Abstract
Multiple sclerosis (MS) is an autoimmune neurodegenerative disease characterized with immunopathobiological events, including lymphocytic infiltration into the central nervous system (CNS), microglia activation, demyelination and axonal degeneration. Although several neuroprotective drugs have been designed for the treatment of MS, complete remission is yet matter of debate. Therefore, development of novel therapeutic approaches for MS is of a high priority in immunological research. Nanomedicine is a recently developed novel medical field, which is applicable in both diagnosis and treatment of several cancers and autoimmune diseases. Although there is a marked progress in neuroimaging through using nanoparticles, little is known regarding the therapeutic potential of nanomedicine in neurological disorders, particularly MS. Moreover, the majority of data is limited to the MS related animal models. In this review, we will discuss about the brain targeting potential of different nanoparticles as well as the role of nanomedicine in the diagnosis and treatment of MS and its animal model, experimental autoimmune encephalomyelitis.
Introduction
Multiple sclerosis (MS) is the most common neurodegenerative disease of the central nervous system (CNS) in young adults; it affects about 1 million people worldwide, with a higher prevalence in Europe and North America (Ghalamfarsa et al. Citation2015; Ransohoff et al. Citation2015). MS is a CNS-related disorder characterized by chronic inflammation, local demyelination, gliosis, variable axonal destruction and substantial immune cell infiltration (Mirshafiey & Jadidi-Niaragh Citation2010b). Experimental autoimmune encephalomyelitis (EAE) is a widely used animal model of MS that has been induced via immunization with spinal cord homogenates, myelin or specific myelin peptides (Gharibi et al. Citation2015). Despite several attempts to develop treatments for MS, effective drugs with fewer side effects are still needed (Kim et al. Citation2015; Kokhaei et al. Citation2015).
Nanomedicine is a novel promising technology for treatment of various autoimmune disorders (Bahrami et al. Citation2015; Jadidi-Niaragh et al. Citation2016). It has been shown that nanoparticles (NP) can be used in both the diagnosis and treatment of immunopathologic diseases (Hosseini et al. Citation2015). However, treatment of neurological disorders has usually been associated with several problems, including the blood–brain barrier (BBB) hindrance and a variety of side effects (Jadidi-Niaragh & Mirshafiey Citation2012). In recent years, nanomedicine has improved the efficacy of diagnosis and CNS-targeted therapy of several neurologic disorders. Nano-engineering has provided important diagnostic/therapeutic advantages (Lu et al. Citation2014). As only small lipophilic molecules can cross the BBB, the use of many therapeutic drugs has been associated with several problems. Nanomedicine may help to overcome this BBB impediment in treating neurologic disorders. The capability of NP to traverse the BBB improved the chances for early diagnosis and effective MS therapy (Wong et al. Citation2012). This review discusses the role of nanomedicine in brain targeting, diagnosis and treatment of MS and its animal model, EAE.
Multiple sclerosis
Multiple sclerosis (MS) is a complex heterogeneous neurologic disorder and various clinical symptoms, such as changes in sensation, visual problems, muscle weakness and difficulty in coordination and speech have been demonstrated in these patients (Jadidi-Niaragh & Mirshafiey Citation2010). MS is characterized by demyelination and axonal loss mediated mainly through T-helper (TH) cells (particularly TH1 and TH17), macrophages and pro-inflammatory mediators (Jadidi-Niaragh & Mirshafiey Citation2011b). Although the etiology of MS is elusive, it seems that MS has an immunopathological trigger arising from gene–environment interactions. It is suggested that diet, sunlight, infections and genetics are of important etiologic factors in MS patients (Jadidi-Niaragh & Mirshafiey Citation2011a).
Multiple sclerosis (MS) is classified into four types. The majority of patients (∼85%) show an initial relapsing remitting (RRMS) disease that may shift toward secondary progressive MS (SPMS). About 10% of MS patients exhibit primary progressive MS (PPMS) characterized by continuous disease progression. Relapsing progressive MS (RPMS), a rare form of MS, is characterized by disease progression from onset, which will be followed by continuous relapses (Mirshafiey & Jadidi-Niaragh Citation2010a; Mirshafiey et al. Citation2014). In spite of promising advances in the understanding of MS pathogenesis, precise details about the neuroinflammatory processes remain elusive. In general, MS is characterized by generation of acute inflammatory lesions initiated by breakdown of the BBB. MS lesions can be categorized into active/inactive and acute/chronic lesions (Popescu & Lucchinetti Citation2012). Despite the presence of a large numbers of macrophages in acute and chronic active lesions, the axons are usually intact. On the other hand, while a few macrophages are present in inactive plaques, loss of axons and oligodendrocytes has been observed in these lesions. Smoldering plaques have been observed in intermediate stage of chronic active and inactive lesions, in which the center of plaques is hypo-cellular, while macrophages are seen frequently at the borders. Finally, shadow plaques are seen in re-myelinated lesions. In general, the acute and chronic active plaques are usually seen in RRMS and SPMS with relapses, while the inactive lesions occur in SPMS and PPMS (Weissert Citation2013). The molecular mimicry and bystander activation following interaction of immune cells with foreign antigens are important factors in induction of MS (Javaheri-Kermani et al. Citation2014; Libbey et al. Citation2014).
It has been shown that autoreactive T-cells that react with self-antigens of the CNS initiate and promote autoimmune responses against the CNS. T-cells (particularly TH cells) orchestrate cellular and humoral responses leading to neuroinflammatory processes that damage myelin, oligodendrocytes and neurons. Dendritic cells (DC) that present self-antigens to auto-reactive T-cells play a crucial role in the induction of autoreactive responses (Xie et al. Citation2015). It seems TH1 and TH17 cells are the main pathogenic populations in the immunopathogenesis of MS (Jadidi-Niaragh & Mirshafiey Citation2011b). Although there are no DC in a healthy CNS, other antigen-presenting cells (APC) like macrophages, microglia, B cells, endothelial cells and astrocytes can prime autoreactive T-cells at the disease initiation stage (Ransohoff & Engelhardt Citation2012; Miljković & Spasojević Citation2013; Yazdani et al. Citation2013). Interestingly, it was reported that microglia and macrophages could differentiate into DC-like cells (Ponomarev et al. Citation2005).
It has been suggested that obstructed blood flow in narrow vessels lead to shear stress, which results in increased production of reactive oxygen and nitrogen species (oxidative stress) in endothelial cells. These reactive species enter astrocytes and increase oxidative stress. Accumulation of oxidative stress leads to activation of macrophages/microglia and induces apoptosis in oligodendrocytes. These sequential events may describe the initial stages of MS occurrence, suggesting a mechanism by which crosstalk between the circulation and the brain can lead to disruption of the BBB. It has been shown that oxidative stress significantly increases the production and function of matrix metallopeptidase (MMP)-2 and -9 in endothelial cells and macrophages (Yazdani et al. Citation2011; Ortiz et al. Citation2013; Mirshafiey et al. Citation2014). The increased levels of these enzymes have been reported in astrocytes in MS lesions (Cuzner et al. Citation1996). The cleavage of extracellular matrix by these enzymes not only damages the CNS parenchyma and BBB integrity, but also creates several protein fragments, which may act as chemoattractants and immunomodulators (Weathington et al. Citation2006). Altogether, these events disturb BBB integrity and recruit various leukocytes into the CNS (Miljković & Spasojević Citation2013) ().
Figure 1. Schematic representation of the immunological and pathological events observed in MS. Oxidative stress in endothelial cells leads to damage to astrocytes, activation of macro-phages/microglial cells and apoptosis in oligodendrocytes. Oxidative stress also increases the production and function of MMPS in endothelial cells and macrophages. These sequential events enhance disruption of BBB. Destruction of the CNS parenchyma and BBB lead to generation of creates chemotactic factors for leukocytes. Therefore, these events disturb BBB integrity and attract leukocytes into the CNS. The myelin-derived antigens will be taken by APC and presented to CD4+ and CD8+ T-cells. Inflammatory mediators such as osteopontin, IL-1, IL-12, TNFα, IL-23 and other cytokines enhance the differentiation of CD4+ TH cells toward TH1 and TH17 cells. These inflammatory leukocytes in the CNS can either directly or indirectly induce neuronal, axonal and oligodendrocyte damage. MS: multiple sclerosis, CNS: central nervous system, TGF: transforming growth factor, IL: interleukin, MQ: macrophage, IFN: interferon, H1R: histamine receptor 1, H2R: histamine receptor 2, JAM: junctional adhesion molecules, MMPs: matrix metalloproteinases, PBM: parenchymal basement membrane, ROS: reactive oxygen species, VBM: vascular basement membrane.
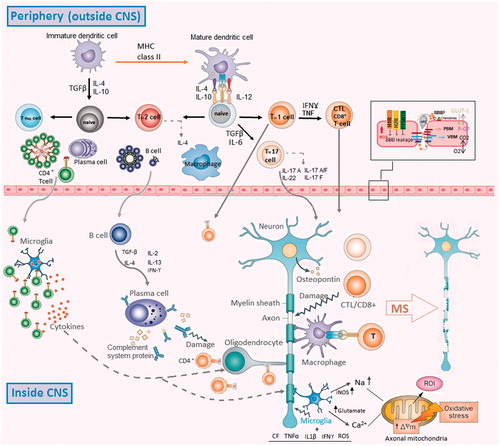
It has been shown that there are a number of different myelin epitopes that act as self-antigens in MS (Bahbouhi et al. Citation2010) and oxidative stress may play an important role in the formation of these epitopes. Consistently, combination of malondialdehyde (MDA) with myelin oligodendrocyte glycoprotein (MOG) improves the phagocytosis of MOG by APC. Moreover, administration of MDA-MOG to mice leads to increased numbers of TH17 and TH1 cells, which are the most important cells in immunopathogenesis of MS (Wållberg et al. Citation2007). Notably, increased levels of MDA and MDA adducts have been detected in cerebrospinal fluid (CSF) of MS patients (Ghabaee et al. Citation2010). These data may indicate that myelin-derived self-antigens are not sufficient to induce autoreactive responses and the presence of myelin-like immunogenic molecules such as MDA-MOG, 4-hydroxy-2-nonenal (HNE)- and neuroketal-adducts, nitrosylated peptides or citrullinated-myelin basic protein (MBP) is crucial to induce autoimmune responses (Gonzalo et al. Citation2012).
As described before, the oxidative stress damages myelin and releases myelin fragments, which are phagocytosed by microglia and macrophages. These cells present self-antigens to CD4+ and CD8+ T-cells and facilitate their differentiation into activated autoreactive cells. The micro-environmental factors such as osteopontin, interleukin (IL)-1, IL-12, IL-23, tumor necrosis factor (TNF)-α and other cytokines secreted by various cells such as astrocytes enhance the differentiation of CD4+ TH cells toward TH1 and TH17 cells (Jadidi-Niaragh & Mirshafiey Citation2011b). Finally, the pro-inflammatory and cytotoxic mediators released by both TH and CD8+ cytotoxic T lymphocytes enhance several self-reactive responses, which damage tissue. On the other hand, DC can collect myelin-derived fragments in the CNS and migrate into the lymph nodes. Following antigen presentation to autoreactive T-cells by these DC, TH and CD8+ T-cells migrate into the CNS and damage tissue where this antigen is present (Miljković & Spasojević Citation2013). Oxidative stress enhances the secretion of different cytokines and chemokines by astrocytes, which recruit leukocytes into the CNS. Specifically, H2O2 increases the expression of VCAM-1 and CCL2 on endothelial cells. Moreover, TH17-derived IL-17 enhances the generation of CCL20 and inhibits the expression of CXCL12 in the endothelial cells, which lead to increased leukocyte infiltration into the CNS (Høglund & Maghazachi Citation2014).
During the last two decades, several studies have performed to introduce new therapeutic approaches for MS patients. Among the current MS drugs, interferon (IFN)-β was the first marketed (Jacobs et al. Citation1996). IFNβ exerts its therapeutic function in part through anti-viral, anti-proliferative, and, particularly, immunomodulatory actions (Kasper & Reder Citation2014). Subsequently, glatiramer acetate was introduced as a MS drug, which affects antigen presentation and cytokine balance (Caporro et al. Citation2014). The humanized anti-VLA-4 monoclonal antibody, natalizumab, inhibits T-cell infiltration into the CNS (Hoepner et al. Citation2014). Fingolimod was the first MS oral drug, which inhibited T-cell migration (Kim et al. Citation2015). Finally, teriflunomide and dimethly fumarate are the newest marketed MS drugs with evidence of efficacy (Jadidi-Niaragh & Mirshafiey Citation2011c). Almost all these drugs are mainly effective for relapsing forms of MS; however, the use of these drugs in MS patients is associated with variety of side effects. Currently, attempts to design new therapeutic agents in MS patients remain ongoing.
Nanoparticles and drug delivery into the CNS
The CNS physiologic barriers, including the BBB and the blood–cerebrospinal fluid (CSF) barrier (BCSFB) limit access to the CNS for the majority of systemically delivered drugs, and hence hamper treatment. Although these barriers inhibit the systemic drug delivery into the CNS, they protect the CNS against infection and toxins. While the BBB is formed by tight junctions of capillary endothelial cells and the astrocytes, BCSFB consists of the tight junctions of choroid plexus cells encompassing the microvascular endothelium (Ortiz et al. Citation2014). These barriers precisely control the transfer of molecules between the blood and CNS or CSF. The transfer of biologic molecules from blood into the CNS or CSF is through a trans-cellular pathway via the capillary endothelial cells or choroid plexus cells in passive and/or active dependent manner. Lipophilic molecules with the molecular weight <600 Daltons can usually cross the BBB and BCSFB. It has been shown that the essential nutrients such as amino acids, carbohydrates and nucleosides may cross BBB and BSCSFB through cellular transport systems such as receptors and carriers. Among these transport mechanisms, the natural transfer of macromolecules conjugated with surface receptor ligands like insulin, lactoferrin or transferrin is in a receptor-mediated manner (). Moreover, cationic molecules and peptides such as albumin can pass across neurologic barriers through receptor-mediated absorptive endocytosis. There are also active transport systems such as system L-transporters, concentrative nucleoside transporter, P-glycoprotein, multidrug resistance-associated proteins, etc., that can transport different molecules into the CNS in adenosine triphosphate (ATP)-dependent manner (Donaghue et al. Citation2014; Lu et al. Citation2014).
Figure 2. (A) The size of the nanoparticles is an important criteria in transportation of loaded drugs. (B) Poly-dispersity of particle size may create variability in the release rates. (C) Surface properties of NP can significantly affect their drug delivery into the CNS. Modification of the surface with polymers such as polyethylene glycol (PEG) can increase the circulation time of conjugated particles. Moreover, surface modification of nanoparticles can affect the interaction of these particles with immune cells.
