Abstract
Silver, ZnO and CuO nanoparticles (NPs) are increasingly used as biocides. There is however increasing evidence of their threat to “non-target” organisms. In such a context, the understanding of the toxicity mechanisms is crucial for both the design of more efficient nano-antimicrobials, i.e. for “toxic by design” and at the same time for the design of nanomaterials that are biologically and/or environmentally benign throughout their life-cycle (safe by design). This review provides a comprehensive and critical literature overview on Ag, ZnO and CuO NPs’ toxicity mechanisms on the basis of various environmentally relevant test species and mammalian cells in vitro. In addition, factors modifying the toxic effect of nanoparticles, e.g. impact of the test media, are discussed. Literature analysis revealed three major phenomena driving the toxicity of these nanoparticles: (i) dissolution of nanoparticles, (ii) organism-dependent cellular uptake of NPs and (iii) induction of oxidative stress and consequent cellular damages. The emerging information on quantitative structure–activity relationship modeling of nanomaterials’ toxic effects and the challenges of extrapolation of laboratory results to the environment are also addressed.
Introduction
Nanoindustry is one of the fastest growing industries in the history of mankind and has been referred to as the next industrial revolution (Lux-Research, Citation2008). Concerning nanotechnological consumer products, the most rapidly growing field is the use of nanoparticle (NP)-based antimicrobials that are promising alternatives to conventional antibiotics (McDonnell & Russell, Citation1999). According to the Woodrow Wilson Database (http://www.nanotechproject.org), there were more than 1300 nanotechnological consumer products on the market as by March 2011, and 313 of them contained nanosilver. Currently, nanosilver is perhaps the most preferred antimicrobial nanomaterial and Ag NP coatings have been used to inhibit the unwanted growth of bacterial biofilms in medical catheters, prostheses, heart valves, etc. In addition to nanosilver, copper oxide (CuO) and zinc oxide (ZnO) have also been suggested to fight against the undesirable growth of bacteria, fungi and algae (Ivask et al., Citation2012). However, release of biocidal NPs from consumer and household products into the waste streams and further into the environment may pose threat to “non-target” organisms, such as natural microbes and aquatic biota. In this respect, Ag NPs are of especially high concern, because according to the recent scientific literature, aquatic species are extremely sensitive to Ag NPs (Kahru & Dubourguier, Citation2010; Bondarenko et al., Citation2013a).
In addition, also CuO NPs at their realistic environmental concentrations (10 µg/L) can induce adverse biological effects in marine invertebrates (Buffet et al., Citation2011). Due to the fact that all the three NPs in question are used for biocidal applications, these nanomaterials need to be intrinsically toxic. On the other hand, Ag, ZnO and CuO NPs have also other fields of use, e.g. in sensor applications, catalysis, electronics, surfactants, etc (Bondarenko et al., Citation2013a). Only a thorough understanding of mechanisms which drive the toxicity of NPs to a specific organism group may address these two controversial goals: development of more efficient antimicrobials or “safe by design” consumer products.
This review summarizes the current knowledge on toxicity mechanisms of Ag, CuO and ZnO nanoparticles to various aquatic test species and mammalian cells in vitro. This review is a follow-up of our previous “nano” hazard assessment-focused review (Bondarenko et al., Citation2013a) which critically analyzed 317 half-lethal or half-effective concentrations [L(E)C50] and/or minimal inhibitory concentrations (MIC) values of Ag, CuO and ZnO NPs for different organisms: crustaceans, algae, fish, nematodes, bacteria, yeasts, protozoa and mammalian cells in vitro. The most toxic of the three NPs to both environmentally relevant organisms as well as to mammalian cell lines were Ag NPs. For most of the studied species, the L(E)C50 values were below 10 mg/L. The L(E)C50 values of Ag NPs for the above-mentioned organisms/cells spanned nearly four orders of magnitude, from as low as 0.01 mg/L for crustaceans to 38 mg/L for protozoa. The toxicity of nanosilver varied also within the same organism group: 275-fold in case of mammalian cells in vitro and 500-fold in case of bacteria and we suggested that this high variability in nanosilver toxicity was due to different capping agents. The latter is also supported by the fact that the variability in toxicity data for uncoated CuO and ZnO NPs was significantly lower. For most of the studied organisms, the L(E)C50 values of CuO NPs were in the range of 10–100 mg/L and the L(E)C50 values of ZnO NPs were in the range of 1–100 mg/L. In case of ZnO, algae were exceptionally sensitive; toxicity of ZnO particles to algae was about 100-fold higher (EC50 0.1 mg/L) than to most other organisms. An interesting finding of our previous paper (Bondarenko et al., Citation2013a) was: although CuO and ZnO NPs are also recommended to inhibit unwanted growth of bacteria, their toxicity to bacteria was not so remarkable – the median MIC values of CuO and ZnO particles were 200 and 500 mg/L, respectively. One reason for this apparently low sensitivity of bacteria to these metallic NPs can be explained by organic-rich growth media usually used in these tests. Most likely the media masked the actual toxicity of these NPs by complexing the released ions and thus lowering their bioavailable fraction. We ranked the NPs according to their environmental hazard using an approach that adheres to EU-Directive 93/67/EEC (CEC, Citation1996) and is based on the lowest median L(E)C50 value of the three key aquatic test organisms: algae, crustaceans and fish. As a result, Ag NPs and ZnO NPs were classified as “very toxic” and CuO NPs as “toxic” to aquatic organisms. Comparing the effective concentrations of Ag, ZnO and CuO NPs on their target organisms (usually bacteria but also yeasts and algae) to the concentrations at which these NPs affected the selected non-target organisms, revealed another surprising fact that these NPs affect the non-target organisms at overlapping or even at lower concentrations than the target ones. Consequently, if the NPs of Ag, ZnO or CuO will be massively used, their discharge and subsequent leaching to surface waters may pose threat to aquatic species. Toxicity values (Bondarenko et al., Citation2013a) for the selected organism groups/cells are presented in .
Table 1. Toxicity of Ag, ZnO and CuO nanoparticles (NPs) and their respective ions to bacteria, yeast, algae, crustaceans, fish and mammalian cells in vitro.
This review aims to give a comprehensive literature overview of the toxicity mechanisms of Ag, ZnO and CuO NPs. Uptake and solubilization of NPs and the subsequent effects, mainly oxidative damage and connected cellular events, were studied for not only bacteria, yeast, aquatic organisms such as algae, protozoa, daphnids, fish, but also mammalian cells in vitro. In addition, the emerging information on quantitative structure–activity relationship (QSAR) modeling of nanomaterials’ toxic effects and the challenges of extrapolation of laboratory results to the real environment are addressed. The general concept of this review is envisaged in the Supplemental Figure S1.
Bibliometric Data Search on Ag, ZnO and CuO Nanoparticles
To analyze the information available on toxicity mechanisms of Ag, ZnO and CuO NPs, we first analyzed the total amount of papers available in ISI Web of Science (WoS) on Ag, ZnO and CuO NPs for each organism/organism group. We used the following (truncated) search-terms: bacter*, MRSA (methicillin resistant Staphylococcus aureus), Escherichia, yeast*, alga*, protozoa*, ciliate*, daphni*, crustacea*, nematode*, C. elegans, fish*, cell* and in vitro (Supplemental Table SI). This selected set of organisms covers various biological hierarchies: prokaryotic and eukaryotic, unicellular and multicellular organisms, different representatives of the aquatic food-chain (algae, crustaceans, fish) used for the classification and labeling of the environmental hazard of chemicals. Moreover, the suit involves not only particle-ingesting organisms (such as Daphnia sp.) but also organisms that are not ingesting particles (bacteria, yeasts, unicellular algae). Last but not least, this set involves both target and non-target organisms for the biocidal action of Ag, ZnO and CuO NPs (Supplemental Figure S1).
According to the search made on 5 May and 6 May 2013, 31 558 papers were retrieved for nanosilver, 47 714 papers for ZnO NPs and 30 409 papers for CuO NPs (Supplemental Table SI). After assigning the aforementioned search terms for different organisms/organism groups, in total 4662 “biological” papers on nanosilver, 2129 papers on ZnO NPs and 1250 papers on CuO NPs were obtained (Supplemental Table SI). Some of the keywords used concerning the organisms were intentionally redundant as we were also looking for antibacterial effects of NPs toward pathogenic bacteria, i.e. Escherichia and MRSA. Interestingly, more papers were obtained for search term “daphni*” than for “crustacea*.” Thus, in the following bibliometric searches on crustaceans, we used the search term “daphni*.”
For this review, we selected 167 publications related to (eco)toxicology and mechanism of action of Ag NPs, ZnO NPs and CuO NPs. About one-third (31%) of the selected papers originated from the journals classified by us as “ecotoxicology and environmental chemistry journals,” 22% from “toxicological,” 18% from “nanotechnological,” 10% from “chemical” and 8% from “medical” journals (Table 2). Half of the 167 publications originated from 13 journals: Environmental Science and Technology (19 papers), ACS Nano (9), Nanotoxicology (8), Toxicology in Vitro (8), Environmental Toxicology and Chemistry (6), Chemical Research in Toxicology, Chemosphere, Environmental Pollution, Small (all 5 papers), Archives of Toxicology, Langmuir, Nano Letters and Science of the Total Environment (all 4 papers) (Supplemental Table SII).
Table 2. Classification of journals in which toxicity data for Ag, ZnO or CuO NPs and different test organisms or cells were published and that are cited in the current Review.
Organism group-wise distribution of data on biological effects of Ag, ZnO and CuO nanoparticles
The bibliometry (Supplemental Table SI) showed that for protozoa and nematodes the information was too scarce to make a detailed study on toxicity mechanisms. Therefore, in our further search for toxicity mechanisms of NPs a refined set of organism groups was used: bacteria, yeasts, algae, daphnids, fish and mammalian cells in vitro. For these six organism groups, there were altogether 3055 papers on nanosilver, 1623 papers on ZnO NPs and 928 papers on CuO NPs (Supplemental Table SI). The organism-wise analysis of these data showed that the highest percentage of nano-papers concerned bacteria (69, 46 and 52% for Ag, ZnO and CuO NPs, respectively). Among the 2108 papers published on bacteria and Ag NPs, 1501 concerned Escherichia coli and Ag NPs which indicates the medical/hygienic emphasis of this research. A relatively high number of papers were published also on mammalian cell cultures and Ag, ZnO or CuO NPs (19, 33 and 23% of the total, respectively). The information concerning environmentally relevant organisms (such as algae, fish and daphnids) was remarkably lower, ranging from 2% to 8% per organism group (Supplemental Table SI). The latter fact is in agreement with our previous observations which have shown that, in general, the development of ecotoxicology lags severely behind toxicology (Kahru & Ivask, Citation2013) and similarly, there is a 10-year lag between nanotoxicology and nanoecotoxicology (Batley et al., Citation2012; Kahru & Dubourguier, Citation2010).
Organism group-wise distribution of data on toxicity mechanisms of Ag, ZnO and CuO nanoparticles
The above-mentioned six organism groups (Supplemental Table SI, indicated in bold) were further analyzed for the availability of toxicity mechanisms’ information ( and Supplemental Table SIII).
Figure 1. Number and share of papers in ISI WoS on May 5th and 6th, 2013 concerning uptake, oxidative stress and other mechanistic nanotoxicological information (damage to membranes, mitochondria, DNA and lipid peroxidation) on Ag (A), ZnO (B) and CuO NPs (C) for bacteria, yeast, algae, daphnids, fish and mammalian cells in vitro separately and for all these organism groups (TOTAL). Data are plotted from Supplemental Table SIII.
