diets containing deficient and plethoric levels of copper, manganese, and zinc. Biol Trace Elem Res. 137:190–205.
- Morsy GM, Abou El-Ala KS, Ali AA. 2013a. Studies on fate and toxicity of nano-alumina in male albino rats: Oxidative stress in the brain, liver and kidney. Toxicol Ind Health. [Epub ahead of print]. DOI: 10.1177/0748233713498462.
- Morsy GM, Abou El-Ala KS, Ali AA. 2013b. Studies on fate and toxicity of nano-alumina in male albino rats: Lethality, bioaccumulation and genotoxicity. Toxicol Ind Health. [Epub ahead of print]. DOI: 10.1177/0748233713498449.
- Mukherjee D, Rai A, Zachariah MR. 2006. Quantitative laser-induced breakdown spec-troscopy for aerosols via internal calibration: Application to the oxidative coatings of aluminum nanoparticles. Aerosol Sci. 37:677–695.
- Nel AE, Mädler L, Velegol D, Xia T, Hoek EM, Somasundaran P, Klaessig F, Castranova V, Thompson M. 2009. Understanding biophysico-chemical interactions at the nano-bio interface. Nat Mater. 8:543–557.
- Oberdörster G, Oberdörster E, Oberdörster J. 2005. Nanotoxicology: An emerging discipline evolving from studies of ultrafine particles. Environ Health Perspect. 113:823–839.
- Oesterling E, Chopra N, Gavalas V, Arzuaga X, Lim EJ, Sultana R, Butterfield DA, Bachas L, Hennig B. 2008. Alumina nanoparticles induce expression of endothelial cell adhesion molecules. Toxicol Lett. 178:160–166.
- Palomäki J, Karisola P, Pylkkänen L, Savolainen K, Alenius H. 2010. Engineered nano-materials cause cytotoxicity and activation on mouse antigen-presenting cells. Toxicology. 267:125–131.
- Park EJ, Lee GH, Shim HW, Kim JH, Cho MH, Kim DW. 2014. Comparison of toxicity of different nanorod-type TiO2 polymorphs in vivo and in vitro. J Appl Toxicol. 34:357–366.
- Park EJ, Lee GH, Shim JH, Cho MH, Lee BS, Kim YB, Kim JH, Kim Y, Kim DW. 2015. Comparison of the toxicity of aluminum oxide nanorods with different aspect ratio. Arch. Toxicol. 88:1771–1782.
- Park EJ, Shim JH, Kim Y, Han BS, Yoon C, Lee S, Cho MH, Lee BS, Kim JH. 2015. A 13-week repeated dose oral toxicity and bioaccumulation of aluminum oxide nanoparticles in mice. Arch Toxicol. 89:371–379.
- Petrarca C, Perrone A, Verna N, Verginelli F, Ponti J, Sabbioni E, Di Giampaolo L, Dadorante V, Schiavone C, Boscolo P, et al. 2006. Cobalt nano-particles modulate cytokine in vitro release by human mononuclear cells mimicking autoimmune disease. Intl J Immunopathol Pharmacol. 19(Suppl 4):11–14.
- Petrasek J, Bala S, Csak T, Lippai D, Kodys K, Menashy V, Barrieau M, Min SY, Kurt-Jones EA, Szabo G. 2012. IL-1 receptor antagonist ameliorates inflammasome-dependent alcoholic steato-hepatitis in mice. J Clin Invest. 122:3476–3489.
- Prabhakar PV, Reddy UA, Singh SP, Balasubramanyam A, Rahman MF, Indu Kumari S, Agawane SB, Murty US, Grover P, Mahboob M. 2012. Oxidative stress induced by aluminum oxide nanomaterials after acute oral treatment in Wistar rats. J Appl Toxicol. 32:436–445.
- Ravishankar RV, Jamuna BA. 2011. Nanoparticles and their potential application as anti-microbials. In: Méndez-Vilas A, editor. Science against microbial pathogens: Communicating current research and technological advances. Badajoz (Spain): Formatex. p. 197–209.
- Roy R, Kumar S, Tripathi A, Das M, Dwivedi, PD. 2014. Interactive threats of nanoparticles to the biological system. Immunol Lett. 158:79–87.
- Saeidnia S, Abdollahi M. 2013. Toxicological and pharmacological concerns on oxidative stress and related diseases. Toxicol Appl Pharmacol. 273:442–455.
- Shrivastava R, Raza S, Yadav A, Kushwaha P, Flora SJ. 2014. Effects of sub-acute exposure to TiO2, ZnO and Al2O3 nanoparticles on oxidative stress and histological changes in mouse liver and brain. Drug Chem Toxicol. 37:336–347.
- Shurin MR, Yanamala N, Kisin ER, Tkach AV, Shurin GV, Murry AR, Leonard HD, Reynolds JS, Gutkin DW, Star A, et al. 2014. Graphene oxide attenuates TH2-type immune responses, but augments airway remodeling and hyper-responsiveness in a murine model of asthma. ACS Nano. 8:5585–5599.
- Staskal DF, Diliberto JJ, De Vito MJ, Birnbaum LS. 2005. Toxicokinetics of BDE 47 in female mice: Effect of dose, route of exposure, and time. Toxicol Sci. 83:215–223.
- Tavakoli AH, Maram PS, Widgeon SJ, Rufner J, van Benthem K, Ushakov S, Sen S, Navrotsky A. 2013. Amorphous alumina nanoparticles: Structure, surface energy, and thermodynamic phase stability. J Phys Chem. 117:17123–17130.
- Tkach AV, Yanamala N, Stanley S, Shurin MR, Shurin GV, Kisin ER, Murray AR, Pareso S, Khaliullin T, Kotchey GP, et al. 2013. Graphene oxide, but not fullerenes, targets immunoproteasomes and suppresses antigen presentation by dendritic cells. Small. 9:1686–1690.
- Vasilevko V, Ghochikyan A, Holterman MJ, Agadjanyan MG. 2002. CD80 (B7-1) and CD86 (B7-2) are functionally equivalent in the initiation and maintenance of CD4+ T-cell proliferation after activation with suboptimal doses of PHA. DNA Cell Biol. 21:137–149.
- Vereda F, Martin-Molina A, Hidalgo-Alvarez R, Quesada-Pérez, M. 2015. Specific ion effects on the electro-kinetic properties of iron oxide nanoparticles: Experiments and simulations. Phys Chem Chem Phys. 17:17069–17078.
- Willhite CC, Karyakina NA, Yokel RA, Yenugadhati N, Wisniewski TM, Arnold IM, Momoli F, Krewski D. 2014. Systematic review of potential health risks posed by pharmaceutical, occupational and consumer exposures to metallic and nano-scale aluminum, aluminum oxides, aluminum hydroxide and its soluble salts. Crit Rev Toxicol. 44(Suppl 4):1–80.
- Zimmemermann HW, Trautwein C, Tacke F. 2012. Functional role of monocytes and macrophages for the inflammatory response in acute liver injury. Front Physiol. 3:56.