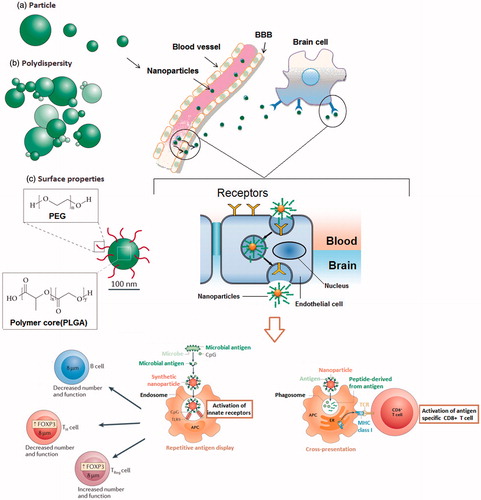
The use of nanoparticulate carriers for drug delivery into the CNS has been studied and some of them such as poly(lactic-co-glycolic acid) (PLGA) are clinically approved that will be discussed. Nanobiotechnology applies nanoscale materials (usually <100 nm in at least one dimension) in pharmaceutical and biomedical approaches, however, there are efforts to bring a more rigorous definition to the nanometer range. It should be noted this size is a recommendation by certain bodies and not a universally applied restriction when describing nanomedicine. Several nanomaterials discussed in this manuscript are >100 nm and materials <1 micron can usually be considered as nanoparticulate. Nevertheless, the CNS-penetrating ability of NP makes them promising tools for early diagnosis of the CNS disorders (Kanwar et al. Citation2012). Infiltration of NP into the CNS is related to their small size and surface features.
Micelles
Micelles exist as natural and synthetic polymers with size range from 2 to 20 nm, depending on composition and concentration. Polymeric micelles are composed of a hydrophobic polymer core [such as poly(propylene glycol)] and a shell of hydrophilic polymer blocks such as poly-ethylene glycol (PEG) (Lu et al. Citation2014). The ability to make nanoscale micelles through self-assembly in a specific solvent is the main feature of amphiphilic block copolymers. Block co-polymers comprise two or more homo-polymer subunits linked by covalent bonds. These nanoscale micelles have been used as carriers in therapeutic systems (Jang et al. Citation2006). Regarding the small size of these carriers, they can transport drugs across neurons to the brain via endocytosis (Kanazawa Citation2015). Polymeric micelles composed of Pluronic block co-polymers (also termed poloxamer) are effective carriers for hydrophobic drugs with long-circulating characteristics via inhibition of phagocytosis by the reticuloendothelial system (Batrakova & Kabanov Citation2008). Pluronic block co-polymers are approved by Food and Drug Administration (FDA) for pharmaceutical and clinical applications and listed in the US and British pharmacopoeia as pharmaceutical excipients (Chowdhary et al. Citation2003).
As pluronic block micelles suppress ABC drug efflux transporters in BBB, they enhance drug delivery into the brain. These efflux ABC transporters are major obstacles for drug delivery into the brain. While the pluronic block micelles suppress drug efflux transporters in the BBB, they exhibit no toxicity in the BBB (Batrakova et al. Citation1998). Pluronic block copolymers modulate drug efflux transporters in part through ATP depletion and membrane fluidization in the BBB (Kabanov et al. Citation2003). It has been demonstrated that the intranasal administration of coumarin loaded methoxyPEG(MPEG)polycaprolactone (MPEG-PCL) nanomicelles to rats was associated with accumulation of coumarin in brain tissues without toxicity (Kanazawa et al. Citation2011). It should be noted that coumarin is a fragrant organic chemical compound in the benzopyrone chemical class and exhibits promising brain permeability and myelin-binding properties (Wang et al. Citation2011a).
Following the deep, high delivery of nano-formulations into the nasal cavity, they reach to olfactory mucosa and are transported into the brain and CSF through the olfactory receptor neurons. There are two feasible routes for nano-formulations to reach into the brain, including the olfactory nerve pathway (axonal transport) and the olfactory epithelial pathway (Landau et al. Citation1994). To overcome the BBB-mediated exclusion of brain-targeted drugs carbopol-based gels are designed for nasal delivery of biopharmaceuticals (Illum Citation2004). Modified polymeric micelles were developed for effective drug delivery into the CNS. Zhang and colleagues were developed a transferrin-conjugated cyclo-(RGDFK)-paclitaxel micelle that could effectively transport drugs into brain in vivo (Zhang et al. Citation2012). The surface modification of micelles with cell-penetrating peptides (CPP) was another promising approach to specific CNS delivery of various micelles loaded with drugs. These CPP are cationic or amphiphilic peptides derived from Tat protein (from human immunodeficiency virus) and a Drosophila antennapedia homeoprotein that enhance intracellular delivery of drugs (Rajagopalan et al. Citation2007b).
A main domain of Tat has a sequence responsible for cellular uptake through the 11-amino acid epitope YGRKKRRQRRR (Rajagopalan et al. Citation2007a). It seems that conjugation of the CPP to the surface of nanocarriers can increase their penetration across the nasal mucosa and target cell membranes in the brain (Kanazawa Citation2015). Conjugation of MPEG-PCL micelles with Tat peptide (MPEG-PCL-Tat) enhanced the intranasal brain delivery of coumarin in rats (Kanazawa et al. Citation2011). Thus, it seems that micelles may be promising tools in the CNS-drug delivery in MS patients. Although, some micelle-based NP are marketed or analyzed in pre-clinical studies (Marcato & Durán Citation2008), there is no approved micelle-based nano-formulation for the treatment of MS.
Nano-emulsions
Nano-emulsions are characterized as a system of water, oil and amphiphile, stabilized with a surface-active film. Films surround the water droplets at the oil/water interface and increase the stability of an emulsion by increasing the interfacial viscosity. These films are created following the adsorption of high-molecular-weight polar molecules that are inter-facially active (surfactant-like behavior) (Lee et al. Citation2014). Oils that are rich in ω-3 and ω-6 poly-unsaturated fatty acids (PUFA) such as fish oils, pine-nut, flaxseed, hemp, safflower and wheat-germ oils can be used in the production of nano-emulsions. Amphiphilic surfactants such as egg phosphatidylcholine and co-surfactant molecules including stearylamine, deoxycholic acid and dioleoyltrimethyl-ammonium propane (DOTAP) can be also applied for stabilization of these nano-formulations (Ganta et al. Citation2010).
Regarding their high loading efficiency, nanoscale diameter and good penetration capacity, nano-emulsions can be attractive devices in drug delivery into the CNS (Lu et al. Citation2014). As a drug carrier, they have several advantages such as effective encapsulation, sustained drug release and long circulatory times. It is suggested that if targeting moieties such as folate (Patlolla & Vobalaboina Citation2008), thiamine (Oyewumi et al. Citation2003), endothelial growth factor receptor (EGFR) binding peptide (Ganta et al. Citation2015), mannose (Rutishauser & Gutknecht Citation1976), etc., to be anchored to the nano-emulsion, they can deliver their cargo with significantly higher efficiencies and remain longer at the disease site to allow for total transfer of the drug molecules. The size of nano-emulsions is <500 nm in diameter; these can successfully incorporate poorly soluble drugs (Ganta et al. Citation2008; Vyas et al. Citation2008). Nano-emulsions release drug molecules through fusion of oil droplets to the biological membrane (Wickline & Lanza Citation2003). There are several methods that can be applied for preparation of nano-emulsions, however, high-energy emulsification method is preferred (Krishnamachari et al. Citation2011). High-energy emulsification approaches use mechanical energy that creates intense disruptive forces leading to the formation of tiny oil droplets. However, high-energy emulsification may not be appropriate for thermo-labile drugs. Regarding the fact that many oils used in nano-emulsions are edible, it is usually administered orally (Kumar et al. Citation2008). Oral administration of saquinavir (HIV protease inhibitor)-loaded nano-emulsion to male Balb/c mice significantly increased the drug levels in the brain (Vyas et al. Citation2008). Orally administered nano-emulsions can be stabilized by deoxycholic acid that promotes a bypass of the first pass metabolism. Moreover, PUFA oil-containing nano-emulsions rapidly transport drug to peripheral sites, particularly the brain (Taogoshi et al. Citation2005). Thus, they were expected to be potent carriers for the delivery of drugs across the brain (Tiwari et al. Citation2006).
There are some nano-emulsions commercialized by industries for biological and medical applications (Marcato & Durán Citation2008). Moreover, there are some FDA-approved nano-emulsion based formulations such as Camptosar and Fluosol that have been analyzed in MS patients (Sarker Citation2005). However, nano-emulsions have not been used for drug delivery to MS patients and this issue can be investigated in future studies.
Liposomes
Liposomes are spherical unilamellar or multilamellar lipid vesicles composed of one or more phospholipid bilayers with aqueous center. Therefore, they can readily encapsulate hydrophilic, amphiphilic and lipophilic drugs in their center or within the phospholipid bilayer. Although there are several methods for production of liposomes, the thin-film hydration or Bangham technique is extensively used which includes dissolution of the lipid in a biologic solvent, solvent evaporation and the dispersion of the lipid film in aqueous media (Bangham et al. Citation1965). Different types of liposomes can be applied for various purposes (Krishnamachari et al. Citation2011). Conventional liposomes usually contain neutral lipids (such as cholesterol, dimyristoyl phosphatidylglycerol, dipalmitoyl phosphatidylcholine) and phosphatidylcholine. In addition to conventional liposomes, there are other types of liposomes such as stealth liposomes (sterically stabilized liposomes), cationic liposomes (amphiphilic molecules composed of a charged head connected to a hydrophobic anchor), archaeosomes (comprise the unique glycerolipids of Archaea), virosomes (unilamellar lipid envelops, derived from viruses), etc., which can be used for different goals. Furthermore, liposomes can also be functionally categorized into stimuli–responsive, pH-sensitive, thermo-sensitive, fusogenic and immunoliposomes that each one has its specific characteristics which let us to choice the best option for our different goals (Tila et al. Citation2015). The short circulating time (due to uptake by phagocytes and self destruction) is one of the most important limitations of liposomes and can be improved in part through decreasing their size (<100 nm) or conjugation with PEG molecules (PEGylation).
In general, nanocarrier systems with lower size have usually higher circulating time compared to large NP, however, this claim is not consistent for all nano-formulations. For example, it is demonstrated that poly-amidoamine (PAMAM) dendrimer magnetic resonance imaging (MRI)-contrast agents appear to have longer circulation times with larger diameter (generation) (Kobayashi et al. Citation2004). The size of the liposome-based NP plays an important role in the clinical outcome. It is demonstrated that liposomes smaller than 100 nm in diameter exhibit a longer half-life in the blood and can evaded from the reticuloendothelial system compared to large liposomes (Allen et al. Citation1993). Similar results were observed when liposomes with the size 250 nm in diameter were compared with liposomes 100 nm in diameter with similar lipid composition (Niven et al. Citation1991).
As recently reviewed in Bozzuto and Molinari (Citation2015), several liposome-based nano-formulations have been marketed or were under investigation in clinical trials for the treatment of various diseases, particularly cancer. However, none were designed for treatment of MS. Doxil (100 nm) was the first liposome-based NP that received FDA approval for the treatment of chemotherapy-refractory Kaposi’s sarcoma in AIDS patients and recurrent epithelial ovarian cancer (Barenholz Citation2012). It has been shown that surface modification of liposomes including transferrin-modified liposomes (Koshkaryev et al. Citation2012), glucose modified liposomes GLU1000-LIP (Xie et al. Citation2012), Tat-conjugated liposomes (Wang et al. Citation2013) and dual transferrin and folate-conjugated liposomes (Niu et al. Citation2011) could increase their brain targeting. Thus, it seems that use of modified liposomes may be effective therapeutic approach in MS therapy.
Nanospheres and nanocapsules
Nanospheres are NP composed of a solid core surrounded by a dense polymer matrix, which is usually prepared through microemulsion polymerization method and have sizes from 100 to1000 nm. Various drugs can be encapsulated, entrapped, dispersed, adsorbed or chemically attached to these NP (Letchford & Burt Citation2007). It is demonstrated that various materials such as collagen, gelatin, chitosan, fibrin, alginate, poly-(α-hydroxy-esters), such as poly-(lactic acid) (PLA), poly-(glycolic acid), PLGA and poly-(caprolactone) (PCL), are the most frequently used synthetic polymers for generation of nanospheres (Wang et al. Citation2011b). Among them, PLGA is a commonly used [and only FDA-approved] compound that exhibits low toxicity and high efficiency compared to the other compounds (Makadia & Siegel Citation2011). Little is known about the efficiency of nanospheres in treatment of neurological disorders. Wolfhart and colleagues demonstrated that surfactant-coated doxorubicin-loaded nanospheres could effectively cross the BBB and reach to the brain parenchyma in animal models (Wohlfart et al. Citation2011, Citation2012). Regarding the existence of limited information, nanospheres should be investigated in a variety of pathologic conditions.
Nanocapsules are characterized with oil-filled cavity surrounded by a thin polymeric envelope (core-shell structure) and have sizes ranging from about 10 to 1000 nm (Hwang & Kim Citation2014). Several compounds such as hydroxypropoxy-methylcellulose, hydroxypropyl-methyl-cellulose, gum arabica, phthalate, diacyl-β-cyclodextrin, poly-(ɛ-caprolactone), poly-(D,L-lactide), poly-(alkylcyanoacrylate), vegetable or mineral oils, ethyloleate and benzyl benzoate can be used for production of nanocapsules. Moreover, both hydrophilic (laryl sulphate, quatery ammonium, or [poly-(oxyethylene) – poly 72-(propylene) glycol]) and lipophilic (natural lecithin) surfactants stabilize the nanocapsules (Kothamasu et al. Citation2012). The interfacial polymerization and the interfacial nanodeposition are the most frequent methods used for the generation of monomer and polymer nanocapsules. It has been shown that the size of nanospheres can affect their stability. Thus, nanospheres composed of PLGA with higher sizes were degraded faster than smaller NP (Dunne et al. Citation2000). It was also reported that the surface modification of nanocapsules with targeting molecules such as transferrin (Ogris et al. Citation1999; Dash et al. Citation2000) and folate (Leamon & Low Citation2001; Gosselin & Lee Citation2002) could enhance the efficacy of these NP in therapeutic interventions. Moreover, conjugation of nanocapsules with CNS-targeting molecules such as OX26 monoclonal antibody (Béduneau et al. Citation2007), Fab fragments (Maruyama et al. Citation1997) or transferrin (Béduneau et al. Citation2008) was also associated with good outcomes. While there have been several attempts to analyze the efficacy of nanocapsules in the treatment of various diseases like cancer (Shen et al. Citation2010; Vanpouille-Box et al. Citation2011), diabetes (Graf et al. Citation2009; Vanpouille-Box et al. Citation2011) and infectious diseases (Paiphansiri et al. Citation2007), there is still no data regarding their therapeutic potential in neurological disorders, particularly MS.