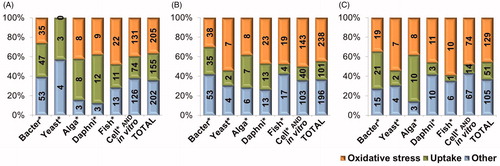
We used the search terms that would reflect the main proposed mechanisms of toxic action of these NPs: oxidative stress, DNA damage, lipid peroxidation, membrane damage and mitochondrial damage (in case of eukaryotic cells). The search term “uptake” was included to characterize the potential for NP internalization (Supplemental Figure S1).
Substantially less papers were published on toxicity mechanisms (Supplemental Table SIII) than on NPs’ biological effects in general (Supplemental Table SI). Interestingly, when in general, 46–69% of the papers describing the toxic effects of Ag, ZnO or CuO NPs concerned bacteria and 19–33% mammalian cell cultures (Supplemental Table SI), then the situation was the opposite when the papers were plotted according to mechanisms of action: only 19–24% of the nanotoxicity mechanisms’ papers concerned bacteria and 53--59% mammalian cell lines (Supplemental Table SIII). The latter was expectable, as mammalian cell cultures have been widely used to study toxicity mechanisms of chemicals and NPs. Remarkably, also the share of toxicity mechanisms’ papers for fish and daphnids was relatively high. Thus, although the total number of papers on NPs and environmentally relevant test organisms was relatively low, many of these were studies, where mechanistic toxicity aspects had been investigated as well.
Main aspects of nano(eco)toxicity mechanisms according to the bibliometry
Among the keywords that were used to search for mechanistic toxicity information for Ag, ZnO and CuO NPs: oxidative stress, DNA damage, lipid peroxidation, membrane damage, mitochondrial damage and uptake, we found that the majority of papers concerned uptake and oxidative stress (18–28% and 36–45%, respectively; Supplemental Table SIII). That was expected as induction of oxidative stress has been considered to be the main paradigm for NPs’ toxicity (Nel et al., Citation2006). Cellular oxidative damage may also cause DNA damage and lipid peroxidation – effects that were elaborated in 11–17% of the mechanistic toxicity papers on nano Ag, ZnO or CuO. Relatively less papers was available on NP induced membrane (6–10%) and mitochondrial (3–5%) damage (Supplemental Table SIII). In order to more thoroughly understand the mechanisms behind the toxicity of Ag, ZnO and CuO NPs, we worked through 33 papers on Ag NPs, 41 papers on ZnO NPs and 44 papers on CuO NPs, and analyzed the recorded/discussed toxicity pathways for different organisms: bacteria, aquatic invertebrates, fish or mammalian cell lines, focusing on nanoparticles’ uptake and oxidative damage. The results are discussed in the next chapters.
Mechanisms Driving the Toxicity of Ag, ZnO and CuO Nanoparticles
As discussed above, bibliometric search on toxicity mechanisms of ZnO, CuO and Ag NPs reveals that particle uptake and oxidative stress were the most studied and discussed effects. On the other hand, all the studied NPs have a metallic component and thus, leaching of metal ions from these particles is a possible scenario. Therefore, in the following chapter, we discuss the factors that affect the physico-chemical behavior: aggregation and dissolution of Ag, ZnO and CuO NPs in the test media and in environmental conditions.
Toxicity of Ag, ZnO and CuO nanoparticles due to dissolution
In general, there is no doubt that the toxicity of Ag, ZnO and CuO NPs is at least partially driven by dissolution. However, the clear differentiation between the roles of dissolved ions and “nano-specific” effects in the observed toxic properties is often difficult to prove experimentally. This is mostly due to methodological difficulties in separation of the dissolved metal ions from nano-sized non-dissolved materials. As discussed in Ivask et al. (Citation2012), the most commonly applied and perhaps the most appropriate techniques for separation of dissolved metal ions from metal nanoparticles are ultracentrifugation and filtration. We will not elaborate here more the separation techniques and focus on results on particle-dependent and dissolved ion-related toxic effects.
Toxicity of ZnO NPs is considered to be clearly dependent on dissolution (Ma et al., Citation2013). That was evident also from the results of our recent review involving a wide range of aquatic organisms and mammalian cells in vitro showing that the median toxicity of ZnO was stunningly similar to that of the Zn-ions, whatever the organism (Bondarenko et al., Citation2013a). The fact that low concentrations (below 1 mg/L) of ZnO NPs almost totally dissolve in aqueous media in the order of hours has been proven (for the review, see Ivask et al., Citation2012) using several analytical methods for quantification of metal-ions: atomic absorption spectroscopy (AAS; Li et al., Citation2011), graphite furnace atomic absorption spectrometry (GFAAS; Peng et al., Citation2011), and inductively coupled plasma atomic emission spectroscopy (ICP-AES; Fairbairn et al., Citation2011; Franklin et al., Citation2007; Rousk et al., Citation2012). Indeed, the toxicity of ZnO NPs and Zn salt has been shown to be comparable in the case of algae Pseudokirchneriella subcapitata (Aruoja et al., Citation2009; Franklin et al., Citation2007), Thalassiosira pseudonana and Chaetoceros gracilis (Peng et al., Citation2011), crustaceans Thamnocephalus platyurus and D. magna (Heinlaan et al., Citation2008; Wiench et al., Citation2009), protozoa T. thermophila (Mortimer et al., Citation2010), bacteria E. coli (Li et al., Citation2011) and yeast S. cerevisiae (Kasemets et al., Citation2009). Dissolution-based toxicity of ZnO has also been shown for mammalian cell lines (Cho et al., Citation2012; Xia et al., Citation2008; Zhang et al., Citation2012). Furthermore, studies where ZnO NPs were doped with increasing concentrations of iron to reduce NP dissolution further confirmed this theory; ZnO NPs with more doped iron were less toxic (Thomas et al., Citation2011).
Many studies have suggested that dissolution is also an important factor which influences the harmful effects of CuO NPs in biological systems (Aruoja et al., Citation2009; Bondarenko et al., Citation2012; Heinlaan et al., Citation2008; Kahru & Dubourguier, Citation2010; Kasemets et al., Citation2013). In our previous studies, we have proposed that solubility-dependent toxicity of CuO NPs is prevalent especially in case of unicellular organisms surrounded by rigid cell wall. This is based on our findings showing that the reactive oxygen species, triggered in bacterial cells after their exposure to CuO NPs, were due to dissolved Cu ions (Bondarenko et al., Citation2012). Additionally, in more complex aquatic organisms like crustaceans D. magna (Blinova et al., Citation2010; Fan et al., Citation2012; Heinlaan et al., Citation2008; Jo et al., Citation2012) the toxic effects of CuO NPs have often been associated with the soluble fraction of NPs as well. Similarly, inhibition of cholinesterase activity in juvenile carp Cyprinus carpio (Zhao et al., Citation2011) and interference with zebrafish embryo hatching (Lin et al., Citation2013) observed after exposure of these organisms to CuO NPs were mostly due to dissolved Cu ions.
It has also been clearly shown that Ag NPs undergo slow oxidation upon contact with water and in the presence of oxygen (i.e. under ambient conditions that are usually used in toxicity assays), NPs are dissolving and releasing ionic silver (Sotiriou et al., Citation2012). For example, Xiu et al. (Citation2012) did not notice any antibacterial effects of Ag NPs in anaerobic conditions where no dissolution was occurring. In aerobic conditions, many studies have found positive correlation between the dissolution rate of Ag NPs and their toxicity to various organisms such as bacteria E. coli (Bondarenko et al., Citation2013b; Gunawan et al., Citation2009; Visnapuu et al., Citation2013), crustaceans D. magna (Hoheisel et al., Citation2012; Jo et al., Citation2012) and T. platyurus (Blinova et al., Citation2013), protozoa Tetrahymena thermophila (Juganson et al., Citation2013), algae Chlamydomonas reinhardtii (Navarro et al., Citation2008) and Raphidocelis subcapitata and fishes Danio rerio (Bar-Ilan et al. Citation2009; Wang et al., Citation2012a), Pimephales promelas (Hoheisel et al., Citation2012) and Oryzias latipes (Kim et al., Citation2011).
On the other hand, although there is a general consensus that dissolution of metal-containing NPs is an important factor driving their toxicity, clear evidence indicates that in certain cases, dissolution does not explain all the toxic effects. For example, a recent toxicogenomic study on toxicity mechanisms of Ag NPs in D. magna demonstrated that Ag NPs had remarkably distinct expression profiles compared to AgNO3 (Poynton et al., Citation2011). Namely, the authors found that major biological processes disrupted by the Ag NPs included protein metabolism and signal transduction, whereas AgNO3 disrupted developmental processes. The differences in the gene expression patterns of Ag NPs and Ag ions were also confirmed with the studies in fish D. rerio and nematode C. elegans (Griffitt et al., Citation2009; Roh et al., Citation2009). In addition, studies with yeast Saccharomyces cerevisiae (Niazi et al., Citation2011) revealed that metallothionein encoding genes (cup1-1 and cup1-2) were strongly induced by Ag NPs (∼45-fold) as well as by Ag-ions (∼22-fold), suggesting that NPs even enhanced Ag-ion stress.
Similarly, CuO NPs have been shown to exhibit other than dissolution-driven toxic effects in mammalian cells (Karlsson et al., Citation2008; Piret et al., Citation2012; Wang et al., Citation2012b; Zhang et al., Citation2012). This is coherent with the findings reported in our previous review (Bondarenko et al., Citation2013a); according to a set of toxicity data collected from the literature, CuO NPs proved more toxic to mammalian cells in vitro than Cu2+ ions. No such effect was observed for Ag and ZnO NPs. As also hypothesized by Limbach et al. (Citation2005), we suggested that once endocytosed, Cu ions leaching from CuO NPs cannot be effectively controlled by the mechanisms that normally regulate the concentration of Cu ions in the cell. Indeed, intracellular copper overload in human cancer cells in vitro was reported when the cells were exposed to lipophilic copper pyrazole–pyridine complex that entered the cells before their dissolution. Therefore, the intracellular toxic concentration of copper was rapidly reached (Tardito et al., Citation2011).
It is also important to note that as the toxicity assays with mammalian cells in vitro use serum, the serum components may disperse and coat NPs (Zook et al., Citation2012) increasing their bioavailability to the cells. For yeast S. cerevisiae, it was shown that if the toxicity tests were performed in protein-rich medium, CuO NPs enhanced the Cu ion-associated stress. Assumingly, the protein-coated NPs sorbed strongly onto the cell surface that was suggested to facilitate the dissolution of CuO in the close vicinity of the yeast cell wall. Interestingly, this effect was prominent in complex organic medium but not in distilled water (Kasemets et al., Citation2013). Evidence that dissolution was not the only factor in the toxicity of metal-containing NPs was also reported for ZnO NPs. For example, although morphologically different ZnO NPs were equally soluble, needle-shaped ZnO NPs inhibited the growth of the diatom Phaeodactylum tricornutum more than spherical NPs (Peng et al., Citation2011). Also, detailed analysis of gene expression profiles and genome-wide mutant library of E. coli (Poynton et al., Citation2011; Reyes et al., Citation2012) showed that the adverse effect of ZnO NPs was not fully explained by solubilization.
In summary, dissolution is an indispensable factor when toxicity of metal-containing NPs is considered. Yet, the issue is relatively complex as also other, nano-specific factors may complement the effect of dissolved metal ions and the distinct role of each factor remains unknown for the moment.