Altogether, nanospheres and nanocapsules demonstrate several advantages such as high drug loading, high circulation time in plasma, ease of surface modification and high resistance against reticuloendothelial system (Gao et al. Citation2013). The nanosphere-based Verigene® system is approved for the identification of influenza A, influenza B and respiratory syncytial virus from nasopharyngeal swab specimens placed in viral transport media (Ginocchio Citation2011). Although the neuroprotective function of these NP has been shown in vitro, little is known regarding their efficacy in vivo. However, it seems that these NP with some surface modifications may be the promising tools in the CNS drug delivery in MS patients (Kanwar et al. Citation2012).
Polymeric NP
Polymeric NP (PNP) is comprised of natural (e.g. albumin, gelatin, chitosan, poly-saccharides) or synthetic (e.g. polyacrylamide, e-poly-caprolactone, polyacrylates) polymers. Based on the preparation process, PNP may appear as nanospheres or nanocapsule structures with sizes ranging from 100 to 1000 nm (Parveen & Sahoo Citation2008). Various PNP, including PLGA, chitosan, polybutylcyanoacrylate (PBCA), PCL, PLA, polyglycolide, poly-(D, L-lactide) and PLGA–PEG have been used for drug delivery for the treatment of different immunopathologic conditions (Danhier et al. Citation2009; Cheng et al. Citation2012; Prabhu et al. Citation2015). Among the PNP found, PLGA, PLA and PCL have been approved by the FDA for human use (Wilczewska et al. Citation2012). PLGA NP has high capacity to protect their cargo from degradation and prolong the release of therapeutic agents, so they have been used in several pre-clinical studies for treatment of various diseases, particularly cancer (Elamanchili et al. Citation2007). PLA NP are homo-polymers that are more crystalline compared to PLGA. Although it is evident that the more attention has been focused on PLGA compared to PLA, it is difficult to say PLGA is better that PLA.
PNP can be classified as biodegradable or non-biodegradable. Biodegradable NP exhibit several advantages, including high stability and less immunogenicity (Krishnamachari et al. Citation2011). These NP are biocompatible and do not stimulate any inflammatory response. The high capacities of these NP for entrapping both hydrophilic and hydrophobic drugs along with excellent release profiles have made them promising tools for drug delivery systems. Several studies have demonstrated the CNS drug delivery through various PNP such as PBCA (Tian et al. Citation2011), methylmethacrylate-sulfopropylmethacrylate-PBCA (Kuo & Chen Citation2006), PLGA/algi-nate (Kim & Martin Citation2006), PLA/PEG (Gao et al. Citation2007) and PLGA (Reddy & Labhasetwar Citation2009). Regarding the low BBB transmission efficiency, surface modification of these NP with molecules such as antibodies, surfactant or transferrin can make them potent carriers for drug delivery into the CNS (Lu et al. Citation2014). Anti-transferrin receptor antibody conjugated PNP could effectively deliver drugs such as methotrexate, proteins like basic fibroblast growth factor, nerve growth factor and brain-derived neurotrophic factor into the CNS (Pardridge et al. Citation1995, Citation1998). Although, there are some FDA approved PNP such as Carmustine for glioblastoma multiform, Risperdal Consta for schizophrenia and Abraxane for mammary cancers, there is no PNP-based therapeutic approved for the diagnosis or treatment of MS (Marcato & Durán Citation2008; Prabhu et al. Citation2015). Moreover, there are only a few pre-clinical studies that have tried to test the efficiency of PNP in drug delivery into the CNS (Foley et al. Citation2012; Nance et al. Citation2012; Zhou et al. Citation2013).
Solid lipid NP
SLN (or lipospheres) are composed of solid lipids, including triglycerides, waxes or complex glyceride mixtures stabilized by surfactants, with an average diameter between 10 and 1000 nm. Among the various techniques used for production of SLN, high-pressure homogenization at high temperatures (Silva et al. Citation2011) and microemulsion (Youssef et al. Citation2012) are the most applied methods used for SLN generation. These NP have several features such as high stability and tolerability, gradual drug release, specific targeting and protection of labile drugs from degradation. However, SLN show some limitations such as low drug-loading potential and drug leakage (de Jesus & Zuhorn Citation2015). Lipid drug conjugates (LDC) and nano-structured lipid carriers (NLC) are improved SLN that have additional advantages compared to SLN (Rostami et al. Citation2014). The main purpose in the development of LDC is to improve the compatibility of lipid based carriers to lipophobic molecules. NLC are lipid carriers containing a certain structure for the increased payload capacity and prevention of drug expulsion.
Accordingly, it was shown that NLC could be classified into three types based on their lipid matrix structure, e.g. imperfect type, multiple types and amorphous or structure-less type. The imperfect type has the lowest oil content and exhibits a defected lipid matrix and compartments for drug storage that leads to drug expulsion (Teeranachaideekul et al. Citation2007). Increases in liquid-phase lipids in the multiple types of NLC inhibit drug expulsion. This type of NLC exhibits a higher drug loading and faster drug release compared to other NLC (Yuan et al. Citation2007). Use of lipids such as hydroxy-octacosanyl-hydroxystearate and isopropyl-myristate leads to preparation of the amorphous type that lacks a crystalline structure and avoids drug expulsion (de Jesus & Zuhorn Citation2015).
SLN exhibits several features such as evasion from the reticuloendothelial (SLN in range of 120–200 nm) (Wohlfart et al. Citation2012), high stability [for even 3 years; Freitas & Müller Citation1998], high drug-loading efficiency, high reproducibility (Fundarò et al. Citation2000), incorporation of both hydrophilic and hydrophobic drugs (Chen et al. Citation2001) and simple production on large scale (Muller et al. Citation2000), that make them potent candidate for drug delivery into the CNS. As a result, SLN have been used to deliver several therapeutic agents such as doxorubicin (Agarwal et al. Citation2011), atazanavir (Chattopadhyay et al. Citation2008), quinine (Gupta et al. Citation2007), docetaxel (Venishetty et al. Citation2013) and quercetin (Dhawan et al. Citation2011) into the brain, as described in previous studies. Regarding the lipidic properties of SLN, it is expected that SLN easily enter into the brain tissues. Because SLN are biodegradable, they show very low toxicity. As such, they were originally thought to be promising candidates for drug delivery into the CNS (Kaur et al. Citation2008). Moreover, it has been reported that surface modification of SLN with targeting molecules such as galactosylation (Xu et al. Citation2009) or folate (Stevens et al. Citation2004) enhanced their specific action.
Thus, the addition of CNS-targeting molecules to SLN may increase specific drug delivery in neurological disorders. In line with this, it was reported that conjugation of SLN with transferrin (Gupta et al. Citation2007) or thiamine (Lockman et al. Citation2003) was associated with better outcomes in the treatment of cerebral malaria and increased brain uptake of drug, respectively. Although several SLN nano-formulations have been tested in pre-clinical studies (Reddy et al. Citation2005; Ye et al. Citation2008), and some of them – such as Nanobase and Nanopearl – were approved by the FDA (Marcato & Durán Citation2008), there are still no marketed SLN-based nano-formulations for neurologic disorders, particularly MS.
Dendrimers
Dendrimers are nanosized (1–100 nm) spherical compounds with repetitively branched structures. Dendrimers contain an initiator core, some interior layers consisted of repeating units and several active surface terminal groups. Dendrimers can be conveniently prepared by Fréchet-type convergent synthesis, in which in the first dendrons are generated and then attached to a core center (Hawker & Frechet Citation1990) or Tomalia-type divergent synthesis, in which the dendrimer is synthesized from the core to the periphery (Esfand & Tomalia Citation2001). There are several types of dendrimers, including PAMAM, poly(propyleneimine) (PPI), poly-l-lysine, melamine, triazine, poly(glycerol), poly[2,2-bis(hydroxymethyl)propionic acid] and poly-(etherhydroxylamine). However, PAMAM-type dendrimers have been studied more than other types as they have been commercially available for the longest period from different companies and have advantages compared to the other types (Menjoge et al. Citation2010; Xu et al. Citation2013).
The high versatility of dendrimers has introduced them as potent nanocarriers in the CNS drug delivery (Xu et al. Citation2013). However, their three key features including monodispersity, their multi-valency and their globular shape make them as potent nanotheranostic devices in biomedical fields (Arseneault et al. Citation2015). Dendrimers can be designed with several reactive groups, facilitating surface modification. Several targeting ligands such as biotin, folic acid, amino acids, peptides, aptamers and monoclonal antibodies have been successfully anchored onto dendrimer surfaces that were associated with selective and efficient targeting (Kesharwani et al. Citation2014a,Citationb). Moreover, conjugation of dendrimers with the CNS targeting molecules such as transferrin (Jackson & Linsley Citation2010; Beg et al. Citation2011), lactoferrin (Huang et al. Citation2008), angiopeps (Demeule et al. Citation2008; Ke et al. Citation2009a), D-glucosamine (Dhanikula et al. Citation2008) and leptin 30 (Liu et al. Citation2010) led to increased accumulation of therapeutic agents in the brain tissue. Both in vitro and in vivo studies have demonstrated the low toxicity and high CNS drug delivery of dendrimers (Hemmer et al. Citation2013; Bertero et al. Citation2014).
Due to their small size and highly packed surface functionalities, the functional groups can lead to synergistic effects of interacting with other biomolecules, i.e. poly-valency. This multi-valency led to the formation of various commercialized dendrimers (such as Priostar [Svenson et al. Citation2006], Starburst [Tomalia & Fréchet Citation2002], Astramol [Zhiryakova & Izumrudov Citation2014], Polylysine poly-L-lysine [Patton et al. Citation2006]) and dendrimer-based products such as Vivagel (Rupp et al. Citation2007), Stratus CS (Christenson et al. Citation2004), Superfect (Liu et al. Citation2012), Dendrimer-docetaxel, Dendrimer-oxaliplatin (Madaan et al. Citation2014), NB-001 and NB-002 (Marcato & Durán Citation2008).
Nanogels
Nanogels are nanosized polymeric microgels composed of a network of charged poly-ionic residues attached to PEG molecules, which can effectively encapsulate various types of oligonucleotides and charged molecules. Nanogels are composed of a crosslinked hydrophilic polymer network, known as hydrogel, with sizes the tens to hundreds nm in diameter. Chemically or physically crosslinked synthetic polymers or biopolymers, such as dextran and polyethylenimine (PEI), are usually constitute to generation of nanogels (Bencherif et al. Citation2009). Different nanogels can be used for different purposes. For example, PEI-derived nanogels can be used for anti-cancer drug delivery (Surtees et al. Citation1992), whereas dextran derived nanogels are applied for imaging tumor-associated macrophages with radionuclides and targeting the bone (Keliher et al. Citation2011). Nanogels demonstrate several advantages as drug carriers such as high drug loading potential, dispersion stability, stability following freeze-dry process and ease of drug formulation. The ionic interactions between negatively charged oligonucleotides and positively charged amines of PEI molecules in cationic nanogels are usually formed spontaneously during some minutes (Vinogradov Citation2007). Conjugation of PEG to PEI has significantly decreased the cytotoxic effects of cationic charge of PEI that results in generation of non-toxic nanogels (Kircheis et al. Citation2001). Though nanogels have usually a shorter plasma half-life compared to liposomes, they show a longer half-life compared to some other NP. Moreover, optimization of nanogels characteristics through modification of PEG content and particle size can significantly increase a plasma half-life of these NP (Oupicky et al. Citation2002).
The stable network of nanogels can provide a promising protection for labile drugs. Although the high molecular mass and small size of nanogels facilitates their passive targeting into favored sites, their surface modification can effectively conduct drug-loaded nanogels to specific sites. Surface modification of nanogels with various targeting molecules such as folate (Nayak et al. Citation2004), targeting proteins (Vinogradov & Kabanov Citation2004) and EGF receptor-binding peptides (Lutsenko et al. Citation2002) was associated with better outcomes. Moreover, there are studies which indicate the CNS drug delivery potential of nanogels such as PEG-PEI (Vinogradov et al. Citation1999) and N-vinyl-pyrrolidone/N-isopropyl-acrylamide (Soni et al. Citation2006). It has been shown that conjugation of nanogels to transferrin, insulin or brain-specific homing peptides can effectively enhance the transport of drugs across the BBB of mice (Vinogradov et al. Citation2004; Vinogradov Citation2007). While nanogels have not yet been analyzed in humans, they are made of FDA-approved materials that can facilitate preparing processes for ultimate use in humans.
Carbon nanomaterials
It has been shown that carbon nano-formulations can be used for various applications such as cancer diagnosis, imaging, targeted photothermal therapy, photoacoustic imaging, drug delivery and tissue engineering (Zhang et al. Citation2014). Carbon NP can be classified into two groups, including carbon nanotubes (CNT) and carbon nanohorns (CNH). CNT which are one-dimensional graphitic materials with unique structure, thermal and electrical features can be categorized into two subsets including single-walled and multi-walled CNT, with diameters of 1–2 nm and lengths from 50–1000 nm (Monaco & Giugliano Citation2014). Various methods such as arc discharge, laser ablation, high-pressure carbon monoxide disproportionation and chemical vapor deposition are used to produce CNT (Battigelli et al. Citation2013). It has been suggested that surface modification of CNT with PAMAM dendrimers can improve their biocompatibility and function (Michaelis et al. Citation2006). Moreover, conjugation of targeting molecules such as EGF onto CNT was also associated with selective action of these nano-formulations, in vitro (Karmakar et al. Citation2011). Chemical adsorption and especially encapsulation are common methods used for drug loading in CNT.
CNH, a type of single-layer nanotubes, share similar features with nanotubes; however, their production cost is lower than CNT and they have sizes of ≈40–50 nm in tubule length and ≈2–3 nm in diameter (Zhang et al. Citation2014). CNH can be classified into three groups: “dahlia-like”, “bud-like” and “seed-like” NP with horn-shaped tips. CNH are generated with high purity through CO2 laser ablation and arc discharge without a metal catalyst. Thus, pure samples of CNH are available more easily than are pure samples of CNT. Moreover, the high surface areas and frequent horn interstices of CNH facilitate the adsorption of large amounts of drugs onto them (Zhu & Xu Citation2010). Nano-precipitation and to lesser extent surface adsorption are selective drug loading methods for CNH (Kempiński et al. Citation2014). Although application of CNT was associated with neuroprotective effects in both in vitro (Claire du Toit et al. Citation2007) and in vivo (Yang et al. Citation2010) studies, their use as a drug carrier is limited, which is in part due to their high toxicity. The high toxicity of CNT is related to their geometric structure and impurities, such as residual metal and amorphous carbon (Hosseini et al. Citation2015).