Overall, the previous examples demonstrated that a common toxicity denominator for Ag, CuO and ZnO NPs is the release of metal ions. The complexity of NP dissolution, different factors affecting the dissolution and its importance in nanotoxicity have been recently discussed by Misra et al. (Citation2012). However, to account for the complexity of different environmental compartments, chemical speciation and bioavailability of the released metals should be considered together with NP dissolution. In this respect, the existing models for trace metal speciation, e.g. the free ion activity model (Anderson et al., Citation1978), biotic ligand model (BLM) (Paquin et al., Citation2002), existing models for bioaccumulation, e.g. biodynamic model (Luoma & Rainbow, Citation2005) and chemo- and biodynamic model (Buffle et al., Citation2009) might be useful and their extension to NPs is highly advisable. Similarly to NPs, the interactions (and thus toxicity) of dissolved ions could be affected by different environmental factors. In general, Ca2+ and Mg2+ cations (that cause water hardness) are known to protect biota and could mitigate the toxicity of toxic metal ions by competing with them for the binding sites on biological membranes (Slaveykova & Wilkinson, Citation2005). In addition, the presence of the above-mentioned cations can modify the surface properties and charge of NPs, favoring their aggregation and thus, decreasing their persistence and probability for contact with biota. Moreover, in the close vicinity of living organisms, pH changes may affect, e.g. NPs dissolution, surface charge, aggregation and thus their reactivity (Misra et al., Citation2012).
Copper and silver are known for their strong complexation. Therefore, the toxicity of Ag and Cu compounds is notoriously dependent on the composition of the exposure environment. Indeed, the solubility of Ag NPs and CuO NPs depends on their interactions with organic material in the test environment (proteins, amino acids, natural organic matter, humic substances) that may coat and disperse NPs or complex metal ions. In addition, as silver ions have high affinity toward sulfur, the formation of Ag2S in environmental compartments containing sulfides remarkably decreases the toxicity of Ag NPs (Levard et al., Citation2012). Reduced solubility and toxicity to crustaceans in natural waters has been observed for Ag NPs (Blinova et al. Citation2013; Gao et al., Citation2009) and CuO NPs (Blinova et al., Citation2010). Analogously, the presence of humic acids completely mitigated the toxic effect of Ag NPs on bacterial growth due to the changes in the surface properties of the particles after the sorption of humic acids onto the NPs (Fabrega et al., Citation2009). On the other hand, it has been shown that natural organic matter (NOM) stabilizes NP suspensions and is thus expected to increase the possibility of contact with biota.
The information on ZnO NPs is more contradictory while Blinova et al. (Citation2010) reported no effects of dissolved organic carbon (DOC) of natural waters on the acute toxicity of ZnO NPs to crustaceans; Bian et al. (Citation2011) showed that under certain conditions humic acids can increase the dissolution of 4 nm ZnO NPs. The authors also compared the dissolution of ZnO NPs of different sizes and showed that the smallest NPs dissolved more readily. Recently Li et al. (Citation2013) showed that in synthetic freshwater and natural waters higher pH, and NOM concentrations reduced solubilization of ZnO NPs and thus lowered their toxicity to E. coli. In addition, as the toxic effect of ZnO NPs to E. coli was mediated by Zn-ions, Ca2+ and Mg2+ both dramatically reduced the toxicity of ZnO NPs (Li et al., Citation2013).
Uptake of nanoparticles and effects of nanoparticles on biological membranes
Uptake and the subsequent particle-related toxicity of internalized NPs have been demonstrated mostly for unicellular aquatic eukaryotes as well as for mammalian cells. On the other hand, a few papers have demonstrated NP uptake by unicellular prokaryotes. In general, the rigid cell wall of unicellular organisms does not allow the internalization of NPs and thus, if internalization has been demonstrated, the reason has been increased permeability of cell wall due to previous injury. It is still difficult to predict how big NPs may enter the cells of prokaryotes. Kloepfer et al. (Citation2005) suggested that the particles should be less than 5 nm in diameter. On the other hand, Applerot et al. (Citation2009) and Brayner et al. (Citation2006) showed that ZnO NPs that were below 10 nm in diameter entered bacterial cells and Lok et al. (Citation2007) and Morones et al. (Citation2005) suggested that Ag NPs between 10 and 16 nm may enter the cells of E. coli. Kumar et al. (Citation2011a) studied the internalization and effects of 30 nm ZnO and 50 nm TiO2 NPs in Salmonella typhimurium – a bacterium that is used in the Ames genotoxicity assay. TEM analysis demonstrated the internalization and uniform distribution of NPs inside the bacterial cells. Even at non-bactericidal concentrations a large number of NPs were taken up. Regarding the mechanisms of the uptake, the authors proposed that non-specific diffusion, non-specific membrane damage and specific uptake (silCBA gene transportation system, through porins) are the potential mechanisms through which the NPs could pass the bacterial cell wall and membranes but the precise mechanism is still unknown.
Uptake of NPs by an organism or cell depends on the organism, NPs size but also on its coating. To illustrate the importance of NP coating in NP internalization potency, we present the data of Huang et al. (Citation2008) who studied the bactericidal properties of ZnO NPs to gram positive bacteria Streptococcus agalactiae and Staphylococcus aureus. They showed that the surface modification of ZnO NPs by polyvinyl alcohol increased membrane permeability of bacteria and the cellular internalization of these NPs whereas there was a ZnO NP structure change inside the cells. The internalization was especially evident in the bacteria that were surrounded by NPs. The hydroxyl groups and PVA macromolecules on the surface of coated ZnO NPs were assumed to promote the accumulation of NPs inside the cells as the alkaline compounds dissolve the external part of the cell membrane, which is the major cellular protective barrier (Huang et al., Citation2008). Analogously, Kumar et al. (Citation2011a) who studied the internalization and effects of ZnO and TiO2 NPs in gram-negative bacteria Salmonella typhimurium showed that the addition of S9 (an enzymatic fraction of the liver) promoted the cellular uptake, probably due to the formation of micelles or protein coating on NPs.
As mentioned earlier, in most studies and in case of larger NPs, the intact cell wall of bacteria, yeasts and unicellular algae (Manusadzianas et al., Citation2012) has been shown to provide protection from NPs entrance to cells. Instead, NPs have been shown to interact with bacteria and small eukaryotic organisms through attachment on cellular surface and migration into the membranes (). Interaction of Ag NPs with the cellular membrane of unicellular yeast Candida albicans inhibited the normal budding process of the yeast, probably due to the destruction of membrane integrity (Kim et al., Citation2009a). Similarly, the direct contact between bacterial membranes and Ag NPs has been shown to facilitate the toxicity of Ag NPs to bacteria (Bondarenko et al., Citation2013b). Interaction between NPs and cellular membrane was also important in the case of unicellular eukaryotic organisms such as algae Ochromonas danica and C. reinhardtii. In these cells, Ag NPs that had attached to the cellular surface contributed to the bioavailability of particle-associated Ag ions (Miao et al. Citation2010; Navarro et al., Citation2008).
Figure 2. Uptake and attachment of NPs on cellular membranes. (A) PVP coated Ag NPs interacting with the membrane of bacteria Escherichia coli (TEM image), (B) CuO NPs in the close vicinity of the cell wall of yeast Saccharomyces cerevisiae (dyed with trypan blue, light microscopy image), (C) Ag NPs taken up by protozoa Tetrahymena thermophila into its food vacuoles (light microscopy image), (D) Light microscopy image of Daphnia magna exposed to CuO NPs: Ag NPs inside the gut and interacting with the gut epithelium (cross-section).
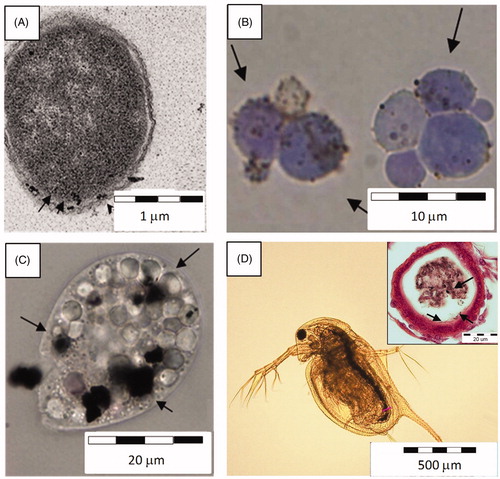
A number of papers have shown that ZnO NPs can attach to the cell wall of microbial cells (Chen et al., Citation2012; Dimkpa et al., Citation2011; Liu et al., Citation2009; Peng et al., Citation2011), and cause aggregation in algae Chlorella sp. Such adhesion of particles may cause mechanical damage; for example, changes in cell morphology and deformation of membranes, disorganization or leakage of intracellular structures (Li et al., Citation2011; Liu et al., Citation2009; Zhu et al., Citation2008), abnormal appearance of mitochondria (Hu et al., Citation2010) and even lack of certain organelles (Peng et al., Citation2011). Supposedly, in the case of unicellular organisms, ZnO NPs increase the permeability of the membranes (Kumar et al., Citation2011b; Xie et al., Citation2011), depolarize cells (Chen et al., Citation2012) and/or perforate the cell walls enabling the NPs to enter the cells (Brayner et al., Citation2006). Additionally, Raman spectrum analysis of ZnO NP-treated E. coli indicated possible destruction of lipids and proteins on cell membrane that might cause the leakage of intracellular contents (Liu et al., Citation2009). However, the latter results are not consistent among the organisms. Several authors have shown that at organism-level ZnO NPs can cause drastic morphological changes in fungi (He et al., Citation2011), attach on nauplii of crustacean Tigriopus japonicas and hamper the movement of D. magna (Poynton et al., Citation2011; Wong et al., Citation2010), damage zebrafish gill cells resulting in shrinkage or loss of cell cytoplasm and abnormal shapes of nuclei (Xiong et al., Citation2011). Reyes et al. (Citation2012) confirmed that in case of bacterial cells, membrane damage and metabolic effects related to energy generation were involved in the toxicity of ZnO NPs. Gogoi et al. (Citation2006) showed that silver NPs (<10 nm) attached to gram-negative E. coli cell wall and resulted in the perforation of the cell wall that lead to the cell death. Direct contact between bacterial cells and Ag NPs facilitated Ag NPs dissolution at cell-NP interface and thus, enhanced the antibacterial effects of nanosilver (Bondarenko et al., Citation2013b).
While in the case of bacterial, algal and yeast cells, the cellular uptake of NPs is not a common scenario, protozoa have been shown to efficiently internalize various NPs (). Endocytosis of Ag NPs into food vacuoles and subsequent excretion of aggregates of these NPs has been demonstrated for protozoan T. thermophila (Juganson et al., Citation2013). Similarly, Mortimer et al. (Citation2011) showed that CuO NPs were readily taken up by protozoa into the food vacuoles. Also in multicellular aquatic organisms that are able to ingest NPs, such as daphnids () and fish, particles per se were found to contribute significantly to the overall toxicity (Poynton et al., Citation2012; Wang et al., Citation2012a). Zhao & Wang (Citation2011) showed that Ag NPs accumulated in the daphnids during a remarkably shorter time period compared to AgNO3. In the same study, it was demonstrated that after the ingestion of Ag NPs, daphnids retained NPs in their gut, whereas bioaccumulation efficiency depended on the surface coating as well as on the size of Ag NPs (Zhao & Wang, Citation2011). Authors postulated that the toxicity of Ag NPs was due to them serving as the sources of intracellular soluble Ag. Indeed, in particle-ingesting organisms, particles may act as Trojan horse-type carriers of metal ions that dissolve intracellularly in various organelles. Interestingly, although CuO NPs were passing the gastrointestinal tract of Daphnia, no NP internalization to gut epithelial cells was seen in TEM-aided visualization (Heinlaan et al., Citation2011). Yet, sub-lethal concentrations of CuO NPs in the gastrointestinal tract of D. magna resulted in extensive bacterial colonization – indication for immunosuppression and/or severe impairment of digestive enzymes by CuO NPs (Heinlaan et al., Citation2011).