A combination of carbon NP and magnetic NP is also proving to be a novel promising approach for biomedical applications. Their combination has been showed a synergistic effect; however, these joint usages still need to be investigated in further studies (Chau et al. Citation2015). It seems that for any clinical application of carbon nanomaterials, several lines of pre-clinical as well as trial studies are clearly necessary.
Nanoparticles in MS
Diagnosis
One of the important issues in the treatment of MS patients is determination of disease stage and neuroinflammatory process. Therefore, we need potent tools to investigate the precise immunopathologic condition, before treatment initiation. As mentioned previously, different immune cells can infiltrate into the CNS and induce neuroinflammation. Although immunohistologic assays can present precise data regarding the nature of lesions, their resolution is limited to small lesion area at one time. On the other hand, using magnetic resonance imaging (MRI), one can follow lesion generation and development over time in several areas. However, non-specific proton changes arising due to non-neuroinflammatory processes may alter signals resulted by T2-weighted MRI. Identification of NP led to improving novel imaging methods, which allow us to specifically detect cellular dependent neuroinflammatory process in the CNS. The gold NP-dependent biobarcode technique is a potent amplifying method to detect very low molecular signals in CSF of neurologic disorders (Georganopoulou et al. Citation2005). Since a few radio-diagnostic approaches are available for detection of MS lesions, including MRI, SWIP, Eco-doppler, application of such a NP-based methods able to detect and quantify ultralow signals in MS lesions could be useful in MS monitoring (Warntjes et al. Citation2008). Use of anisotropic silver NP (AgNP) in localized surface plasmon resonance is another application of NP in visualization of MS lesions that helps to detect very weak signals. In this technique, the ultralow interaction of AgNP-protein induces perturbations in the refractive index of the surrounding magnetic field that, in turn, are detected (Haes et al. Citation2005). There is also another NP-based monitoring method known as atomic force or scanning force microscopy that is a very high-resolution type of scanning probe microscopy for resolution of fractions of a nanometer (Lamprecht et al. Citation2014).
Although gadolinium-DTPA is commonly used for detection of neuroinflammatory process in MS lesions, new emerging approaches such as super-paramagnetic iron oxide particles (SPION) can be used to demonstrate immune cells infiltration in MS lesions (Zhao et al. Citation2014; Neuwelt et al. Citation2015). SPION have a 4–8 nm iron core surrounded with a dextran layer, with overall particle size of 50–150 nm. On the other hand, ultra-small super-paramagnetic iron oxide particles (USPION) have been created, which have smaller overall particle size of 10–50 nm. Following systemic administration of these paramagnetic NP, they will be phagocytosed by macrophages and also to some extent cleared by reticuloendothelial system in the liver and spleen. After the migration of labeled macrophages into the CNS, these NP can be visualized in vivo by MRI (Stoll & Bendszus Citation2010). Using USPION, researchers have been successfully visualized the infiltration of macrophages, CD4+ and CD8+ T-cells in brain of rat models of amyotrophic lateral sclerosis (ALS) (Machtoub et al. Citation2010; Bataveljić et al. Citation2011). USPION could also help with neuroimaging and precise visualization of inflammatory sites in the brains of EAE animal models (Stoll & Bendszus Citation2010; Hunger et al. Citation2014). It has also been possible to connect SPION through their surface conjugation with targeting molecules such as antibodies against anti-E-selection, vascular cell-adhesion molecule (VCAM) and specific neurotransmitter receptors that are involved in neuroinflammatory processes in MS (Weinstein et al. Citation2010).
Super-paramagnetic iron oxide particles (SPION) can also be useful in therapeutic approaches through their surface modification. Conjugation of environmental stimuli-sensitive polymers on the surface of paramagnetic polymers can make them useful as therapeutic devices. These therapeutic paramagnetic NP are known as “Smart” SPION. Different MS drugs such as IFNβ can be loaded in smart SPION using these surface conjugated polymers. The different environmental conditions in normal and inflamed tissues, for example the lower pH in inflamed tissues, can be used a drug-release mechanism for injured tissues. Several stimuli-sensitive polymers such as triblock poly(styrene-block2-vinylpyridine-block-ethylene oxide), poly(vinylmethylsiloxane), poly(N-isopropylacryla-mide), polystyrene-poly(methylmethacrylate) and polystyrenepoly(methylmethacrylate) can be used for surface conjugation with SPION (Mahmoudi et al. Citation2011).
Proteinticles are other useful tools in the diagnosis of MS. Proteinticles are nanoscale particles with constant structure and surface topology, which are self-assembled inside cells. Through addition or insertion of different peptides in different regions of these particles, several functional proteinticles can be easily synthesized. Lee et al. (Citation2013) generated proteinticles composed of the extracellular domain of MOG that distinguish autoantibodies on the surface of human ferritin-based proteinticles for the 3D diagnosis of MS.
In spite of several diagnostic and therapeutic advantages of paramagnetic NP in MS, it is suggested that the use of these NP may change the physiologic function of labeled cells and MS clinical outcomes. It has been demonstrated that phagocytosis of paramagnetic NP by macro-phages of mouse and rat leads to phenotype shift in macrophages toward anti-inflammatory M2 phenotype, which was associated with increased production of IL-10 and decreased TNFα, in vitro (Siglienti et al. Citation2006), however, there are contradictory results (Hsiao et al. Citation2008). Moreover, it is reported that while the administration of mesenchymal stem cells (MSC) can attenuate EAE progression, administration of SPION-labeled MSC into the same mice led to disease progression (Schäfer et al. Citation2008). Controversially, it is reported that transplantation of SPION-labeled bone marrow stroma cells-derived neural stem cells into the rhesus monkeys was safe and without side effects (Ke et al. Citation2009b). Thus, it seems that there is no consensus regarding the safety issues related to application of paramagnetic NP in the diagnosis and treatment of MS and this field needs to further investigations.
There are other novel NP-based monitoring approaches, such as bioconjugated tau protein AuNP, multipartite NP and quantum dots (QD) that show investigation in this field is ongoing and calls for further studies (Neely et al. Citation2009).
Treatment
In addition to diagnostic applications of NP in MS, they can also be considered as potent drug carriers in MS therapy (). There are several studies related to the capability of different NP in drug delivery in different pathologic conditions, particularly in neurologic disorders. These NP are mainly applied in two ways, including neuro-protection and anti-inflammation or both. Merodio et al. (Citation2000) documented the capability of albumin NP in crossing BBB and their brain distribution in EAE rat models, for the first time. The generated biotinylated NP were present in the lumbar portion of the spinal cord, overlying the meningeal and perivascular areas, optic chiasma, iris and the area of the Purkinje cells of the cerebellum. They also demonstrated that macrophages and microglial cells were found delivered albumin NP into the CNS. Moreover, Cavaletti et al. (Citation2009) showed that positively-charged liposomes targeted acute neuro-inflammatory areas in EAE rats.
Table 1. Studies related to the role of nanoparticles in the treatment of EAE and MS.
As neuroprotective therapy, Basso et al. (Citation2008) used N-methyl-D-aspartate (NMDA) receptor antagonist conjugated-ABS-75 compound (a fullerene derivative) to treat chronic progressive myelin-oligodendrocyte glycoprotein (MOG) induced-EAE in NOD mice. These compounds could exert both anti-oxidant and anti-excitotoxic functions. Those authors showed that treatment of mice with this NP following disease onset could effectively decrease EAE progression; this effect was correlated with decreased axonal loss and demyelination in the spinal cord. While this drug significantly decreased oxidative stress, infiltration of CD11b+ cells and CCL2 generation in the spinal cord of EAE mice, it had no interference with antigen-specific T-cell responses. Moreover, the in vitro analysis of ABS-75 compound further substantiated its neuroprotective and anti-oxidative effects in part through protection of neurons against glutamate-induced injury and restoration of glutamine synthetase and glutamate transporter expression in astrocytes in neuroinflammatory condition. That study suggested that fullerene and its derivatives might be considered neuroprotective anti-oxidative agents in the treatment of EAE and MS. Fullerenes are carbon spheres that have unique electronic features that make them useful device for diagnostic and therapeutic applications (Dellinger et al. Citation2013). Moreover, Rittchen et al. (Citation2015) recently demonstrated that PLGA-based NP targeted with antibodies against NG-2 chondroitin sulfate proteoglycan can specifically deliver leukemia inhibitory factor (LIF), a cytokine with pro-myelination potential, to oligodendrocyte precursor cells (OPC) to promote their differentiation to mature oligodendrocytes that are able to repair myelin in vivo.
A recent study demonstrated that combination therapy with lenalidomide and anti-oxidant cerium oxide NP (nano-ceria) decreased demyelination and neurological symptoms in EAE mice and provided a potential application in the treatment of MS (Eitan et al. Citation2015). It has also been reported that tempamine-loaded PEGylated nanoliposomes could effectively inhibit EAE in mice. It should be noted that tempamine is a stable radical with anti-oxidant and pro-apoptotic features. It have been shown that 3 wt% tempamine-loaded NP could reach the brain of EAE mice compared to only a level of 1 wt% in healthy control mice (Kizelsztein et al. Citation2009).
Jenkins et al. (2011) tried to use magnetic NP for gene delivery to rat oligodendrocyte precursor cells derived for transplants. They showed that transfection efficacy of therapeutic genes to oligodendrocyte precursors using magnetic NP was significantly better than other common non-viral transfection methods such as electroporation and lipofection. Moreover, gene transfer by magnetic NP has no adverse effects on cell division. Using brain slices as the host tissue in 3D tissue engineering models, it has shown that transfected cells had high trans-plantation capacity, as they had ability to migrate, divide, produce to daughter cells and integrate within host tissue. Regarding the results reported in that study, it seemed magnetic NP could be a potent alternative to common approaches for gene delivery to oligodendrocyte precursor cells.
Since cerium oxide NP can fluctuate between the +3 and +4 valence states, they can give or receive electrons. Thus, they can be considered as potent anti-oxidative agents in MS therapy. It has recently been shown that the intravenous administration of cerium oxide NP into EAE mice led to reduction of reactive oxygen species levels and disease attenuation. Thus, it seems that cerium oxide NP may be effective in the MS therapy in part through reduction of oxidative stress process (Heckman et al. Citation2013). Similarly, it has been demonstrated that cerium and yttrium oxide (CeO2 and Y2O3) NP could effectively reduce the oxidative stress in hippocampal neuronal cells (Schubert et al. Citation2006) and in the rat hippocampus (Hosseini et al. Citation2014).
It has been suggested that dendrimers can target both microglia and astrocytes that are crucial cells involved in neuroinflammatory processes in neurological disorders like MS. The subarachnoid administration of fluorescent-labeled neutral generation-4-PAMAM dendrimers to a rabbit model of cerebral palsy was associated with localization of these small NP in activated microglia and astrocytes (Dai et al. Citation2010). Thus, dendrimers might be considered potent anti-inflammatory drug carriers to treat neurological disorders like MS. Microglia and astrocytes play an important role in the pathogenesis of cerebral palsy, a chronic neurologic childhood disorder. It was reported that systemic administration of PAMAM dendrimers led to localization of these NP in activated microglia and astrocytes in the brain of rabbit models of cerebral palsy. Further, administration of N-acetyl-l-cysteine (NAC)-loaded dendrimers suppressed neuroinflammation and increased motor function (Kannan et al. Citation2012).
Interestingly, it has been reported that the gold NP can be used for tolerance induction through expansion of regulatory T (Treg)-cells in EAE mice. Yeste et al. (Citation2012) showed that treatment of DC with 2-(1'H-indole-3'-carbonyl)-thiazole-4-carboxylic acid methyl ester (ITE) and a T-cell epitope from MOG (35–55)-loaded gold NP stabilized with a layer of PEG molecule led to induction of Treg cells in vitro. Furthermore, administration of these NP into EAE mice was associated with disease attenuation and Treg population expansion. These reports implied this was a novel method for control of disease progression through induction of Treg cells by NP. Similarly, other investigators analyzed the efficiency of myelin basic protein (MBP)-derived peptides loaded in the monolayer mannosylated liposomes on the progression of EAE in DA rats. They showed that administration of MBP peptides (46–62, 124–139 and 147–160) incorporated into liposomes to DA rats inhibited disease progression and decreased level of anti-MBP antibodies (Yeste et al. Citation2012). Furthermore, administration of proteolipid protein (PLP)-conjugated PLGA NP to EAE mice could effectively inhibit disease induction (Eaton et al. Citation2013). Similarly, vaccination of EAE mice with PLGA-NP loaded with the MOG35–55 autoantigen and recombinant IL-10 was associated with ameliorating effects (Cappellano et al. Citation2014).
As the high-dose glucocorticosteroid hormones are a mainstay in the treatment of relapses in MS, Schmidt and coworkers developed long-circulating PEG-coated prednisolone liposomes. The intravenous administration of these NP into EAE rat models was associated with increased accumulation of prednisolone in the CNS and spinal cord of EAE animals compared to healthy controls (Schmidt et al. Citation2003). In another study, Linker and colleagues have compared the efficiency of liposomal prednisolone to liposomal methylprednisolone in chronic-relapsing MOG-induced EAE model. They showed that methylprednisolone liposomes were superior to prednisolone-containing liposomes and free methylprednisolone in EAE mitigation (Linker et al. Citation2008). It has been demonstrated that minocycline loaded PEGylated-liposomes could attenuate the CNS autoimmune disease (Hu et al. Citation2009).
Most recently, Yuan et al. (Citation2014) produced a novel NP composed of a Tat49-57 cationic peptide and MOG33-35, accompanied with the plasmid containing murine B- and T-lymphocyte attenuator (BTLA) gene. It is suggested that BTLA may have a modulatory role in maintaining peripheral tolerance. They showed that the administration of NP-loaded DC could induce immune tolerance in CD4+ T-cells and inhibit EAE progression. Similarly, Sestak et al. (2014) reported how the physical characteristics of the carrier such as structure, size and solubility can modulate the immune response following treating EAE. They evaluated a bifunctional peptide (small and soluble), Soluble Antigen Arrays (SAgAs) (large and soluble) and PLGA NP (large and insoluble) all exhibiting PLP and intercellular cell adhesion molecule-1 ligand derived from αL-integrin (CD11a237–246). These authors reported the simultaneous presence of both peptides on a soluble carrier exert the highest EAE suppression.
Hunter et al. (Citation2014) used the PLGA NP for the induction of antigen-specific T-cell tolerance in relapsing-remitting EAE mice. They showed that myelin antigen-conjugated NP could effectively attenuate disease symptoms. Ameliorative effects of NP were associated with significant reduction in infiltration of encephalitogenic TH1 and TH17 cells as well as inflammatory monocytes/macrophages into the CNS. In addition, because infection with Helicobacter pylori could protect MS patients against disease progression (Li et al. Citation2007), it was hypothesized that immunogenic components derived from H. pylori packaged in NP – which are targeted for the CNS – might also alleviate symptoms of MS (Pezeshki et al. Citation2008). To date, this strategy has not been reported on in the literature.