Using juvenile carp Cyprinus carpio it was shown that CuO NPs were taken up and accumulated in the fish, mostly in intestine and gills (Zhao et al., Citation2011). Using zebrafish D. rerio embryos as a model, Lee et al. (Citation2007) showed that single Ag NPs, 5–46 nm in diameter, were transported into the embryos through the chorion pore canals and trapped inside the inner mass of the embryos. Authors suggested that accumulation of NPs in embryos was responsible for the developmental abnormalities.
In the case of mammalian cell lines, the uptake of NPs is a very common scenario. From the 10 papers chosen to analyze the toxicity mechanisms of Ag, ZnO and CuO NPs, the uptake of Ag NPs was shown in six and the uptake of CuO and ZnO NPs in three (). It has also been demonstrated that the uptake pathway to mammalian cells depends on coating of NPs and internalization efficiency depended on the size of NPs, being, in general, the highest for 40–50 nm particles (Arvizo et al. Citation2012; Chithrani & Chan, Citation2007). Studies with Ag NPs have shown that Ag NPs applied at equal mass can be even more toxic to mammalian cells in vitro than Ag ions (Kim et al., Citation2009b; Liu et al., Citation2010; Park et al., Citation2011). Remarkably, this intrinsic particle-specific cytotoxicity was mostly evident in case of Ag NPs with the primary size under 20 nm (Kim et al., Citation2009b; Liu et al., Citation2010; Park et al., Citation2011). Most probably, there was a local release of high concentrations of Ag ions from Ag NPs that were taken up by the cells to lysosomes, which eventually lead to toxicity. Cellular localization studies have shown that in addition to endosomes and lysosomes, e.g. CuO NPs localize also in the nucleus and mitochondria of HepG2 and A549 cells (Piret et al., Citation2012; Wang et al., Citation2012b). It has been shown that once in the mammalian body, NPs will be efficiently distributed. In rodents regardless of the exposure route, the primary target organs of Ag NPs were mostly liver, spleen and lungs, i.e. organs that contain high numbers of phagocytosing cells (summarized in Johnston et al., Citation2010).
Figure 3. Summary on adverse effects of Ag, CuO and ZnO nanoparticles in mammalian cell cultures. Ten papers were selected for each nanoparticle; numbers on graphs show the number of articles where the indicated effect was studied and observed as a positive result (data are summarized from Supplemental Tables SIV–SVI).
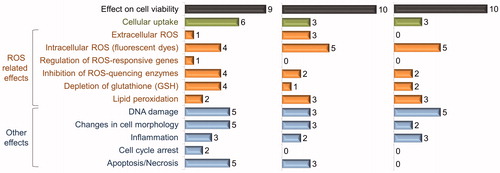
In short, NPs uptake and the resulting NP-specific effects are more prominent in case of multicellular eukaryotic organisms. For unicellular organisms with rigid cell wall the internalization of NPs is rather an exception and mostly reported as a side-effect, accompanying membrane injury that also may be contributed to the coating of NPs. Therefore, due to the differences between the test organisms, one single biotest cannot predict the (eco)toxicological effects of NPs and in order to carry out proper risk assessment, a battery of tests where organisms and cells at different levels of biological organization are used should be applied.
Formation of biocorona phenomenon was already addressed almost 10 years ago (Cedervall et al., Citation2007) and is now a widely accepted model for NPs behavior in an environment that is rich in organic components. Indeed, as NPs are prone to attach to organic matter, the surface coating defines much of their bioactivity. Therefore, the formation of biocorona on the surface of NPs is one of the main factors influencing the final toxicological outcome. For instance, inside the body NPs are constantly surrounded by different biomolecules, mainly proteins, that form a spontaneous coating which evolves in time and enables different biological responses at different times of exposure. Additionally, the presence of biomolecular corona may confer specific interactions between the NP–corona complex and the cell surface including triggering of regulated cell uptake (Lesniak et al., Citation2013). The formation of the corona also depends on the type and coating of NPs. For example, uncoated and surfactant-free silver NPs promoted maximum protein (bovine serum albumin) coating due to increased changes in entropy (Podila et al., Citation2012). Moreover, the biocorona is also formed by enzymatic proteins as has been shown in case of 20 nm citrate coated silver NPs and firefly luciferase as a model enzyme (Käkinen et al., Citation2013).
Oxidative stress and the related effects of nanoparticles
Oxidative damage, caused either by extracellular or by internalized NPs, has been considered one of the main causes of NP toxicity (Nel et al., Citation2006). Reactive oxygen species-induced oxidative stress and the resulting physiological effects have been demonstrated at almost all the levels of biological organization, from bacteria to fish as well as in mammalian cell lines in vitro. Oxygen-derived radicals or reactive oxygen species (ROS) are constantly generated at low levels in aerobic living systems. In addition to normal physiological stimuli, production of ROS may also be triggered by oxidative stress. Under normal conditions, ROS-induced damage is regulated by cellular antioxidant cascade: enzymes superoxide dismutases (SOD), catalases, glutathione peroxidase and non-enzymatic antioxidants such as α-tocopherol, ascorbic acid and glutathione (Blokhina et al., Citation2003). As a result of excessive production of oxygen-based radicals they become prominent toxicological intermediates, and are commonly involved in oxidative stress, defined as an imbalance between the production of ROS and the ability of a biological system to detoxify the reactive intermediates or to repair the resulting damage (Sayre et al., Citation2005).
Various inorganic NPs, including ZnO, CuO and Ag NPs, induced oxidative stress in aquatic microorganisms as summarized in a recent review by von Moos & Slaveykova (Citation2013). The cellular mechanisms underlying ROS generation and oxidative stress induced by NPs in aquatic microorganisms and the methods for the investigation of ROS and oxidative stress were exhaustively discussed (von Moos & Slaveykova, Citation2013). Therefore, the following chapter discusses separately the effects of Ag, ZnO and CuO NPs to environmentally relevant organisms and to mammalian cell lines, keeping the emphasis on oxidative damage.
Oxidative stress-related effects of Ag, ZnO and CuO nanoparticles on environmentally relevant organisms
For assessing the potential of NPs to exert oxidative stress to various environmentally relevant organisms, several biomarkers such as increased activities of SOD, catalase (CAT), glutathione-S-transferase (GST), glutathione peroxidase (GPx) and increased levels of metallothionein-like proteins have been measured (see below). In addition, as lipid molecules make up approximately 30–80% of biological membranes by mass, peroxidation of lipids in response to reactive oxygen species is a very likely scenario (Harrison & Lunt, Citation1980) and also used as a biomarker of oxidative stress.
There are several examples demonstrating that oxidative stress is one of the main drivers of Ag NPs toxic effects. Yet, the original cause of oxidative stress either by nanoparticulate Ag or dissolved Ag ions often remains unexplained. Ivask et al. (Citation2010) studied the toxic effects of Ag NPs to recombinant E. coli mutants deficient in different SOD and showed that mutant strains (especially the triple mutant sodABC) were more sensitive to Ag NPs than the wild type; however, analogous effects were observed for Ag ions. Induction of ROS by Ag NPs has also been shown in zebrafish D. rerio (Choi & Hu, Citation2008). Interestingly, while several studies suggest that ROS may be one of the mechanisms via which Ag NPs influence biological systems, this mechanism of toxicity was mostly apparent in the cases of less toxic Ag NPs releasing less Ag ions (Sotiriou & Pratsinis, Citation2010; Yang et al., Citation2012).
Based on the current knowledge, CuO NPs are prone to exert oxidative damage even more than Ag NPs. Similarly to Ag NPs, the extent of contribution of nanoparticulate CuO and the dissolved Cu ions to the production of reactive oxygen species is still debatable. It is well known that ions of redox-active metals, including copper, may yield free radicals via the Fenton-type reaction and inflict intracellular oxidative stress (Valko et al., Citation2005). The cupric ion [Cu (II)] can be reduced to cuprous ion [Cu (I)] in the presence of biological reducing agents such as ascorbic acid or glutathione (GSH) and consequently, reactive hydroxyl radicals are generated from hydrogen peroxide via Fenton reaction.
Induction of reactive oxygen species by CuO NPs has been demonstrated at various levels of biological organization. Using luminescent bacterial tests, we have shown ROS-generating potential of aqueous suspensions of CuO NPs in recombinant E. coli strains (Ivask et al., Citation2010). Recently, we showed that CuO NPs induced oxidative stress and DNA damage defense systems in recombinant E. coli strains already at very low subtoxic concentrations (0.1 mg Cu/L) (Bondarenko et al., Citation2012). However, these effects were most likely triggered by dissolved Cu ions. It has also been shown that oxidative damage is induced by extracellular, membrane surface-attached CuO NPs. Applerot et al. (Citation2012) demonstrated that upon attachment to bacteria, CuO NPs induced lipid peroxidation and intracellular oxidative stress. Effects of CuO NPs on membrane lipids have been also described for unicellular protozoa T. thermophila by Mortimer et al. (Citation2011): in response to exposure to CuO NPs (80 mg/L), protozoa adjusted its membrane fatty acid composition to more rigid by decreasing the amount of unsaturated fatty acids (C18:3 cis-6, 9, 12 and C18:2 cis-9, 12) and increasing the amount of saturated fatty acids (C18:0 and C16:0).
Lipid peroxidation caused by CuO NPs has also been demonstrated in tissue and gills of rainbow trout (Shaw et al., Citation2012), bacteria E. coli and Bacillus subtilis (Applerot et al., Citation2012) and green algae C. reinhardtii (Cheloni & Slaveykova, Citation2013).
ZnO is a photocatalyst that can promote ROS generation at or above its band gap energy 3.37 eV that corresponds to 368 nm light (Ma et al., Citation2013). Indeed, Yu et al. (Citation2011) and Xiong et al. (Citation2011) showed that under illumination, at concentrations above 10 mg/L, suspensions of ZnO NPs induced hydroxyl radicals (•OH). At the same time, no hydroxyl radical production was detected in case of bulk ZnO particles. A study with bacteria Pseudomonas chlororaphis revealed that ZnO NPs may induce intracellular ROS even irrespective of illumination (Dimkpa et al., Citation2011). ROS defense systems have been demonstrated to participate in ZnO toxicity response of bacteria. E. coli sodAB- double and sodABC− triple mutants were more sensitive to ZnO NPs and bulk ZnO than the wild-type E. coli bacterium (Ivask et al., Citation2010). Lipid peroxidation due to ZnO NPs’-induced reactive oxygen radicals has been observed in several organisms ranging from bacteria (Kumar et al., Citation2011b) and earthworms (Hu et al., Citation2010) to fish (Hao & Chen, Citation2012; Xiong et al., Citation2011). The increase in lipid peroxidation levels due to exposure to ZnO particles might indicate that the self-scavenging capacity of antioxidant defense systems was exceeded (Hao & Chen, Citation2012). Yet, studies using the most common oxidative stress biomarkers have not reported a common mechanism of action of ZnO NPs as the patterns are different among species and even in different organs of the same individual. For instance, SOD activity showed a decreasing tendency in zebrafish liver but was augmented in zebrafish gut tissue (Xiong et al., Citation2011) and in gill, liver, brain and intestine of carp (Hao & Chen, Citation2012). However, in nano ZnO-exposed marine crustaceans (copepods) Tigriopus japonicus, there was no change in SOD expression (Wong et al., Citation2010) but instead, a 4-fold increase in general stress protein hsp70 was observed. Also, Ivask et al. (Citation2010) did not observe induction of superoxide anion sensing recombinant bacteria during the exposure to zinc compounds, suggesting that most probably other ROS than superoxide anion () were formed in response to ZnO NPs. However, the current knowledge on the extent to which oxidative damage is responsible for ZnO NPs mechanism of action is still questionable.