Interestingly, DC can be considered as a therapeutic target in disease treatment with NP. Since the tolerogenic function of Treg cells in autoimmune diseases is usually impaired, induction of functional Treg cells could represent a potential therapy in treatment of autoimmune disorders, such as MS. Manipulation of DC is appropriate approach for induction of Treg cells. As discussed earlier, it was reported that activation of ligand-activated transcription factor aryl hydrocarbon receptor by ITE or other ligands led to generation of DC that induced Treg cell development. Yeste et al. (Citation2012) showed that co-administration of ITE and MOG35–55 (through NP) into DC enhanced the generation of Treg cells. These authors also reported that NP-treated DC could induce Treg cells in vitro and suppress development of EAE. It was also reported that administration of DC treated with NP containing MOG and co-inhibitory receptor BTLA into EAE mice led to an up-regulation of Treg cells that was associated with increased IL-10 and TGFβ generation and decreased IL-2 and IFNγ production. While these manipulated DC inhibited the T-cell response to MOG, immune responses to irrelevant exogenous antigens remained intact. In another study, NP-treated DC suppressed cell infiltration into the spinal cord and attenuated EAE development (Yuan et al. Citation2014). Gammon et al. (Citation2015) suggested that treatment of DC with NP containing N-phenyl-7-(hydroxyimino)-cyclopropa-[b]chromen-1a-carboxamide (PHCCC) could skew the balance status between Treg and TH17 cells toward Treg cells which leads to protection in EAE mice. Taken together, these studies highlight the potential to induce tolerance through manipulation of DC by NP containing tolerogenic factors so as to polarize T-cells toward Treg cells.
In addition to drug delivery, it has been shown that nanomedicine can also be useful in neurosurgery. In addition to clearing tumor cells, nanosurgery can also help to nano-imaging and tumor monitoring. It was suggested that magnetic NP such as cytobots and karyobots might enhance re-myelination and inhibit disease progression (Freitas Citation2005; Khawaja Citation2011). Recent advances showed that some NP such as QD, femtosecond pulses of near-infrared laser, multipartite NP and atomic force microscopy cantilever have made it possible to perform nanosurgery at the single-cell level. The atomic force microscopy tips are supplied with ultrathin needles 200–300 nm in diameter that can penetrate the cell membrane upon indentation to a depth of 1–2 μm. A 3D imaging by confocal microscopy demonstrated that the needle could penetrate both the cellular and nuclear membranes (Obataya et al. Citation2005; Jeffries et al. Citation2007).
Conclusions
Drug delivery into the CNS is the most prominent problem in treatment of MS. However, recent advances in development of various nanoscale materials, which have high capacity in targeted drug delivery have opened new window in treatment of the CNS-related diseases such as MS. As mentioned, nanomedicine could be useful in MS through different ways, including neuroimaging (diagnosis), drug delivery and nanosurgery. According to the data reported by Cavaletti et al. (Citation2009), cationic NP may penetrate BBB better than anionic NP. Moreover, it was reported that conjugation of NP with molecules such as monoclonal antibodies (OX26, 8D3 and 83-14), CCP, apolipoproteins, thiamine, transferrin, folate, glycosides or lactoferrin could specifically conduct NP toward the CNS. Unfortunately, the majority of studies are limited to EAE animal models and little in known regarding the therapeutic efficacy of NP in MS (). Thus, before designing any NP-based therapeutic methods for MS patients, more investigations are necessary.
Disclosure statement
The authors report no conflicts of interest. The authors alone are responsible for the content of this review.
References
- Agarwal A, Agrawal H, Tiwari S, Jain S, Agrawal GP. 2011. Cationic ligand appended nanoconstructs: A prospective strategy for brain targeting. Int JPharm. 421:189–201.
- Allen T, Hansen C, Guo L. 1993. Subcutaneous administration of liposomes: A comparison with the intravenous and intraperitoneal routes of injection. Biochim Biophys Acta Biomemb. 1150:9–16.
- Arseneault M, Wafer C, MORIN J-F. 2015. Recent advances in click chemistry applied to dendrimer synthesis. Molecules. 20:9263–9294.
- Bahbouhi B, Pettré S, Berthelot L, Garcia A, Ngono AE, Degauque N, Michel L, Wiertlewski S, Lefrère F, Meyniel C. 2010. T-cell recognition of self-antigen presenting cells by protein transfer assay reveals a high frequency of anti-myelin T-cells in multiple sclerosis. Brain. 133:1622–1636.
- Bahrami B, Mohammadnia-Afrouzi M, Bakhshaei P, Yazdani Y, Ghalamfarsa G, Yousefi M, Sadreddini S, Jadidi-Niaragh F, Hojjat-Farsangi M. 2015. Folate-conjugated nanoparticles as a potent therapeutic approach in targeted cancer therapy. Tumor Biol. 36:5727–5742.
- Bangham A, Standish MM, Watkins J. 1965. Diffusion of univalent ions across the lamellae of swollen phospholipids. J Mol Biol. 13:238–252.
- Barenholz YC. 2012. Doxil®—the first FDA-approved nano-drug: Lessons learned. J Control Release. 160:117–134.
- Basso AS, Frenkel D, Quintana FJ, Costa-Pinto FA, Petrovic-Stojkovic S, Puckett L, Monsonego A, Bar-Shir A, Engel Y, Gozin M, et al. 2008. Reversal of axonal loss and disability in a mouse model of progressive multiple sclerosis. J Clin Invest. 118:1532.
- Bataveljić D, Stamenković S, Bačić G, Andjus PR. 2011. Imaging cellular markers of neuroinflammation in the brain of the rat model of amyotrophic lateral sclerosis. Acta Physiol Hungarica. 98:27–31.
- Batrakova EV, Han H-Y, Miller DW, Kabanov AV. 1998. Effects of pluronic P85 unimers and micelles on drug permeability in polarized BBMEC and Caco-2 cells. Pharm Res. 15:1525–1532.
- Batrakova EV, Kabanov AV. 2008. Pluronic block copolymers: Evolution of drug delivery concept from inert nanocarriers to biological response modifiers. J Control Release. 130:98–106.
- Battigelli A, Ménard-Moyon C, Da Ros T, Prato M, Bianco A. 2013. Endowing carbon nanotubes with biological and biomedical properties by chemical modifications. Adv Drug Deliv Rev 65:1899–1920.
- Béduneau A, Hindré F, Clavreul A, Leroux J-C, Saulnier P, Benoit J-P. 2008. Brain targeting using novel lipid nanovectors. J Control Release. 126:44–49.
- Béduneau A, Saulnier P, Hindré F, Clavreul A, Leroux J-C, Benoit J-P. 2007. Design of targeted lipid nanocapsules by conjugation of whole antibodies and antibody Fab’fragments. Biomaterials. 28:4978–4990.
- Beg S, Samad AI, Alam M, Nazish I. 2011. Dendrimers as novel systems for delivery of neuropharmaceuticals to the brain. CNS Neurol Disord Drug Targets. 10:576–588.
- Bencherif SA, Siegwart DJ, Srinivasan A, Horkay F, Hollinger JO, Washburn NR, Matyjaszewski K. 2009. Nanostructured hybrid hydrogels prepared by a combination of atom transfer radical polymerization and free radical polymerization. Biomaterials. 30:5270–5278.
- Bertero A, Boni A, Gemmi M, Gagliardi M, Bifone A, Bardi G. 2014. Surface functionalisation regulates polyamidoamine dendrimer toxicity on blood–brain barrier cells and the modulation of key inflammatory receptors on microglia. Nanotoxicology. 8:158–168.
- Bozzuto G, Molinari A. 2015. Liposomes as nanomedical devices. Int J Nanomed 10:975–981.
- Caporro M, Disanto G, Gobbi C, Zecca C. 2014. Two decades of subcutaneous glatiramer acetate injection: Current role of the standard dose, and new high-dose low-frequency glatiramer acetate in relapsing–remitting multiple sclerosis treatment. Patient Prefer Adherence. 8:1123–1134.
- Cappellano G, Woldetsadik AD, Orilieri E, Shivakumar Y, Rizzi M, Carniato F, Gigliotti CL, Boggio E, Clemente N, Comi C. 2014. Subcutaneous inverse vaccination with PLGA particles loaded with a MOG peptide and IL-10 decreases the severity of experimental autoimmune encephalomyelitis. Vaccine. 32:5681–5689.
- Cavaletti G, Cassetti A, Canta A, Galbiati S, Gilardini A, Oggioni N, Rodriguez-Menendez V, Fasano A, Liuzzi GM, Fattler U, et al. 2009. Cationic liposomes target sites of acute neuroinflammation in experimental autoimmune encephalomyelitis. Mol Pharmaceut. 6:1363–1370.
- Chattopadhyay N, Zastre J, Wong H-L, Wu XY, Bendayan R. 2008. Solid lipid nanoparticles enhance the delivery of the HIV protease inhibitor, atazanavir, by a human brain endothelial cell line. Pharm Res. 25:2262–2271.
- Chau NDQ, Ménard-Moyon C, Kostarelos K, Bianco A. 2015. Multifunctional carbon nanomaterial hybrids for magnetic manipulation and targeting. Biochem Biophys Res Commun. 468:454–462.
- Chen D-B, Yang T-Z, Lu W-L, Zhang Q. 2001. In vitro and in vivo study of two types of long-circulating solid lipid nanoparticles containing paclitaxel. Chem Pharm Bull. 49:1444–1447.
- Cheng M-R, Li Q, Wan T, He B, Han J, Chen H-X, Yang F-X, Wang W, Xu H-Z, Ye T. 2012. Galactosylated chitosan/5-fluorouracil nanoparticles inhibit mouse hepatic cancer growth and its side effects. World J Gastroenterol. 18:6076.
- Chowdhary RK, Chansarkar N, Sharif I, Hioka N, Dolphin D. 2003. Formulation of benzoporphyrin derivatives in pluronics. Photochem Photobiol. 77:299–303.
- Christenson RH, Cervelli DR, Bauer RS, Gordon M. 2004. Stratus® CS cardiac troponin I method: Performance characteristics including imprecision at low concentrations. Clin Biochem. 37:679–683.
- Claire du Toit L, Pillay V, Choonara YE, Pillay S, Harilall S-L. 2007. Patenting of nanopharmaceuticals in drug delivery: No small issue. Recent Patents Drug Deliv Formulation. 1:131–142.
- Cuzner ML, Gveric D, Strand C, Loughlin AJ, Paemen L, Opdenakker G, Newcombe J. 1996. The expression of tissue-type plasminogen activator, matrix metalloproteases and endogenous inhibitors in the central nervous system in multiple sclerosis: Comparison of stages in lesion evolution. J Neuropathol Exp Neurol. 55:1194–1204.
- Dai H, Navath RS, Balakrishnan B, Guru BR, Mishra MK, Romero R, Kannan RM, Kannan S. 2010. Intrinsic targeting of inflammatory cells in the brain by polyamidoamine dendrimers upon subarachnoid administration. Nanomedicine. 5:1317–1329.
- Danhier F, Lecouturier N, Vroman B, Jérôme C, Marchand-Brynaert J, Feron O, Préat V. 2009. Paclitaxel-loaded PEGylated PLGA-based nanoparticles: In vitro and in vivo evaluation. J Control Release. 133:11–17.
- Dash PR, Read ML, Fisher KD, Howard KA, Wolfert M, Oupicky D, Subr V, Strohalm J, Ulbrich K, Seymour LW. 2000. Decreased binding to proteins and cells of polymeric gene delivery vectors surface modified with a multivalent hydrophilic polymer and retargeting through attachment of transferrin. J Biol Chem. 275:3793–3802.
- De Jesus MB, Zuhorn IS. 2015. Solid lipid nanoparticles as nucleic acid delivery system: Properties and molecular mechanisms. J Control Release. 201:1–13.
- Dellinger A, Zhou Z, Connor J, Madhankumar A, Pamujula S, Sayes CM, Kepley CL. 2013. Application of fullerenes in nanomedicine: An update. Nanomedicine. 8:1191–1208.
- Demeule M, Currie JC, Bertrand Y, Ché C, Nguyen T, Régina A, Gabathuler R, Castaigne JP, Béliveau R. 2008. Involvement of the low‐density lipoprotein receptor‐related protein in the transcytosis of the brain delivery vector Angiopep‐2. J Neurochem. 106:1534–1544.
- Dhanikula RS, Argaw A, Bouchard J-F, Hildgen P. 2008. Methotrexate loaded polyether-copolyester dendrimers for the treatment of gliomas: Enhanced efficacy and intratumoral transport capability. Mol Pharm. 5:105–116.
- Dhawan S, Kapil R, Singh B. 2011. Formulation development and systematic optimization of solid lipid nanoparticles of quercetin for improved brain delivery. J Pharm Pharmacol. 63:342–351.
- Donaghue IE, Tam R, Sefton MV, Shoichet MS. 2014. Cell and biomolecule delivery for tissue repair and regeneration in the central nervous system. J Control Release. 190:219–227.
- Dunne M, Corrigan O, Ramtoola Z. 2000. Influence of particle size and dissolution conditions on the degradation properties of polylactide-co-glycolide particles. Biomaterials. 21:1659–1668.
- Eaton VL, Vasquez KO, Goings GE, Hunter ZN, Peterson JD, Miller SD. 2013. Optical tomographic imaging of near infrared imaging agents quantifies disease severity and immunomodulation of experimental autoimmune encephalomyelitis in vivo. J Neuroinflammation. 10:138.
- Eitan E, Hutchison ER, Greig NH, Tweedie D, Celik H, Ghosh S, Fishbein KW, Spencer RG, Sasaki CY, Ghosh P. 2015. Combination therapy with lenalidomide and nanoceria ameliorates CNS autoimmunity. Exp Neurol. 273:151–160.
- Elamanchili P, Lutsiak CM, Hamdy S, Diwan M, Samuel J. 2007. “Pathogen-mimicking” nanoparticles for vaccine delivery to dendritic cells. J Immunother. 30:378–395.
- Esfand R, Tomalia DA. 2001. Poly (amidoamine)(PAMAM) dendrimers: From biomimicry to drug delivery and biomedical applications. Drug Discov Today. 6:427–436.
- Foley CP, Nishimura N, Neeves KB, Schaffer CB, Olbricht WL. 2012. Real-time imaging of perivascular transport of nanoparticles during convection-enhanced delivery in the rat cortex. Ann Biomed Eng. 40:292–303.
- Freitas C, Müller RH. 1998. Effect of light and temperature on zeta potential and physical stability in solid lipid nanoparticle (SLN™) dispersions. Int J Pharm. 168:221–229.