Oxidative stress related and other effects of Ag, CuO and ZnO nanoparticles on mammalian cells in vitro
In this paragraph, we discuss the toxicity mechanisms of NPs to mammalian cells in vitro separately from environmentally relevant organisms, mainly because according to our bibliometric analysis ( and Supplemental Table SIII), more than 50% of papers describing one or more mechanistic toxicity aspects of Ag, ZnO or CuO NPs concerned mammalian cells. The highlights for molecular toxicity mechanisms obtained by analysis of the literature concerning the above-mentioned NPs and mammalian cell lines are summarized in Supplemental Tables SIV–SVI.
There is a plethora of mammalian cell lines available for toxicity testing and as the cell lines originate from different tissues it is reasonable to assume that they may differ in their response to NPs. In general, the cellular systems have been selected according to the portals of entry of NPs and the respective target cells in the body. The human body has several biological interfaces for direct substance exchange with the environment, i.e. the skin, respiratory tract and gastrointestinal tract which are thus also major portals of entry for NPs. When NPs were administered intravenously to rats, the levels were the highest in the liver, followed in decreasing order by the levels in the spleen, lung and kidney (Fabian et al., Citation2008). Due to that, most of the in vitro toxicity assays with mammalian cells have been performed with cellular models of the respiratory system, e.g. human lung adenocarcinomic cell line A549 (Bachand et al., Citation2012; Foldbjerg et al., Citation2011; Karlsson et al., Citation2008; Kaur & Tikoo, Citation2013; Liu et al., Citation2010) and murine macrophage cell line RAW264.7 (Bachand et al., Citation2012; Kaur & Tikoo, Citation2013; Park et al., Citation2010, Citation2011); as well as liver models such as rat liver cell line BRL 3A (Hussain et al., Citation2005) and human hepatocellular carcinoma cell line HepG2 (Kim et al., Citation2009b; Liu et al., Citation2010; Nowrouzi et al., Citation2010). In our previous review (Bondarenko et al., Citation2013a), we showed that nearly 30% of the EC50 values published on Ag, CuO and ZnO NPs (altogether 75 values) originated from A549 cells in vitro, although nearly 20 different cell lines were used in total.
As already emphasized above, the majority of the toxicity studies on mammalian cell lines are conducted to understand the toxicity mechanisms (Supplemental Figure S1 and Table SIII).
Similarly to environmental test organisms, the majority of the effects of NPs, reported for mammalian cell lines, can be linked to reactive oxygen species. In 4 out of 10 papers describing the cellular toxicity of Ag NPs and in 5 of 10 papers describing the cellular toxicity of ZnO and CuO NPs, induction of intracellular ROS was recorded (). Yet, to date, it is unclear whether ROS induced by NPs is the direct cause for cytotoxicity or a secondary effect of cellular disturbance (Park et al., Citation2011; Shvedova et al., Citation2010). Also, intracellular ROS levels did not correlate with the extracellularly generated ROS in a cell free system (Park et al., Citation2011; Shi et al., Citation2012; Xia et al., Citation2008), suggesting that intracellular ROS is not the effect of particles per se but is rather a result of interaction of NPs with the cellular environment. Especially high levels of ROS were induced in various mammalian cells by Ag NPs of less than 20 nm in diameter (Foldbjerg et al., Citation2011; Kim et al., Citation2009a; Liu et al., Citation2010), thus the potential of NPs to induce ROS may be connected to their surface area. Interestingly, Ag NPs induced the up-regulation of ROS-responsive genes at m-RNA level (Kim et al., Citation2009a), whereas no increase was observed at protein activity level (Arora et al., Citation2008). Furthermore, several authors reported the inhibition of the activity of SOD – the main cellular enzymatic scavenger of superoxide radicals – by Ag NPs (Liu et al., Citation2010; Nowrouzi et al., Citation2010). This indicates that cytotoxicity of Ag NPs may arise not only from induction of ROS but also from the inhibition of the activity of ROS scavenging proteins. Indeed, for murine macrophage cell line RAW264.7, Park et al. (Citation2011) suggested that the generation of ROS was a secondary effect of Ag NPs rather than the primary cause of cytotoxicity. Induction of ROS in mammalian cells has been demonstrated also for CuO NPs. For example, addition of antioxidant N-acetylcysteine (NAC) mitigated the cytotoxicity of CuO NPs to human larynx epidermoid carcinoma cell line HEp-2 cells (Cho et al., Citation2012; Fahmy & Cormier, Citation2009).
Several articles claim that in mammalian cell cultures, ZnO NPs have particle-specific toxicity on mitochondrial function by increasing the mitochondrial inner membrane permeability and impairing the respiratory chain, thus leading to energy dissipation, oxidative stress and even apoptosis as shown using isolated rat liver mitochondria by Li et al. (Citation2012). Sharma et al. (Citation2012) using human liver cells in vitro also showed that ZnO NPs caused DNA damage and cell death whereas the mode of cell death was apoptosis mediated by the ROS triggered mitochondrial pathway.
They also provided the detailed molecular mechanism of ZnO-induced mitochondrial apoptosis pathway in HepG2 cells and concluded that ZnO NPs per se, rather than the released ions, were toxic and that the generation of ROS and ROS-triggered apoptosis was attributed to the semiconductor and nanolevel characteristics of ZnO NPs. Also, Kim et al. (Citation2010) exposed primary cultured rat alveolar epithelial cell monolayers to ZnO NPs and observed injury of the cells in dose- and time-dependent manner caused at least partially by free Zn ions released from ZnO NPs, mitochondrial dysfunction and increased intracellular ROS.
It has been proposed that intracellular ROS induced by NPs inflict DNA damage. By studying time-dependent accumulation of ROS and activation of DNA damage-responsive proteins in A549 cells Wang et al. (Citation2012b) suggested that intracellular CuO NPs first generated ROS, which subsequently induced the expression of p38 and p53 proteins and lead to irreversible DNA damage. Thus, the DNA damage was a secondary effect of elevated levels of ROS as was also suggested by Cronholm et al. (Citation2011) and Park et al. (Citation2011). In addition to DNA damage, elevated levels of intracellular ROS can induce production of inflammation promoting cytokines (Park et al., Citation2010, Citation2011). Among a number of inflammatory markers measured by Park et al. (Citation2011) from RAW 264.7 macrophages after exposure to Ag NPs, the secretion of G-CSF (granulocyte colony-stimulating factor-1) was the most prominent. Another study using A549 cells exposed in vitro to occupationally equivalent concentrations of Ag NPs showed that exposure to AgNPs in occupational settings will probably not cause acute oxidative stress and/or cytotoxicity in alveolar epithelial cells but can elicit an inflammatory response. Interestingly, the secretion of IL-8 (interleukin 8) in A549 cells was induced by 20 nm and not by 60 nm Ag NPs (Bachand et al., Citation2012).
Norzila et al. (Citation2000) and Piret et al. (Citation2012) studied the involvement of 96 genes in the immunity and pro-inflammatory response to CuO NPs in HepG2 cells and showed overexpression of transcripts, coding for chemokines and proteins, able to recruit and activate immune cells. Remarkably, the overproduction of IL-8 was abolished by free radical scavenger (NAC) treatment, showing the direct role of ROS on inflammation (Piret et al., Citation2012). Similarly, the expression of several inflammatory markers was observed in A549 cells exposed to CuO NPs, but also to the supernatant of CuO NPs at a slightly decreased level (Cho et al., Citation2012). In the same study, CuO but not its supernatant recruited eosinophils, showing CuO particle-specific ability to induce inflammation (Cho et al., Citation2012). As a result of chronic inflammation and elevated levels of ROS, cell death by either apoptosis or necrosis may occur. Indeed, cytotoxicity induced by high Ag NPs concentrations seems to be realized through necrosis. Using HT-1080 and A431 cell lines as models and caspase-3 activity as an endpoint, Arora et al. (Citation2008) suggested that the apoptotic cell death prevailed at relatively low concentrations of Ag NPs, whereas at higher cytotoxic doses, necrosis occurred. There were also other studies suggesting the minor role of apoptotic pathway in toxicity of Ag NPs to mammalian cells in vitro (Foldbjerg et al., Citation2011; Liu et al., Citation2010).
Modeling of Toxicity of Metal-Containing Nanoparticles
As discussed above, there are numerous studies investigating various mechanisms of biological action of NPs. Yet, no clear toxicity pathways for NPs have been proposed. Toxicity modeling is one tool that could help to clarify the specific mode of action of NPs to a specific organism or cell. These models usually referred to as quantitative structure–activity relationships (QSARs), enable the prediction of activity or properties of chemicals based on their physico-chemical or structural parameters. QSARs are widely used in drug development in order to simplify the design process of leads, or, in the toxicity prediction of chemicals. Also, the EU chemicals regulation REACH accepts in silico methods, including QSARs, as an alternative to toxicity testing under certain conditions (Burello & Worth, Citation2011a,Citationb). As the development and production of new NPs accelerates, the traditional in vivo and in vitro approaches in safety assessment will inevitably lag behind also in this field. In addition, as the experimental evaluation of the safety of chemicals is expensive and time-consuming, computational methods have been found to be efficient alternatives for predicting the potential toxicity and environmental impact of new nanomaterials before mass production. As an example, Puzyn et al. (Citation2011) showed that the QSAR method commonly used to predict the physico-chemical properties of chemical compounds can be applied to predict the toxicity of various metal oxides. Thus, QSAR methods, or more appropriately quantitative nanostructure–activity relationships (QNARs) are expected to fill data gaps and guide safe design of nanomaterials.
Requirements for QNAR models and specific features of nanoparticles
To build a QNAR model two kinds of data are needed – those that characterize the physico-chemical and/or structural parameters (nanoparticle descriptors) of the “chemical” entity and those that describe the biological effects or activity. While there are elaborate methods in place to calculate different descriptors for chemicals, derivation of adequate descriptors for nanomaterials is remarkably more complicated. First, NPs cannot be characterized as a defined entity but are rather a group that is separated from chemicals in a somewhat arbitrary way. NPs are complex assemblies of inorganic and/or organic compounds, sometimes coated or functionalized with diverse organic molecules where the exact stoichiometry may vary from one particle to another. Even if the composition of a NP is well known, its three-dimensional structure may be highly complex (Fourches et al., Citation2011). In addition to variable composition and specific three-dimensional structure, calculation of molecular descriptors is hindered because NPs are usually too large for standard computational approaches, e.g. quantum mechanical calculations that are used for molecules. Gajewicz et al. (Citation2012) demonstrated that calculation of the band gap energy for NPs was meaningful for particles less than 5 nm in diameter whereas the particles between 15 and 90 nm rather behaved like bulk materials and their size effect could thus be neglected. Therefore, for nanomaterials new type of descriptors involving perhaps a number of simplifications but being able to describe the unusual structural properties, have to be developed.
The data about biological effects of the chemical entities are as important as descriptors for a QSAR model. In theory, the biological effect data used for model development should be dependent on the intended use of the studied chemicals in their lifecycle (exposure of humans or the environment). A useful QNAR model should be based on a number of chemical entities and their biological effects.
Small scale high throughput (HTP) test systems with high number of different cell types and endpoints have been suggested as the way to go in nanotoxicological hazard assessment in the future (Damoiseaux et al., Citation2011). For example, for nanomaterials (George et al., Citation2011), a HTP automated multiparametric assay with zebrafish embryos the results of which are comparable to in vivo responses have been developed. Shaw et al. (Citation2008) have applied several cell lines and endpoints to assess the effects of an iron oxide NP library.
In addition to quantity, the quality of toxicological data used for QSAR modeling is of vital importance. As suggested by Gajewicz et al. (Citation2012), the modelers need to critically evaluate the biological effects data and even go to experimental details. However, this may be complicated due to the lack of details in experimental results or due to the insufficient experience from the modeler’s side. It should be emphasized that interpretation of data in the case of NPs may be demanding even for an experienced toxicologist. Therefore, care should be taken in interpreting the toxicological results recorded for NPs in different test systems.
Data collection for QNARs
Despite the vast number of scientific publications concerning toxicity of NPs (more than 5000 papers was available for toxicological aspects Ag, ZnO and CuO NPs; Supplemental Table SI), not many of the published data can be used for building a QSAR model. In most cases, this is due to variability between the test protocols and results from different laboratories; consequently, the data for a QSAR model should preferably be obtained in the same laboratory and using a similar test. As suggested by Gajewicz et al. (Citation2012), only datasets with full characterization of the experimental protocol and the nanomaterial used (shape, SEM/TEM photo, concentration, purity, size, etc.) should be considered as good and reliable information. According to our experience, the most common issue with the toxicological data, especially those published before 2010 is the lack of accurate characterization of NPs used in the experiments. To illustrate the differences between different test system, we bring an example from Brunner et al. (Citation2006) who showed that for human mesothelioma MSTO-211H cells in vitro the toxicity of seven metal oxide NPs and asbestos decreased in the order Fe2O3≈ asbestos > ZnO > CeO2 ≈ ZrO2 ≈ TiO2 ≈ Ca3(PO4)2 whereas for rodent 3T3 fibroblast cells the order was ZnO > asbestos ≈ ZrO2 > Ca3(PO4)2 ≈ Fe2O3 ≈ CeO ≈ TiO2. As the obtained toxicity patterns for these two cell lines were very different, these data cannot be possibly included in one model. Even if the nanomaterial(s) used are adequately described and the test protocols are standardized, usually the number of different particles tested is very low. Unfortunately, most of the published studies only provide data for one specific particle, which may not even be representative of this class of NPs (e.g. a rod-shaped Ag particle cannot be used to predict the toxicity of a spherical Ag particle or a rod-shaped carbonaceous structure).
Currently, there are just a few studies that can be used as a basis for QSAR development. A large data set of 51 differently coated (dextran and other polymers, e.g. ethylene diamine, protamine, carboxylic acid, basic peptides) or non-coated iron-oxide (Fe3O4 or Fe2O3) particles and CdSe core-ZnS shell quantum dots was studied by Shaw et al. (Citation2008). The authors characterized the effects of these NPs to two different cell lines in vitro using four different endpoints. Based on the results, also an initial hierarchical classification of NPs was performed. Another large toxicological dataset was published by Weissleder et al. (Citation2005) who studied the cellular effects of 109 super-paramagnetic iron oxide NPs using four different cell lines. Recently, Zhang et al. (Citation2012) published in vitro and in vivo toxicological data for 24 metal oxide NPs. For in vitro assays, the authors used two different cell lines, human bronchial epithelial cells (BEAS-2B) and rat alveolar macrophage cells (RAW) and three toxicity endpoints. In vivo studies were conducted in parallel using mouse oropharyngeal aspiration. Also, smaller sets of data have been published; for example, Liu et al. (Citation2011) published a dataset consisting of nine metal oxides and their effects on viability of bronchial epithelial cells; Hu et al. (Citation2009) analyzed the effects of seven metal oxide NPs to bacteria Escherichia coli. This dataset was later expanded and used for QSAR development by Puzyn et al. (Citation2011).
Currently developed nano-QSAR/QNAR models
There are only a few reports on the development of QSARs for NPs. The studies carried out this far suggest it is impossible to develop a universal model for all the NPs. Therefore, distinct models must be constructed for different applicability domains (NPs with different shapes, compositions etc.) and for different biological endpoints, as described below. So far, the existing data clearly show that inert structures of carbon nanotubes exhibit their biological effects mainly via surface treatment while the toxicity of other kinds of NPs may be also driven by material properties, dissolution, etc.
Most of the models developed so far predict the general toxic effects of NPs. Fourches et al. (Citation2010) and Epa et al. (Citation2012) used a dataset of Shaw et al. (Citation2008) to model the toxicity (effects on ATP content, mitochondrial membrane potential and apoptosis) of a set of 51 metallic NPs (iron-oxide particles and CdSe core-ZnS shell quantum dots) to mammalian cells in vitro. Liu et al. (Citation2011) used atomization energy, period of the metal from which NP was composed of, NPs primary size and volume fraction, to predict the NPs effects on viability of human transformed bronchial epithelial cells (BEAS-2B).
In addition to models that predict the general cytotoxicity of NPs, certain models have been developed to predict the uptake and potential mechanisms of toxicity – dissolution of NPs and NPs potential to induce reactive oxygen radicals. Chau & Yap (Citation2012) used a dataset from Weissleder et al. (Citation2005) on 109 fluorescent superparamagnetic iron oxide NPs to model the uptake of iron oxide NPs by human pancreatic cancer (PaCa2) cells. The model showed that the uptake of these NPs was mainly (R2 = 0.8) determined by hydrogen bonding capacity, acidity lipophilicity/hydrophilicity and van der Waals forces of the NPs. It is interesting to note that when the authors modeled the biological effects of this set of NPs to different human cell lines, they found almost no overlap between the molecular descriptors that predicted the uptake by human umbilical vein endothelial (HUVEC) cells and PaCa2 cells. Thus, the effects of NPs may be very cell-specific even within one organism which shows again that indeed, no universal model can be developed. Puzyn et al. (Citation2011) showed that, for Escherichia coli, the toxicity of metal oxide NPs was mainly dependent on the enthalpy of formation of a gaseous cation (ΔHMe+) from these NPs, i.e. the chemical instability of metal oxides leading to dissolution. Thus, this model links dissolution of metal oxide NPs to their effects to bacteria. On the other hand, in mammalian cells, the toxicity of metal oxide NPs was mostly driven by oxidative stress inducing potential of the NPs. Zhang et al. (Citation2012) modeled mammalian cytotoxicity of 24 metal oxide NPs based on NPs bandgap energy, i.e. the potential of the NP to accept electrons from biological molecules (Burello & Worth Citation2011b).
Interestingly, in all the developed models size was not included as an important parameter. As discussed by Burello & Worth (Citation2011b) and Puzyn et al. (Citation2011), if NPs are bigger than 15–20 nm in diameter, their size is not an important variable determining their biological effects. Yet, the effect of smaller sizes on molecular descriptors still needs to be elucidated. Also, as suggested by various authors, grouping of NPs to subgroups is necessary to develop a conceptual framework for nano QSARs. Finally, to improve the already developed nano-QSAR models, it is vital that both the quality of experimental test data as well as the generation of nano-specific descriptors is improved.
Conclusions and outlook
Studies of the toxicity mechanisms are crucial for understanding the impact of NPs on the living organisms. The information on mechanisms is needed for the design of more efficient nano-antimicrobials (toxic by design) and equally for the design of NPs that are biologically and/or environmentally benign throughout their life-cycle (safe by design).
Our literature search showed that although still scarce, the information on biological effects of three antimicrobial NPs – Ag, CuO and ZnO NPs and their physico-chemical drivers is accumulating. The key properties driving the toxicity of the three NPs are: intrinsic toxicity of the metal and the solubility of NPs dictated by the chemical properties of the metal, uptake and the potential to induce oxidative stress. While most of the studies are performed in well-standardized and controlled test environment where the physico-chemical behavior of NPs can be predicted, in the real exposure environment the intrinsic parameters of NPs are usually modified due to complex nature of the test environment itself. This issue has been recognized by many researchers and increasingly more testing is performed not only according to OECD or ISO procedures which are crucial to follow the classification and labeling procedures but also in environmentally more relevant conditions. Accumulation of good quality data on nanoparticles biological effects and their drivers combined with the knowledge on physico-chemical behavior of NPs in environmental conditions establishes a future basis for adequate methods to predict the potential impact of NPs in biological and/or environmental systems.
Declaration of interest
The authors report no conflicts of interest and are responsible for the content and writing of the article.
Supplementary Materials
Download PDF (338 KB)Acknowledgements
MM, VS and AK thank the Scientific Exchange Program NMS-CH. This research was supported by the Estonian target funding project SF0690063s08, ETF8561, ETF9001, ETF9347, EU 7th Framework Program under grant agreement no. 263147 (NanoValid) and No. 309314 (MODERN). The study was supported by the EU Regional Development Foundation, Environmental Conservation and Environmental Technology R&D Program project TERIKVANT (3.2.0802.11-0043). VS acknowledges the support of the Swiss National Research Program 64 on the Opportunities and Risk of Nanomaterials.