- Freitas RA. 2005. Nanotechnology, nanomedicine and nanosurgery. Int J Surg. 3:243–246.
- Führmann T, Ghosh M, Otero A, Goss B, Pearse DD, Dalton PD. 2015. Peptide-functionalized polymeric nanoparticles for active targeting of damaged tissue in animals with experimental autoimmune encephalomyelitis. Neurosci Lett. 602:126–132.
- Fundarò A, Cavalli R, Bargoni A, Vighetto D, Zara GP, Gasco MR. 2000. Non-stealth and stealth solid lipid nanoparticles (SLN) carrying doxorubicin: Pharmacokinetics and tissue distribution after iv administration to rats. Pharmacol Res. 42:337–343.
- Gammon JM, Tostanoski LH, Adapa AR, Chiu Y-C, Jewell CM. 2015. Controlled delivery of a metabolic modulator promotes regulatory T cells and restrains autoimmunity. J Control Release. 210:169–178.
- Ganta S, Deshpande D, Korde A, Amiji M. 2010. A review of multifunctional nanoemulsion systems to overcome oral and CNS drug delivery barriers. Mol Membr Biol. 27:260–273.
- Ganta S, Paxton JW, Baguley BC, Garg S. 2008. Pharmacokinetics and pharmacodynamics of chlorambucil delivered in parenteral emulsion. Int J Pharm. 360:115–121.
- Ganta S, Singh A, Kulkarni P, Keeler AW, Piroyan A, Sawant RR, Patel NR, Davis B, Ferris C, O’Neal S. 2015. EGFR targeted theranostic nanoemulsion for image-guided ovarian cancer therapy. Pharm Res 32:2753–2763.
- Gao H, Pang Z, Jiang X. 2013. Targeted delivery of nano-therapeutics for major disorders of the central nervous system. Pharm Res 30:2485–2498.
- Gao X, Wu B, Zhang Q, Chen J, Zhu J, Zhang W, Rong Z, Chen H, Jiang X. 2007. Brain delivery of vasoactive intestinal peptide enhanced with the nanoparticles conjugated with wheat germ agglutinin following intranasal administration. J Control Release. 121:156–167.
- Georganopoulou DG, Chang L, Nam J-M, Thaxton CS, Mufson EJ, Klein WL, Mirkin CA. 2005. Nanoparticle-based detection in cerebral spinal fluid of a soluble pathogenic biomarker for Alzheimer's disease. Proc Natl Acad Sci USA. 102:2273–2276.
- Ghabaee M, Jabedari B, Al-E-Eshagh N, Ghaffarpour M, Asadi F. 2010. Serum and cerebrospinal fluid antioxidant activity and lipid peroxidation in Guillain-Barre syndrome and multiple sclerosis patients. Int J Neurosci. 120:301–304.
- Ghalamfarsa G, Mahmoudi M, Mohammadnia-Afrouzi M, Yazdani Y, Anvari E, Hadinia A, Ghanbari A, Setayesh M, Yousefi M, Jadidi-Niaragh F. 2015. IL-21 and IL-21 receptor in the immunopathogenesis of multiple sclerosis. J Immunotoxicol. [Epub ahead of print].
- Gharibi T, Ahmadi M, Seyfizadeh N, Jadidi-Niaragh F, Yousefi M. 2015. Immunomodulatory characteristics of mesenchymal stem cells and their role in the treatment of multiple sclerosis. Cell Immunol. 293:113–121.
- Ginocchio CC. 2011. Strengths and weaknesses of FDA-approved/cleared diagnostic devices for the molecular detection of respiratory pathogens. Clin Infect Dis. 52:S312–S325.
- Gonzalo H, Brieva L, Tatzber F, Jové M, Cacabelos D, Cassanyé A, Lanau‐Angulo L, Boada J, Serrano JC, González C. 2012. Lipidome analysis in multiple sclerosis reveals protein lipoxidative damage as a potential pathogenic mechanism. J Neurochem. 123:622–634.
- Gosselin MA, Lee RJ. 2002. Folate receptor-targeted liposomes as vectors for therapeutic agents. Biotechnol Annu Rev. 8:103–131.
- Graf A, Rades T, Hook SM. 2009. Oral insulin delivery using nanoparticles based on microemulsions with different structure-types: Optimization and in vivo evaluation. Eur J Pharm Sci. 37:53–61.
- Gupta Y, Jain A, Jain SK. 2007. Transferrin-conjugated solid lipid nanoparticles for enhanced delivery of quinine dihydrochloride to the brain. J Pharm Pharmacol. 59:935–940.
- Haes AJ, Chang L, Klein WL, van Duyne RP. 2005. Detection of a biomarker for Alzheimer's disease from synthetic and clinical samples using a nanoscale optical biosensor. J Am Chem Soc. 127:2264–2271.
- Hawker CJ, Frechet JM. 1990. Preparation of polymers with controlled molecular architecture. A new convergent approach to dendritic macromolecules. J Am Chem Soc. 112:7638–7647.
- Heckman KL, Decoteau W, Estevez A, Reed KJ, Costanzo W, Sanford D, Leiter JC, Clauss J, Knapp K, Gomez C. 2013. Custom cerium oxide nanoparticles protect against a free radical mediated autoimmune degenerative disease in the brain. ACS Nano. 7:10582–10596.
- Hemmer R, Hall A, Spaulding R, Rossow B, Hester M, Caroway M, Haskamp A, Wall S, Bullen HA, Morris C. 2013. Analysis of biotinylated generation 4 poly (amidoamine)(PAMAM) dendrimer distribution in the rat brain and toxicity in a cellular model of the blood-brain barrier. Molecules. 18:11537–11552.
- Hoepner R, Faissner S, Salmen A, Gold R, Chan A. 2014. Efficacy and side effects of natalizumab therapy in patients with multiple sclerosis. J Central Nerv Syst Dis. 6:41–49.
- Høglund RA, Maghazachi AA. 2014. Multiple sclerosis and the role of immune cells. World J Exp Med. 4:27–37.
- Hosseini A, Sharifi AM, Abdollahi M, Najafi R, Baeeri M, Rayegan S, Cheshmehnour J, Hassani S, Bayrami Z, Safa M. 2014. Cerium and yttrium oxide nanoparticles against lead-induced oxidative stress and apoptosis in rat hippocampus. Biol Trace Element Res. 164:80–89.
- Hosseini M, Haji-Fatahaliha M, Jadidi-Niaragh F, Majidi J, Yousefi M. 2015. The use of nanoparticles as a promising therapeutic approach in cancer immunotherapy. Artif Cells Nanomed Biotechnol. [Epub ahead of print].
- Hsiao JK, Chu HH, Wang YH, Lai CW, Chou PT, Hsieh ST, Wang JL, Liu HM. 2008. Macrophage physiological function after superparamagnetic iron oxide labeling. NMR Biomed. 21:820–829.
- Hu W, Metselaar J, Ben L-H, Cravens PD, Singh MP, Frohman EM, Eagar TN, Racke MK, Kieseier BC, Stüve O. 2009. PEG minocycline-liposomes ameliorate CNS autoimmune disease. PLoS One. 4:e4151.
- Huang R, Ke W, Liu Y, Jiang C, Pei Y. 2008. The use of lactoferrin as a ligand for targeting the polyamidoamine-based gene delivery system to the brain. Biomaterials. 29:238–246.
- Hunger M, Budinger E, Zhong K, Angenstein F. 2014. Visualization of acute focal lesions in rats with experimental autoimmune encephalomyelitis by magnetic nanoparticles, comparing different MRI sequences including phase imaging. J Magn Resonance Imaging. 39:1126–1135.
- Hunter Z, McCarthy DP, Yap WT, Harp CT, Getts DR, Shea LD, Miller SD. 2014. A biodegradable nanoparticle platform for the induction of antigen-specific immune tolerance for treatment of autoimmune disease. ACS Nano. 8:2148–2160.
- Hwang SR, Kim K. 2014. Nano-enabled delivery systems across the blood–brain barrier. Arch Pharm Res. 37:24–30.
- Illum L. 2004. Is nose‐to‐brain transport of drugs in man a reality? J Pharm Pharmacol. 56:3–17.
- Jackson AL, Linsley PS. 2010. Recognizing and avoiding siRNA off-target effects for target identification and therapeutic application. Nat Rev Drug Discov. 9:57–67.
- Jacobs LD, Cookfair DL, Rudick RA, Herndon RM, Richert JR, Salazar AM, Fischer JS, Goodkin DE, Granger CV, Simon JH. 1996. Intramuscular interferon beta-1a for disease progression in relapsing multiple sclerosis. Ann Neurol. 39:285–294.
- Jadidi-Niaragh F, Atyabi F, Rastegari A, Mollarazi E, Kiani M, Razavi A, Yousefi M, Kheshtchin N, Hassannia H, Hadjati J. 2016. Down-regulation of CD73 in 4T1 breast cancer cells through siRNA-loaded chitosan-lactate nanoparticles. Tumor Biol. [Epub ahead of print].
- Jadidi-Niaragh F, Mirshafiey A. 2010. Histamine and histamine receptors in pathogenesis and treatment of multiple sclerosis. Neuropharmacology. 59:180–189.
- Jadidi-Niaragh F, Mirshafiey A. 2011a. Regulatory T-cell as orchestra leader in immunosuppression process of multiple sclerosis. Immunopharmacol Immunotoxicol. 33:545–567.
- Jadidi-Niaragh F, Mirshafiey A. 2011b. TH17 cell, the new player of neuroinflammatory process in multiple sclerosis. Scand J Immunol. 74:1–13.
- Jadidi-Niaragh F, Mirshafiey A. 2011c. Therapeutic approach to multiple sclerosis by novel oral drugs. Recent Patents Inflamm Allergy Drug Discov. 5:66–80.
- Jadidi-Niaragh F, Mirshafiey A. 2012. The deviated balance between regulatory T-cell and TH17 in autoimmunity. Immunopharmacol Immunotoxicol. 34:727–739.
- Jang JS, Kim SY, Lee SB, Kim KO, Han JS, Lee YM. 2006. Poly (ethylene glycol)/poly (ɛ-caprolactone) diblock copolymeric nanoparticles for non-viral gene delivery: The role of charge group and molecular weight in particle formation, cytotoxicity and transfection. J Control Release. 113:173–182.
- Javaheri-Kermani M, Farazmandfar T, Ajami A, Yazdani Y. 2014. Impact of hepcidin antimicrobial peptide on iron overload in tuberculosis patients. Scand J Infect Dis. 46:693–696.
- Jeffries GD, Edgar JS, Zhao Y, Shelby JP, Fong C, Chiu DT. 2007. Using polarization-shaped optical vortex traps for single-cell nanosurgery. Nano Lett. 7:415–420.
- Jenkins SI, Pickard MR, Granger N, Chari DM. 2011. Magnetic nanoparticle-mediated gene transfer to oligodendrocyte precursor cell transplant populations is enhanced by magnetofection strategies. ACS Nano. 5:6527–6538.
- Kabanov AV, Batrakova EV, Miller DW. 2003. Pluronic® block copolymers as modulators of drug efflux transporter activity in the blood–brain barrier. Adv Drug Deliv Rev. 55:151–164.
- Kanazawa T. 2015. Brain delivery of small interfering ribonucleic acid and drugs through intranasal administration with nano-sized polymer micelles. Med Devices (Auckland, NZ). 8:57–64.
- Kanazawa T, Taki H, Tanaka K, Takashima Y, Okada, H. 2011. Cell-penetrating peptide-modified block copolymer micelles promote direct brain delivery via intranasal administration. Pharm Res. 28:2130–2139.
- Kannan S, Dai H, Navath RS, Balakrishnan B, Jyoti A, Janisse J, Romero R, Kannan RM. 2012. Dendrimer-based postnatal therapy for neuroinflammation and cerebral palsy in a rabbit model. Sci Transl Med. 4:130ra46.
- Kanwar JR, Sun X, Punj V, Sriramoju B, Mohan RR, Zhou S-F, Chauhan A, Kanwar RK. 2012. Nanoparticles in the treatment and diagnosis of neurological disorders: Untamed dragon with fire power to heal. Nanomed Nanotechnol Biol Med. 8:399–414.
- Karmakar A, Xu Y, Mahmood MW, Zhang Y, Saeed LM, Mustafa T, Ali S, Biris AR, Biris AS. 2011. Radio-frequency induced in vitro thermal ablation of cancer cells by EGF functionalized carbon-coated magnetic nanoparticles. J Mater Chem. 21:12761–12769.
- Kasper LH, Reder AT. 2014. Immunomodulatory activity of interferon‐beta. Ann Clin Transl Neurol. 1:622–631.
- Kaur IP, Bhandari R, Bhandari S, Kakkar V. 2008. Potential of solid lipid nanoparticles in brain targeting. J Control Release. 127:97–109.
- Ke W, Shao K, Huang R, Han L, Liu Y, Li J, Kuang Y, Ye L, Lou J, Jiang C. 2009a. Gene delivery targeted to the brain using an Angiopep-conjugated polyethyleneglycol-modified polyamidoamine dendrimer. Biomaterials. 30:6976–6985.
- Ke Y-Q, Hu C-C, Jiang X-D, Yang Z-J, Zhang H-W, Ji H-M, Zhou L-Y, Cai Y-Q, Qin L-S, Xu R-X. 2009b. In vivo magnetic resonance tracking of Feridex-labeled bone marrow-derived neural stem cells after autologous transplantation in rhesus monkey. J Neurosci Methods. 179:45–50.
- Keliher EJ, Yoo J, Nahrendorf M, Lewis JS, Marinelli B, Newton A, Pittet MJ, Weissleder R. 2011. 89Zr-labeled dextran nanoparticles allow in vivo macrophage imaging. Bioconjug Chem. 22:2383–2389.
- Kempiński W, Łoś S, Kempiński M, Markowski D. 2014. Experimental techniques for the characterization of carbon nanoparticles–a brief overview. Beilstein J Nanotechnol. 5:1760–1766.
- Kesharwani P, Tekade RK, Jain NK. 2014a. Formulation development and in vitro-in vivo assessment of the fourth-generation PPI dendrimer as a cancer-targeting vector. Nanomedicine. 9:2291–2308.
- Kesharwani P, Tekade RK, Jain NK. 2014b. Generation dependent cancer targeting potential of poly (propyleneimine) dendrimer. Biomaterials. 35:5539–5548.
- Khawaja AM. 2011. The legacy of nanotechnology: Revolution and prospects in neurosurgery. Int J Surg. 9:608–614.
- Kim D-H, Martin DC. 2006. Sustained release of dexamethasone from hydrophilic matrices using PLGA nanoparticles for neural drug delivery. Biomaterials. 27:3031–3037.
- Kim W, Zandoná ME, Kim S-H, Kim HJ. 2015. Oral disease-modifying therapies for multiple sclerosis. J Clin Neurol. 11:9–19.