References
- Anderson MA, Morel FMM, Guillard RRL. 1978. Growth limitation of a coastal diatom by low zinc ion activity. Nature 276:70–1
- Applerot G, Lellouche J, Lipovsky A, Nitzan Y, Lubart R, Gedanken A, Banin E. 2012. Understanding the antibacterial mechanism of CuO nanoparticles: Revealing the route of induced oxidative stress. Small 8:3326–37
- Applerot G, Lipovsky A, Dror R, Perkas N, Nitzan Y, Lubart R, Gedanken A. 2009. Enhanced antibacterial activity of nanocrystalline ZnO due to increased ROS-mediated cell injury. Adv Funct Mater 19:842–52
- Arora S, Jain J, Rajwade JM, Paknikar KM. 2008. Cellular responses induced by silver nanoparticles: in vitro studies. Toxicol Lett 179:93–100
- Aruoja V, Dubourguier H-C, Kasemets K, Kahru A. 2009. Toxicity of nanoparticles of CuO, ZnO and TiO2 to microalgae Pseudokirchneriella subcapitata. Sci Total Environ 407:1461–8
- Arvizo RR, Bhattacharyya S, Kudgus RA, Giri K, Bhattacharya R, Mukherjee P. 2012. Intrinsic therapeutic applications of noble metal nanoparticles: Past, present and future. Chem Soc Rev 41:2943–70
- Bachand GD, Allen A, Bachand M, Achyuthan KE, Seagrave JC, Brozik SM. 2012. Cytotoxicity and inflammation in human alveolar epithelial cells following exposure to occupational levels of gold and silver nanoparticles. J Nanopart Res 14:1212
- Bar-Ilan O, Albrecht RM, Fako VE, Furgeson DY. 2009. Toxicity assessments of multisized gold and silver nanoparticles in zebrafish embryos. Small 5:1897–910
- Batley GE, Kirby JK, McLaughlin MJ. 2012. Fate and risks of nanomaterials in aquatic and terrestrial environments. Acc Chem Res 46:854–62
- Bian S-W, Mudunkotuwa IA, Rupasinghe T, Grassian VH. 2011. Aggregation and dissolution of 4 nm ZnO nanoparticles in aqueous environments: influence of pH, ionic strength, size, and adsorption of humic acid. Langmuir 27:6059–68
- Blinova I, Ivask A, Heinlaan M, Mortimer M, Kahru A. 2010. Ecotoxicity of nanoparticles of CuO and ZnO in natural water. Environ Pollut 158:41–7
- Blinova I, Niskanen J, Kajankari P, Kanarbik L, Kakinen A, Tenhu H, et al. 2013. Toxicity of two types of silver nanoparticles to aquatic crustaceans Daphnia magna and Thamnocephalus platyurus. Environ Sci Pollut R 20:3456–63
- Blokhina O, Virolainen E, Fagerstedt KV. 2003. Antioxidants, oxidative damage and oxygen deprivation stress: a review. Ann Bot London 91:179–94
- Bondarenko O, Ivask A, Kakinen A, Kahru A. 2012. Sub-toxic effects of CuO nanoparticles on bacteria: kinetics, role of Cu ions and possible mechanisms of action. Environ Pollut 169:81–9
- Bondarenko O, Juganson K, Ivask A, Kasemets K, Mortimer M, Kahru A. 2013a. Toxicity of Ag, CuO and ZnO nanoparticles to selected environmentally relevant test organisms and mammalian cells in vitro: a critical review. Arch Toxicol 84:1181–200
- Bondarenko O, Ivask A, Käkinen A, Kurvet I, Kahru A. 2013b. Particle-cell contact enhances antibacterial activity of silver nanoparticles. PLoS ONE 8:e64060
- Brayner R, Ferrari-Iliou R, Brivois N, Djediat S, Benedetti MF, Fiévet F. 2006. Toxicological impact studies based on Escherichia coli bacteria in ultrafine ZnO nanoparticles colloidal medium. Nano Lett 6:866–70
- Brunner TJ, Wick P, Manser P, Spohn P, Grass RN, Limbach LK, Bruinink A, Stark WJ. 2006. In vitro cytotoxicity of oxide nanoparticles: Comparison to asbestos, silica, and the effect of particle solubility. Environ Sci Technol 40:4374–81
- Buffet P-E, Tankoua OF, Pan J-F, Berhanu D, Herrenknecht C, Poirier L, et al. 2011. Behavioural and biochemical responses of two marine invertebrates Scrobicularia plana and Hediste diversicolor to copper oxide nanoparticles. Chemosphere 84:166–74
- Buffle J, Wilkinson KJ, van Leeuwen HP. 2009. Chemodynamics and bioavailability in natural waters. Environ Sci Technol 43:7170–4
- Burello E, Worth A. 2011a. Computational nanotoxicology: Predicting toxicity of nanoparticles. Nat Nanotechnol 6:138–9
- Burello E, Worth AP. 2011b. QSAR modeling of nanomaterials. WIREs Nanomed Nanobiotechnol 3:298–306
- CEC. 1996. Technical Guidance Documents in support of the Commission Directive 93/67/EEC on Risk Assessment for New Notified Substances and the Commission Regulation (EC) 1488/94 on Risk Assessment for Existing Substances. Commission of the European Communities/European Chemicals Bureau, Ispra
- Cedervall T, Lynch I, Lindman S, Berggård T, Thulin E, Nilsson H, et al. 2007. Understanding the nanoparticle–protein corona using methods to quantify exchange rates and affinities of proteins for nanoparticles. Proc Natl Acad Sci USA 104:2050–5
- Chau YT, Yap CW. 2012. Quantitative nanostructure-activity relationship modelling of nanoparticles. RSC Adv 2:8489–96
- Cheloni G, Slaveykova VI. 2013. Optimization of the C11-BODIPY581/591 dye for the determination of lipid oxidation in Chlamydomonas reinhardtii by flow cytometry. Cytometry A. doi: 10.1002/cyto.a.22338
- Chen P, Powell BA, Mortimer M, Ke PC. 2012. Adaptive interactions between zinc oxide nanoparticles and Chlorella sp. Environ Sci Technol 46:12178–85
- Chithrani BD, Chan WCW. 2007. Elucidating the mechanism of cellular uptake and removal of protein-coated gold nanoparticles of different sizes and shapes. Nano Lett 7:1542–50
- Cho W-S, Duffin R, Poland CA, Duschl A, Oostingh GJ, MacNee W, et al. 2012. Differential pro-inflammatory effects of metal oxide nanoparticles and their soluble ions in vitro and in vivo; zinc and copper nanoparticles, but not their ions, recruit eosinophils to the lungs. Nanotoxicology 6:22–35
- Choi O, Hu Z. 2008. Size dependent and reactive oxygen species related nanosilver toxicity to nitrifying bacteria. Environ Sci Technol 42:4583–8
- Cronholm P, Midander K, Karlsson HL, Elihn K, Wallinder IO, Moller L. 2011. Effect of sonication and serum proteins on copper release from copper nanoparticles and the toxicity towards lung epithelial cells. Nanotoxicology 5:269–81
- Damoiseaux R, George S, Li M, Pokhrel S, Ji Z, France B, et al. 2011. No time to lose-high throughput screening to assess nanomaterial safety. Nanoscale 3:1345–60
- Dimkpa CO, Calder A, Britt DW, McLean JE, Anderson AJ. 2011. Responses of a soil bacterium, Pseudomonas chlororaphis O6 to commercial metal oxide nanoparticles compared with responses to metal ions. Environ Pollut 159:1749–56
- Epa VC, Burden FR, Tassa C, Weissleder R, Shaw S, Winkler DA. 2012. Modeling biological activities of nanoparticles. Nano Lett 12:5808–12
- Fabian E, Landsiedel R, Ma-Hock L, Wiench K, Wohlleben W, Ravenzwaay B. 2008. Tissue distribution and toxicity of intravenously administered titanium dioxide nanoparticles in rats. Arch Toxicol 82:151–7
- Fabrega J, Fawcett SR, Renshaw JC, Lead JR. 2009. Silver nanoparticle impact on bacterial growth: effect of pH, concentration, and organic matter. Environ Sci Technol 43:7285–90
- Fahmy B, Cormier SA. 2009. Copper oxide nanoparticles induce oxidative stress and cytotoxicity in airway epithelial cells. Toxicol In Vitro 23:1365–71
- Fairbairn EA, Keller AA, Maedler L, Zhou D, Pokhrel S, Cherr GN. 2011. Metal oxide nanomaterials in seawater: linking physicochemical characteristics with biological response in sea urchin development. J Hazard Mater 192:1565–71
- Fan WH, Shi ZW, Yang XP, Cui MM, Wang XL, Zhang DF, et al. 2012. Bioaccumulation and biomarker responses of cubic and octahedral Cu2O micro/nanocrystals in Daphnia magna. Water Res 46:5981–8
- Foldbjerg R, Dang DA, Autrup H. 2011. Cytotoxicity and genotoxicity of silver nanoparticles in the human lung cancer cell line, A549. Arch Toxicol 85:743–50
- Fourches D, Pu D, Tassa C, Weissleder R, Shaw SY, Mumper RJ, Tropsha A. 2010. Quantitative nanostructure−activity relationship modeling. ACS Nano 4:5703–12
- Fourches D, Pu D, Tropsha A. 2011. Exploring quantitative nanostructure-activity relationships (QNAR) modeling as a tool for predicting biological effects of manufactured nanoparticles. Comb Chem High T Scr 14:217–225
- Franklin NM, Rogers NJ, Apte SC, Batley GE, Gadd GE, Casey PS. 2007. Comparative toxicity of nanoparticulate ZnO, bulk ZnO, and ZnCl2 to a freshwater microalga (Pseudokirchneriella subcapitata): the importance of particle solubility. Environ Sci Technol 41:8484–90
- Gajewicz A, Rasulev B, Dinadayalane TC, Urbaszek P, Puzyn T, Leszczynska D, Leszczynski J. 2012. Advancing risk assessment of engineered nanomaterials: application of computational approaches. Adv Drug Deliver Rev 64:1663–93
- Gao J, Youn S, Hovsepyan A, Llaneza VnL, Wang Y, Bitton G, Bonzongo J-CJ. 2009. Dispersion and toxicity of selected manufactured nanomaterials in natural river water samples: effects of water chemical composition. Environ Sci Technol 43:3322–8
- George S, Xia T, Rallo R, Zhao Y, Ji Z, Lin S, et al. 2011. Use of a high-throughput screening approach coupled with in vivo zebrafish embryo screening to develop hazard ranking for engineered nanomaterials. ACS Nano 5:1805–17
- Gogoi SK, Gopinath P, Paul A, Ramesh A, Ghosh SS, Chattopadhyay A. 2006. green fluorescent protein-expressing Escherichia coli as a model system for investigating the antimicrobial activities of silver nanoparticles. Langmuir 22:9322–8
- Griffitt RJ, Hyndman K, Denslow ND, Barber DS. 2009. Comparison of molecular and histological changes in zebrafish gills exposed to metallic nanoparticles. Toxicol Sci 107:404–15
- Gunawan C, Teoh WY, Marquis CP, Lifia J, Amal R. 2009. Reversible antimicrobial photoswitching in nanosilver. Small 5:341–4
- Hao L, Chen L. 2012. Oxidative stress responses in different organs of carp (Cyprinus carpio) with exposure to ZnO nanoparticles. Ecotox Environ Safe 80:103–10
- Harrison RG, Lunt G. 1980. Membrane Components in Biological Membranes: Their Structure and Function, 2nd ed. Glasgow, London: Blackie, Vol. 62, 101
- He L, Liu Y, Mustapha A, Lin M. 2011. Antifungal activity of zinc oxide nanoparticles against Botrytis cinerea and Penicillium expansum. Microbiol Res 166:207–15
- Heinlaan M, Ivask A, Blinova I, Dubourguier H-C, Kahru A. 2008. Toxicity of nanosized and bulk ZnO, CuO and TiO2 to bacteria Vibrio fischeri and crustaceans Daphnia magna and Thamnocephalus platyurus. Chemosphere 71:1308–16
- Heinlaan M, Kahru A, Kasemets K, Arbeille B, Prensier G, Dubourguier H-C. 2011. Changes in the Daphnia magna midgut upon ingestion of copper oxide nanoparticles: a transmission electron microscopy study. Water Res 45:179–90
- Hoheisel SM, Diamond S, Mount D. 2012. Comparison of nanosilver and ionic silver toxicity in Daphnia magna and Pimephales promelas. Environ Toxicol Chem 31:2557–63
- Hu CW, Li M, Cui YB, Li DS, Chen J, Yang LY. 2010. Toxicological effects of TiO2 and ZnO nanoparticles in soil on earthworm Eisenia fetida. Soil Biol Biochem 42:586–91
- Hu X, Cook S, Wang P, Hwang H-m. 2009. In vitro evaluation of cytotoxicity of engineered metal oxide nanoparticles. Sci Total Environ 407:3070–2
- Huang Z, Zheng X, Yan D, Yin G, Liao X, Kang Y, et al. 2008. Toxicological effect of ZnO nanoparticles based on bacteria. Langmuir 24:4140–4