- Kircheis R, Blessing T, Brunner S, Wightman L, Wagner E. 2001. Tumor targeting with surface-shielded ligand–polycation DNA complexes. J Control Release. 72:165–170.
- Kizelsztein P, Ovadia H, Garbuzenko O, Sigal A, Barenholz Y. 2009. Pegylated nanoliposomes remote-loaded with the anti-oxidant tempamine ameliorate experimental autoimmune encephalomyelitis. J Neuroimmunol. 213:20–25.
- Kobayashi H, Jo S-K, Kawamoto S, Yasuda H, Hu X, Knopp MV, Brechbiel MW, Choyke PL, Star RA. 2004. Polyamine dendrimer-based MRI contrast agents for functional kidney imaging to diagnose acute renal failure. J Magn Resonan Imaging. 20:512–518.
- Kokhaei P, Jadidi-Niaragh F, Sotoodeh Jahromi A, Osterborg A, Mellstedt H, Hojjat-Farsangi M. 2015. Ibrutinib-A double-edge sword in cancer and autoimmune disorders. J Drug Target. [Epub ahead of print].
- Koshkaryev A, Piroyan A, Torchilin VP. 2012. Increased apoptosis in cancer cells in vitro and in vivo by ceramides in transferrin-modified liposomes. Cancer Biol Ther. 13:50–60.
- Kothamasu P, Kanumur H, Ravur N, Maddu C, Parasuramrajam R, Thangavel S. 2012. Nanocapsules: The weapons for novel drug delivery systems. BioImpacts. 2:71–81.
- Krishnamachari Y, Geary SM, Lemke CD, Salem AK. 2011. Nanoparticle delivery systems in cancer vaccines. Pharm Res. 28:215–236.
- Kumar M, Misra A, Mishra A, Mishra P, Pathak K. 2008. Mucoadhesive nanoemulsion-based intranasal drug delivery system of olanzapine for brain targeting. J Drug Target 16:806–814.
- Kuo Y-C, Chen H-H. 2006. Effect of nanoparticulate polybutylcyanoacrylate and methylmethacrylate–sulfopropylmethacrylate on the permeability of zidovudine and lamivudine across the in vitro blood–brain barrier. Int J Pharm. 327:160–169.
- Lamprecht C, Hinterdorfer P, Ebner A. 2014. Applications of biosensing atomic force microscopy in monitoring drug and nanoparticle delivery. Expert Opin Drug Deliv. 11:1237–1253.
- Landau AJ, Eberhardt RT, Frishman WH. 1994. Intranasal delivery of cardiovascular agents: An innovative approach to cardiovascular pharmacotherapy. Am Heart J. 127:1594–1599.
- Leamon CP, Low PS. 2001. Folate-mediated targeting: From diagnostics to drug and gene delivery. Drug Discov Today. 6:44–51.
- Lee J-H, Seo HS, Song JA, Kwon KC, Lee EJ, Kim HJ, Lee EB, Cha YJ, Lee J. 2013. Proteinticle engineering for accurate 3D diagnosis. ACS Nano. 7:10879–10886.
- Lee J, Nikolov A, Wasan D. 2014. Surfactant micelles containing solubilized oil decrease foam film thickness stability. J Colloid Interface Sci. 415:18–25.
- Letchford K, Burt H. 2007. A review of the formation and classification of amphiphilic block copolymer nanoparticulate structures: Micelles, nanospheres, nanocapsules and polymersomes. Eur J Pharm Biopharm. 65:259–269.
- Li W, Minohara M, Su JJ, Matsuoka T, Osoegawa M, Ishizu T, Kira J-I. 2007. Helicobacter pylori infection is a potential protective factor against conventional multiple sclerosis in the Japanese population. J Neuroimmunol. 184:227–231.
- Libbey JE, Cusick MF, Fujinami RS. 2014. Role of pathogens in multiple sclerosis. Int Rev Immunol. 33:266–283.
- Linker RA, Weller C, Lühder F, Mohr A, Schmidt J, Knauth M, Metselaar JM, Gold R. 2008. Liposomal glucocorticosteroids in treatment of chronic autoimmune demyelination: Long-term protective effects and enhanced efficacy of methylprednisolone formulations. Exp Neurol. 211:397–406.
- Liu H, Wang H, Yang W, Cheng Y. 2012. Disulfide cross-linked low generation dendrimers with high gene transfection efficacy, low cytotoxicity, and low cost. J Am Chem Soc. 134:17680–17687.
- Liu Y, Li J, Shao K, Huang R, Ye L, Lou J, Jiang C. 2010. A leptin derived 30-amino-acid peptide modified pegylated poly-L-lysine dendrigraft for brain targeted gene delivery. Biomaterials. 31:5246–5257.
- Lockman PR, Oyewumi MO, Koziara JM, Roder KE, Mumper RJ, Allen DD. 2003. Brain uptake of thiamine-coated nanoparticles. J Control Release. 93:271–282.
- Lu C-T, Zhao Y-Z, Wong HL, Cai J, Peng L, Tian X-Q. 2014. Current approaches to enhance CNS delivery of drugs across the brain barriers. Int J Nanomedicine. 9:2241–2257.
- Lutsenko S, Feldman N, Severin S. 2002. Cytotoxic and anti-tumor activities of doxorubicin conjugates with the epidermal growth factor and its receptor-binding fragment. J Drug Target. 10:567–571.
- Machtoub L, Pfeiffer R, Backovic A, Frischauf S, Wick MC. 2010. Molecular imaging cellular SPIO uptake with nonlinear optical microscopy. J Med Imaging Radiat Sci. 41:159–164.
- Madaan K, Kumar S, Poonia N, Lather V, Pandita D. 2014. Dendrimers in drug delivery and targeting: Drug-dendrimer interactions and toxicity issues. J Pharmacy Bioallied Sci. 6:139–150.
- Mahmoudi M, Sahraian MA, Shokrgozar MA, Laurent S. 2011. Superparamagnetic iron oxide nanoparticles: Promises for diagnosis and treatment of multiple sclerosis. ACS Chem Neurosci. 2:118–140.
- Makadia HK, Siegel SJ. 2011. Poly lactic-co-glycolic acid (PLGA) as biodegradable controlled drug delivery carrier. Polymers. 3:1377–1397.
- Marcato PD, Durán N. 2008. New aspects of nanopharmaceutical delivery systems. J Nanosci Nanotechnol. 8:2216–2229.
- Maruyama K, Takahashi N, Tagawa T, Nagaike K, Iwatsuru M. 1997. Immunoliposomes bearing polyethyleneglycol-coupled Fab′ fragment show prolonged circulation time and high extravasation into targeted solid tumors in vivo. FEBS Lett. 413:177–180.
- Menjoge AR, Kannan RM, Tomalia DA. 2010. Dendrimer-based drug and imaging conjugates: Design considerations for nanomedical applications. Drug Discov Today. 15:171–185.
- Merodio M, Irache JM, Eclancher F, Mirshahi M, Villarroya H. 2000. Distribution of albumin nanoparticles in animals induced with the experimental allergic encephalomyelitis. J Drug Target. 8:289–303.
- Michaelis K, Hoffmann MM, Dreis S, Herbert E, Alyautdin RN, Michaelis M, Kreuter J, Langer K. 2006. Covalent linkage of apolipoprotein E to albumin nanoparticles strongly enhances drug transport into the brain. J Pharmacol Exp Ther. 317:1246–1253.
- Miljković D, Spasojević I. 2013. Multiple sclerosis: Molecular mechanisms and therapeutic opportunities. Antioxidants Redox Signal. 19:2286–2334.
- Mirshafiey A, Asghari B, Ghalamfarsa G, Jadidi-Niaragh F, Azizi G. 2014. The significance of matrix metalloproteinases in the immunopathogenesis and treatment of multiple sclerosis. Sultan Qaboos Univ Med J. 14:e13.
- Mirshafiey A, Jadidi-Niaragh F. 2010a. Immunopharmacological role of the leukotriene receptor antagonists and inhibitors of leukotrienes generating enzymes in multiple sclerosis. Immunopharmacol Immunotoxicol. 32:219–227.
- Mirshafiey A, Jadidi-Niaragh F. 2010b. Prostaglandins in pathogenesis and treatment of multiple sclerosis. Immunopharmacol Immunotoxicol. 32:543–554.
- Monaco AM, Giugliano M. 2014. Carbon-based smart nanomaterials in biomedicine and neuroengineering. Beilstein J Nanotechnol. 5:1849–1863.
- Muller RH, Mader K, Gohla S. 2000. Solid lipid nanoparticles (SLN) for controlled drug delivery – A review of the state of the art. Eur J Pharm Biopharm. 50:161–177.
- Nance EA, Woodworth GF, Sailor KA, Shih T-Y, Xu Q, Swaminathan G, Xiang D, Eberhart C, Hanes J. 2012. A dense poly-(ethylene glycol) coating improves penetration of large polymeric nanoparticles within brain tissue. Sci Transl Med. 4:149ra119.
- Nayak S, Lee H, Chmielewski J, Lyon LA. 2004. Folate-mediated cell targeting and cytotoxicity using thermoresponsive microgels. J Am Chem Soc. 126:10258–10259.
- Neely A, Perry C, Varisli B, Singh AK, Arbneshi T, Senapati D, Kalluri JR, Ray PC. 2009. Ultrasensitive and highly selective detection of Alzheimer’s disease biomarker using two-photon Rayleigh scattering properties of gold nanoparticle. ACS Nano. 3:2834–2840.
- Neuwelt A, Sidhu N, Hu C-AA, Mlady G, Eberhardt SC, Sillerud LO. 2015. Iron-based superparamagnetic nanoparticle contrast agents for MRI of infection and inflammation. Am J Roentgenol. 204:W302.
- Niu R, Zhao P, Wang H, Yu M, Cao S, Zhang F, Chang J. 2011. Preparation, characterization, and anti-tumor activity of paclitaxel-loaded folic acid modified and TAT peptide conjugated PEGylated polymeric liposomes. J Drug Target. 19:373–381.
- Niven RW, Speer M, Schreier H. 1991. Nebulization of liposomes. II. The effects of size and modeling of solute release profiles. Pharm Res. 8:217–221.
- Obataya I, Nakamura C, Han S, Nakamura N, Miyake J. 2005. Nanoscale operation of a living cell using an atomic force microscope with a nanoneedle. Nano Lett. 5:27–30.
- Ogris M, Brunner S, Schüller S, Kircheis R, Wagner E. 1999. PEGylated DNA/transferrin-PEI complexes: Reduced interaction with blood components, extended circulation in blood and potential for systemic gene delivery. Gene Ther. 6:595–605.
- Ortiz GG, Pacheco-Moisés FP, Bitzer-Quintero OK, Ramírez-Anguiano AC, Flores-Alvarado LJ, Ramírez-Ramírez V, Macias-Islas MA, Torres-Sánchez ED. 2013. Immunology and oxidative stress in multiple sclerosis: Clinical and basic approach. Clin Dev Immunol. 2013.
- Ortiz GG, Pacheco-Moisés FP, Macías-Islas MÁ, Flores-Alvarado LJ, Mireles-Ramírez MA, González-Renovato ED, Hernández-Navarro VE, Sánchez-López AL, Alatorre-Jiménez MA. 2014. Role of the blood-brain barrier in multiple sclerosis. Arch Med Res. 45:687–697.
- Oupicky D, Ogris M, Howard KA, Dash PR, Ulbrich K, Seymour LW. 2002. Importance of lateral and steric stabilization of polyelectrolyte gene delivery vectors for extended systemic circulation. Mol Ther. 5:463–472.
- Oyewumi MO, Liu S, Moscow JA, Mumper RJ. 2003. Specific association of thiamine-coated gadolinium nanoparticles with human breast cancer cells expressing thiamine transporters. Bioconj Chem. 14:404–411.
- Paiphansiri U, Tangboriboonrat P, Landfester K. 2007. Antiseptic nanocapsule formation via controlling polymer deposition onto water‐in‐oil miniemulsion droplets. Macromol Biosci. 6:33–40.
- Pardridge WM, Kang Y-S, Buciak JL, Yang, J. 1995. Human insulin receptor monoclonal antibody undergoes high affinity binding to human brain capillaries in vitro and rapid transcytosis through the blood–brain barrier in vivo in the primate. Pharm Res. 12:807–816.
- Pardridge WM, Wu D, Sakane T. 1998. Combined use of carboxyl-directed protein pegylation and vector-mediated blood-brain barrier drug delivery system optimizes brain uptake of brain-derived neurotrophic factor following intravenous administration. Pharm Res. 15:576–582.
- Parveen S, Sahoo SK. 2008. Polymeric nanoparticles for cancer therapy. J Drug Target. 16:108–123.
- Patlolla RR, Vobalaboina V. 2008. Folate-targeted etoposide-encapsulated lipid nanospheres. J Drug Target. 16:269–275.
- Patton D, Sweeney YC, Mccarthy T, Hillier S. 2006. Preclinical safety and efficacy assessments of dendrimer-based (SPL7013) microbicide gel formulations in a non-human primate model. Antimicrob Agents Chemother. 50:1696–1700.
- Pezeshki MZ, Zarrintan S, Zarrintan MH. 2008. Helicobacter pylori nanoparticles as a potential treatment of conventional multiple sclerosis. Med Hypotheses. 70:1223.
- Ponomarev ED, Shriver LP, Maresz K, Dittel BN. 2005. Microglial cell activation and proliferation precedes the onset of CNS autoimmunity. J Neurosci Res. 81:374–389.
- Popescu BFG, Lucchinetti CF. 2012. Pathology of demyelinating diseases. Annu Rev Pathol Mech Dis. 7:185–217.
- Prabhu RH, Patravale VB, Joshi MD. 2015. Polymeric nanoparticles for targeted treatment in oncology: Current insights. Int J Nanomedicine. 10:1001.
- Rajagopalan R, Xavier J, Rangaraj N, Rao NM, Gopal V. 2007a. Recombinant fusion proteins TAT-Mu, Mu and Mu-Mu mediate efficient non-viral gene delivery. J Gene Med. 9:275–286.
- Rajagopalan R, Xavier J, Rangaraj N, Rao NM, Gopal V. 2007b. Recombinant fusion proteins TAT‐Mu, Mu and Mu‐Mu mediate efficient non‐viral gene delivery. J Gene Med. 9:275–286.
- Ransohoff RM, Engelhardt B. 2012. The anatomical and cellular basis of immune surveillance in the central nervous system. Nat Rev Immunol. 12:623–635.
- Ransohoff RM, Hafler DA, Lucchinetti CF. 2015. Multiple sclerosis — A quiet revolution. Nat Rev Neurol. 11:134–142.
- Reddy LH, Sharma R, Chuttani K, Mishra A, Murthy R. 2005. Influence of administration route on tumor uptake and biodistribution of etoposide loaded solid lipid nanoparticles in Dalton's lymphoma tumor bearing mice. J Control Release. 105:185–198.
- Reddy MK, Labhasetwar V. 2009. Nanoparticle-mediated delivery of superoxide dismutase to the brain: An effective strategy to reduce ischemia-reperfusion injury. FASEB J. 23:1384–1395.
- Rittchen S, Boyd A, Burns A, Park J, Fahmy TM, Metcalfe S, Williams A. 2015. Myelin repair in vivo is increased by targeting oligodendrocyte precursor cells with nanoparticles encapsulating leukaemia inhibitory factor (LIF). Biomaterials. 56:78–85.
- Rostami E, Kashanian S, Azandaryani AH, Faramarzi H, Dolatabadi JEN, Omidfar K. 2014. Drug targeting using solid lipid nanoparticles. Chem Phys Lipids. 181:56–61.
- Rupp R, Rosenthal SL, Stanberry LR. 2007. VivaGel™(SPL7013 Gel): A candidate dendrimer–microbicide for the prevention of HIV and HSV infection. Int J Nanomedicine. 2:561–566.
- Rutishauser H, Gutknecht M. 1976. Parabolische und hyperbolische partielle Differentialgleichungen. Vorlesungen über Numerische Mathematik. Basel: Springer.
- Sarker DK. 2005. Engineering of nanoemulsions for drug delivery. Curr Drug Deliv. 2:297–310.
- Schäfer R, Ayturan M, Bantleon R, Kehlbach R, Siegel G, Pintaske J, Conrad S, Wolburg H, Northoff H, Wiskirchen J. 2008. The use of clinically approved small particles of iron oxide (SPIO) for labeling of mesenchymal stem cells aggravates clinical symptoms in experimental autoimmune encephalomyelitis and influences their in vivo distribution. Cell Transpl. 17:923–941.
- Schmidt J, Metselaar JM, Wauben MH, Toyka KV, Storm G, Gold R. 2003. Drug targeting by long-circulating liposomal glucocorticosteroids increases therapeutic efficacy in a model of multiple sclerosis. Brain. 126:1895–1904.
- Schubert D, Dargusch R, Raitano J, Chan S-W. 2006. Cerium and yttrium oxide nanoparticles are neuroprotective. Biochem Biophys Res Commun. 342:86–91.
- Sestak JO, Fakhari A, Badawi AH, Siahaan TJ, Berkland C. 2014. Structure, size, and solubility of antigen arrays determines efficacy in experimental autoimmune encephalomyelitis. AAPS J. 16:1185–1193.
- Shen Y, Jin E, Zhang B, Murphy CJ, Sui M, Zhao J, Wang J, Tang J, Fan M, Van Kirk E. 2010. Prodrugs forming high drug loading multifunctional nanocapsules for intracellular cancer drug delivery. J Am Chem Soc. 132:4259–4265.
- Siglienti I, Bendszus M, Kleinschnitz C, Stoll G. 2006. Cytokine profile of iron-laden macrophages: Implications for cellular magnetic resonance imaging. J Neuroimmunol. 173:166–173.
- Silva A, González-Mira E, García M, Egea M, Fonseca J, Silva R, Santos D, Souto E, Ferreira D. 2011. Preparation, characterization and biocompatibility studies on risperidone-loaded solid lipid nanoparticles (SLN): High pressure homogenization versus ultrasound. Colloids Surf B Biointerfaces. 86:158–165.
- Soni S, Babbar AK, Sharma RK, Maitra A. 2006. Delivery of hydrophobised 5-fluorouracil derivative to brain tissue through intravenous route using surface modified nanogels. J Drug Target. 14:87–95.
- Stevens PJ, Sekido M, Lee RJ. 2004. A folate receptor–targeted lipid nanoparticle formulation for a lipophilic paclitaxel prodrug. Pharm Res. 21:2153–2157.
- Stoll G, Bendszus M. 2010. New approaches to neuroimaging of central nervous system inflammation. Curr Opin Neurol. 23:282–286.
- Surtees RA, Rodeck C, Clayton PT. 1992. Aromatic L-amino acid decarboxylase deficiency: Clinical features, diagnosis, and treatment of a new inborn error of neurotransmitter amine synthesis. Neurology. 42:1980–1988.
- Svenson S, Chauhan AS, Reyna LA, Tomalia DA. 2006. Starburst® and Priostar™ dendrimers for controlled drug delivery applications. Polym Preprints. 47:150.
- Taogoshi T, Nomura A, Murakami T, Nagai J, Takano M. 2005. Transport of prostaglandin E1 across the blood‐brain barrier in rats. J Pharm Pharmacol. 57:61–66.
- Teeranachaideekul V, Müller RH, Junyaprasert VB. 2007. Encapsulation of ascorbyl palmitate in nanostructured lipid carriers (NLC) – Effects of formulation parameters on physicochemical stability. Int J Pharm. 340:198–206.
- Tian X-H, Lin X-N, Wei F, Feng W, Huang Z-C, Wang P, Ren L, Diao Y. 2011. Enhanced brain targeting of temozolomide in polysorbate-80 coated polybutylcyanoacrylate nanoparticles. Int J Nanomedicine. 6:445–452.
- Tila D, Ghasemi S, Yazdani-Arazi SN, Ghanbarzadeh S. 2015. Functional liposomes in the cancer-targeted drug delivery. J Biomater Appl. 30:3–16.
- Tiwari S, Tan Y-M, Amiji M. 2006. Preparation and in vitro characterization of multifunctional nanoemulsions for simultaneous MR imaging and targeted drug delivery. J Biomed Nanotechnol. 2:217–224.
- Tomalia DA, Fréchet JM. 2002. Discovery of dendrimers and dendritic polymers: A brief historical perspective. J Polym Sci A Polym Chem 40:2719–2728.
- Vanpouille-Box C, Lacoeuille F, Roux J, Aubé C, Garcion E, Lepareur N, Oberti F, Bouchet F, Noiret N, Garin E. 2011. Lipid nanocapsules loaded with rhenium-188 reduce tumor progression in a rat hepatocellular carcinoma model. PLoS One. 6:e16926.
- Venishetty VK, Samala R, Komuravelli R, Kuncha M, Sistla R, Diwan PV. 2013. β-Hydroxybutyric acid grafted solid lipid nanoparticles: A novel strategy to improve drug delivery to brain. Nanomedicine Nanotechnol Biol Med. 9:388–397.
- Vinogradov S, Batrakova E, Kabanov A. 1999. Poly-(ethylene glycol)–polyethyleneimine NanoGel™ particles: Novel drug delivery systems for antisense oligonucleotides. Colloids Surf B Biointerfaces. 16:291–304.
- Vinogradov SV. 2007. Polymeric nanogel formulations of nucleoside analogs. Expert Opin Drug Deliv. 4:5–17.
- Vinogradov SV, Batrakova EV, Kabanov AV. 2004. Nanogels for oligonucleotide delivery to the brain. Bioconj Chem. 15:50–60.
- Vinogradov SV, Kabanov AV. 2004. Synthesis of Nanogel carriers for delivery of active phosphorylated nucleoside analogues. In: Papers presented at the meeting of American Chemical Society. Division of Polymer Chemistry. NIH Public Access. Polymer Prepr. 228(Pt 2):296.
- Vyas TK, Shahiwala A, Amiji MM. 2008. Improved oral bioavailability and brain transport of Saquinavir upon administration in novel nanoemulsion formulations. Int J Pharm. 347:93–101.
- Wållberg M, Bergquist J, Achour A, Breij E, Harris RA. 2007. Malondialdehyde modification of myelin oligodendrocyte glycoprotein leads to increased immunogenicity and encephalitogenicity. Eur J Immunol. 37:1986–1995.
- Wang C, Wu C, Zhu J, Miller RH, Wang Y. 2011a. Design, synthesis, and evaluation of coumarin-based molecular probes for imaging of myelination. J Med Chem. 54:2331–2340.
- Wang H, Leeuwenburgh SC, Li Y, Jansen JA. 2011b. The use of micro- and nanospheres as functional components for bone tissue regeneration. Tissue Eng B Rev. 18:24–39.
- Wang Y, Su W, Li Q, Li C, Wang H, Li Y, Cao Y, Chang J, Zhang L. 2013. Preparation and evaluation of lidocaine hydrochloride-loaded TAT-conjugated polymeric liposomes for transdermal delivery. Int J Pharm. 441:748–756.
- Warntjes J, Leinhard OD, West J, Lundberg P. 2008. Rapid magnetic resonance quantification on the brain: Optimization for clinical usage. Magn Resonance Med. 60:320–329.
- Weathington NM, van Houwelingen AH, Noerager BD, Jackson PL, Kraneveld AD, Galin FS, Folkerts G, Nijkamp FP, Blalock JE. 2006. A novel peptide CXCR ligand derived from extracellular matrix degradation during airway inflammation. Nat Med. 12:317–323.
- Weinstein JS, Varallyay CG, Dosa E, Gahramanov S, Hamilton B, Rooney WD, Muldoon LL, Neuwelt EA. 2010. Superparamagnetic iron oxide nanoparticles: Diagnostic magnetic resonance imaging and potential therapeutic applications in neurooncology and central nervous system inflammatory pathologies, a review. J Cereb Blood Flow Metab. 30:15–35.
- Weissert R. 2013. The immune pathogenesis of multiple sclerosis. J Neuroimmune Pharmacol. 8:857–866.
- Wickline SA, Lanza GM. 2003. Nanotechnology for molecular imaging and targeted therapy. Circulation. 107:1092–1095.
- Wilczewska AZ, Niemirowicz K, Markiewicz KH, Car H. 2012. Nanoparticles as drug delivery systems. Pharmacol Rep. 64:1020–1037.
- Wohlfart S, Gelperina S, Kreuter J. 2012. Transport of drugs across the blood–brain barrier by nanoparticles. J Control Release. 161:264–273.
- Wohlfart S, Khalansky AS, Gelperina S, Begley D, Kreuter J. 2011. Kinetics of transport of doxorubicin bound to nanoparticles across the blood–brain barrier. J Control Release. 154:103–107.
- Wong HL, Wu XY, Bendayan R. 2012. Nanotechnological advances for the delivery of CNS therapeutics. Adv Drug Deliv Rev. 64:686–700.
- Xie F, Yao N, Qin Y, Zhang Q, Chen H, Yuan M, Tang J, Li X, Fan W, Zhang Q. 2012. Investigation of glucose-modified liposomes using polyethylene glycols with different chain lengths as the linkers for brain targeting. Int J Nanomed. 7:163–175.
- Xie Z-X, Zhang H-L, Wu X-J, Zhu J, Ma D-H, Jin T. 2015. Role of the immunogenic and tolerogenic subsets of dendritic cells in multiple sclerosis. Mediators Inflamm. 2015:513295.
- Xu L, Zhang H, Wu Y. 2013. Dendrimer advances for the central nervous system delivery of therapeutics. ACS Chem Neurosci. 5:2–13.
- Xu Z, Chen L, Gu W, Gao Y, Lin L, Zhang Z, Xi Y, Li Y. 2009. The performance of docetaxel-loaded solid lipid nanoparticles targeted to hepatocellular carcinoma. Biomaterials. 30:226–232.
- Yang Z, Zhang Y, Yang Y, Sun L, Han D, Li H, Wang C. 2010. Pharmacological and toxicological target organelles and safe use of single-walled carbon nanotubes as drug carriers in treating Alzheimer disease. Nanomed Nanotechnol Biol Med. 6:427–441.
- Yazdani Y, Keyhanvar N, Kalhor HR, Rezaei A. 2013. Functional analyses of recombinant mouse hepcidin-1 in cell culture and animal model. Biotechnol Lett. 35:1191–1197.
- Yazdani Y, Sadeghi H, Alimohammadian M, Andalib A, Moazen F, Rezaei A. 2011. Expression of an innate immune element (mouse hepcidin-1) in baculovirus expression system and the comparison of its function with synthetic human hepcidin-25. Iran J Pharm Res. 10:559–568.
- Ye J, Wang Q, Zhou X, Zhang N. 2008. Injectable actarit-loaded solid lipid nanoparticles as passive targeting therapeutic agents for rheumatoid arthritis. Int J Pharm. 352:273–279.
- Yeste A, Nadeau M, Burns EJ, Weiner HL, Quintana FJ. 2012. Nanoparticle-mediated codelivery of myelin antigen and a tolerogenic small molecule suppresses experimental autoimmune encephalomyelitis. Proc Natl Acad Sci USA. 109:11270–11275.
- Youssef T, Fadel M, Fahmy R, Kassab K. 2012. Evaluation of hypericin-loaded solid lipid nanoparticles: Physicochemical properties, photostability and phototoxicity. Pharm Dev Technol. 17:177–186.
- Yuan B, Zhao L, Fu F, Liu Y, Lin C, Wu X, Shen H, Yang Z. 2014. A novel nanoparticle containing MOG peptide with BTLA induces T cell tolerance and prevents multiple sclerosis. Mol Immunol. 57:93–99.
- Yuan H, Wang L-L, Du Y-Z, You J, Hu F-Q, Zeng S. 2007. Preparation and characteristics of nanostructured lipid carriers for control-releasing progesterone by melt-emulsification. Colloids Surf B Biointerfaces. 60:174–179.
- Zhang P, Hu L, Yin Q, Feng L, Li Y. 2012. Transferrin-modified c [RGDfK]-paclitaxel loaded hybrid micelle for sequential blood-brain barrier penetration and glioma targeting therapy. Mol Pharm. 9:1590–1598.
- Zhang Y, Petibone D, Xu Y, Mahmood M, Karmakar A, Casciano D, Ali S, Biris AS. 2014. Toxicity and efficacy of carbon nanotubes and graphene: The utility of carbon-based nanoparticles in nanomedicine. Drug Metab Rev. 46:232–246.
- Zhao X, Zhao H, Chen Z, Lan M. 2014. Ultrasmall superparamagnetic iron oxide nanoparticles for magnetic resonance imaging contrast agent. J Nanosci Nanotechnol. 14:210–220.
- Zhiryakova MV, Izumrudov VA. 2014. Interaction of astramol poly (propyleneimine) dendrimers with DNA and poly (methacrylate) anion in water and water–salt solutions. J Phys Chem B. 118:8819–8826.
- Zhou J, Patel TR, Sirianni RW, Strohbehn G, Zheng M-Q, Duong N, Schafbauer T, Huttner AJ, Huang Y, Carson RE. 2013. Highly penetrative, drug-loaded nanocarriers improve treatment of glioblastoma. Proc Natl Acad Sci USA. 110:11751–11756.
- Zhu S, Xu G. 2010. Single-walled carbon nanohorns and their applications. Nanoscale. 2:2538–2549.