- Hussain SM, Hess KL, Gearhart JM, Geiss KT, Schlager JJ. 2005. In vitro toxicity of nanoparticles in BRL 3A rat liver cells. Toxicol In Vitro 19:975–83
- Ivask A, Bondarenko O, Jepihhina N, Kahru A. 2010. Profiling of the reactive oxygen species-related ecotoxicity of CuO, ZnO, TiO2, silver and fullerene nanoparticles using a set of recombinant luminescent Escherichia coli strains: differentiating the impact of particles and solubilised metals. Anal Bioanal Chem 398:701–16
- Ivask A, George S, Bondarenko O, Kahru A. 2012. Metal-containing nano-antimicrobials: differentiating the impact of solubilized metals and particles. In: Cioffi A & Rai M, eds. Nano-Antimicrobials: Progress and Prospects. New York: Springer, 253--90
- Jo HJ, Choi JW, Lee SH, Hong SW. 2012. Acute toxicity of Ag and CuO nanoparticle suspensions against Daphnia magna: the importance of their dissolved fraction varying with preparation methods. J Hazard Mater 227:301–8
- Johnston HJ, Hutchison G, Christensen FM, Peters S, Hankin S, Stone V. 2010. A review of the in vivo and in vitro toxicity of silver and gold particulates: particle attributes and biological mechanisms responsible for the observed toxicity. Crit Rev Toxicol 40:328–46
- Juganson K, Mortimer M, Ivask A, Kasemets K, Kahru A. 2013. Extracellular conversion of silver ions into silver nanoparticles by protozoan Tetrahymena thermophila. Environ Sci Process Impacts 15:244–50
- Kahru A, Dubourguier H-C. 2010. From ecotoxicology to nanoecotoxicology. Toxicology 269:105–19
- Kahru A, Ivask A. 2013. Mapping the dawn of nanoecotoxicological research. Acc Chem Res 46:823–33
- Käkinen A, Ding F, Chen P, Mortimer M, Kahru A, Ke PC. 2013. Interaction of firefly luciferase and silver nanoparticles and its impact on enzyme activity. Nanotechnology 24:345101
- Karlsson HL, Cronholm P, Gustafsson J, Moeller L. 2008. Copper oxide nanoparticles are highly toxic: a comparison between metal oxide nanoparticles and carbon nanotubes. Chem Res Toxicol 21:1726–32
- Kasemets K, Ivask A, Dubourguier H-C, Kahru A. 2009. Toxicity of nanoparticles of ZnO, CuO and TiO2 to yeast Saccharomyces cerevisiae. Toxicol In Vitro 23:1116–22
- Kasemets K, Suppi S, Kunnis-Beres K, Kahru A. 2013. Toxicity of CuO nanoparticles to yeast Saccharomyces cerevisiae BY4741 wild-type and its nine isogenic single-gene deletion mutants. Chem Res Toxicol 26:356–67
- Kaur J, Tikoo K. 2013. Evaluating cell specific cytotoxicity of differentially charged silver nanoparticles. Food Chem Toxicol 51:1–14
- Kim J, Kim S, Lee S. 2011. Differentiation of the toxicities of silver nanoparticles and silver ions to the Japanese medaka (Oryzias latipes) and the cladoceran Daphnia magna. Nanotoxicology 5:208–14
- Kim K-J, Sung W, Suh B, Moon S-K, Choi J-S, Kim J, Lee D. 2009a. Antifungal activity and mode of action of silver nano-particles on Candida albicans. BioMetals 22:235–42
- Kim S, Choi JE, Choi J, Chung K-H, Park K, Yi J, Ryu D-Y. 2009b. Oxidative stress-dependent toxicity of silver nanoparticles in human hepatoma cells. Toxicol In Vitro 23:1076–84
- Kim YH, Fazlollahi F, Kennedy IM, Yacobi NR, Hamm-Alvarez SF, Borok Z, et al. 2010. Alveolar epithelial cell injury due to zinc oxide nanoparticle exposure. Am J Resp Crit Care 182:1398–409
- Kloepfer JA, Mielke RE, Nadeau JL. 2005. Uptake of CdSe and CdSe/ZnS quantum dots into bacteria via purine-dependent mechanisms. Appl Environ Microb 71:2548–57
- Kumar A, Pandey AK, Singh SS, Shanker R, Dhawan A. 2011a. Cellular uptake and mutagenic potential of metal oxide nanoparticles in bacterial cells. Chemosphere 83:1124–32
- Kumar A, Pandey AK, Singh SS, Shanker R, Dhawan A. 2011b. Engineered ZnO and TiO2 nanoparticles induce oxidative stress and DNA damage leading to reduced viability of Escherichia coli. Free Radical Bio Med 51:1872–81
- Lee KJ, Nallathamby PD, Browning LM, Osgood CJ, Xu X-HN. 2007. In vivo imaging of transport and biocompatibility of single silver nanoparticles in early development of zebrafish embryos. ACS Nano 1:133–43
- Lesniak A, Salvati A, Santos-Martinez MJ, Radomski MW, Dawson KA, Åberg C. 2013. Nanoparticle adhesion to the cell membrane and its effect on nanoparticle uptake efficiency. J Am Chem Soc 135:1438–44
- Levard C, Hotze EM, Lowry GV, Brown GE, Jr. 2012. Environmental transformations of silver nanoparticles: impact on stability and toxicity. Environ Sci Technol 46:6900–14
- Li J-H, Liu X-R, Zhang Y, Tian F-F, Zhao G-Y, Yu Q-L-Y, Jiang F-L, Liu Y. 2012. Toxicity of nano zinc oxide to mitochondria. Toxicol Res 1:137–44
- Li M, Lin D, Zhu L. 2013. Effects of water chemistry on the dissolution of ZnO nanoparticles and their toxicity to Escherichia coli. Environ Pollut 173:97–102
- Li M, Zhu L, Lin D. 2011. Toxicity of ZnO nanoparticles to Escherichia coli: mechanism and the influence of medium components. Environ Sci Technol 45:1977–83
- Limbach LK, Li Y, Grass RN, Brunner TJ, Hintermann MA, Muller M, et al. 2005. Oxide nanoparticle uptake in human lung fibroblasts: effects of particle size, agglomeration, and diffusion at low concentrations. Environ Sci Technol 39:9370–6
- Lin S, Zhao Y, Ji Z, Ear J, Chang CH, Zhang H, et al. 2013. Zebrafish high-throughput screening to study the impact of dissolvable metal oxide nanoparticles on the hatching enzyme, ZHE1. Small 9:1776–85
- Liu R, Rallo R, George S, Ji Z, Nair S, Nel AE, Cohen Y. 2011. Classification nanoSAR development for cytotoxicity of metal oxide nanoparticles. Small 7:1118–26
- Liu W, Wu Y, Wang C, Li HC, Wang T, Liao CY, et al. 2010. Impact of silver nanoparticles on human cells: effect of particle size. Nanotoxicology 4:319–30
- Liu Y, He L, Mustapha A, Li H, Hu ZQ, Lin M. 2009. Antibacterial activities of zinc oxide nanoparticles against Escherichia coli O157:H7. J Appl Microbiol 107:1193–201
- Lok C-N, Ho C-M, Chen R, He Q-Y, Yu W-Y, Sun H, et al. 2007. Silver nanoparticles: partial oxidation and antibacterial activities. J Biol Inorg Chem 12:527–34
- Luoma SN, Rainbow PS. 2005. Why is metal bioaccumulation so variable? Biodynamics as a unifying concept. Environ Sci Technol 39:1921–31
- Lux-Research. 2008. Nanomaterials state of the market Q3 2008: Stealth success, broad impact. Report. [Online] https://portal.luxresearchinc.com/research/document_excerpt/3735 [Last accessed 30 October 2013]
- Ma H, Williams PL, Diamond SA. 2013. Ecotoxicity of manufactured ZnO nanoparticles: A review. Environ Pollut 172:76–85
- Manusadzianas L, Caillet C, Fachetti L, Gylyte B, Grigutyte R, Jurkoniene S, et al. 2012. Toxicity of copper oxide nanoparticle suspensions to aquatic biota. Environ Toxicol Chem 31:108–14
- McDonnell G, Russell AD. 1999. Antiseptics and disinfectants: activity, action, and resistance. Clin Microbiol Rev 12:147–79
- Miao A-J, Luo Z, Chen C-S, Chin W-C, Santschi PH, Quigg A. 2010. Intracellular uptake: a possible mechanism for silver engineered nanoparticle toxicity to a freshwater alga Ochromonas danica. PLoS ONE 5:e15196
- Misra SK, Dybowska A, Berhanu D, Luoma SN, Valsami-Jones E. 2012. The complexity of nanoparticle dissolution and its importance in nanotoxicological studies. Sci Total Environ 438:225–32
- Morones JR, Elechiguerra JL, Camacho A, Holt K, Kouri JB, Ramírez JT, Yacaman MJ. 2005. The bactericidal effect of silver nanoparticles. Nanotechnology 16:2346--53
- Mortimer M, Kasemets K, Kahru A. 2010. Toxicity of ZnO and CuO nanoparticles to ciliated protozoa Tetrahymena thermophila. Toxicology 269:182–9
- Mortimer M, Kasemets K, Vodovnik M, Marinsek-Logar R, Kahru A. 2011. Exposure to CuO nanoparticles changes the fatty acid composition of protozoa Tetrahymena thermophila. Environ Sci Technol 45:6617–24
- Navarro E, Piccapietra F, Wagner B, Marconi F, Kaegi R, Odzak N, et al. 2008. Toxicity of silver nanoparticles to Chlamydomonas reinhardtii. Environ Sci Technol 42:8959–64
- Nel A, Xia T, Madler L, Li N. 2006. Toxic potential of materials at the nanolevel. Science 311:622–7
- Niazi JH, Sang B-I, Kim YS, Gu MB. 2011. Global gene response in Saccharomyces cerevisiae exposed to silver nanoparticles. Appl Biochem Biotech 164:1278–91
- Norzila MZ, Fakes K, Henry RL, Simpson J, Gibson PG. 2000. Interleukin-8 secretion and neutrophil recruitment accompanies induced sputum eosinophil activation in children with acute asthma. Am J Resp Crit Care 161:769–74
- Nowrouzi A, Meghrazi K, Golmohammadi T, Golestani A, Ahmadian S, Shafiezadeh M, et al. 2010. Cytotoxicity of subtoxic AgNP in human hepatoma cell line (HepG2) after long-term exposure. Iran Biomed J 14:23–32
- Paquin PR, Gorsuch JW, Apte S, Batley GE, Bowles KC, Campbell PGC, et al. 2002. The biotic ligand model: a historical overview. Comp Biochem Phys C 133:3–35
- Park E-J, Yi J, Kim Y, Choi K, Park K. 2010. Silver nanoparticles induce cytotoxicity by a Trojan-horse type mechanism. Toxicol In Vitro 24:872–8
- Park MVDZ, Neigh AM, Vermeulen JP, de la Fonteyne LJJ, Verharen HW, Briede JJ, et al. 2011. The effect of particle size on the cytotoxicity, inflammation, developmental toxicity and genotoxicity of silver nanoparticles. Biomaterials 32:9810–7
- Peng X, Palma S, Fisher NS, Wong SS. 2011. Effect of morphology of ZnO nanostructures on their toxicity to marine algae. Aquat Toxicol 102:186–96
- Piret JP, Jacques D, Audinot JN, Mejia J, Boilan E, Noel F, et al. 2012. Copper(II) oxide nanoparticles penetrate into HepG2 cells, exert cytotoxicity via oxidative stress and induce pro-inflammatory response. Nanoscale 4:7168–84
- Podila R, Chen R, Ke PC, Rao AM. 2012. Effects of surface functional groups on the formation of nanoparticle-protein corona. Appl Phys Lett 101:263701
- Poynton HC, Lazorchak JM, Impellitteri CA, Blalock BJ, Rogers K, Allen HJ, et al. 2012. Toxicogenomic responses of nanotoxicity in Daphnia magna exposed to silver nitrate and coated silver nanoparticles. Environ Sci Technol 46:6288–96
- Poynton HC, Lazorchak JM, Impellitteri CA, Smith ME, Rogers K, Patra M, et al. 2011. Differential gene expression in Daphnia magna suggests distinct modes of action and bioavailability for ZnO nanoparticles and Zn ions. Environ Sci Technol 45:762–8
- Puzyn T, Rasulev B, Gajewicz A, Hu X, Dasari TP, Michalkova A, et al. 2011. Using nano-QSAR to predict the cytotoxicity of metal oxide nanoparticles. Nat Nanotechnol 6:175–8
- Reyes VC, Li M, Hoek EMV, Mahendra S, Damoiseaux R. 2012. Genome-wide assessment in Escherichia coli reveals time-dependent nanotoxicity paradigms. ACS Nano 6:9402–15
- Roh J-Y, Sim SJ, Yi J, Park K, Chung KH, Ryu D-Y, Choi J. 2009. Ecotoxicity of silver nanoparticles on the soil nematode Caenorhabditis elegans using functional ecotoxicogenomics. Environ Sci Technol 43:3933–40
- Rousk J, Ackermann K, Curling SF, Jones DL. 2012. Comparative toxicity of nanoparticulate CuO and ZnO to soil bacterial communities. PLoS ONE 7:e34197
- Sayre L, Moreira P, Smith M, Perry G. 2005. Metal ions and oxidative protein modification in neurological disease. Ann Ist Super Sanita 41:143–64
- Sharma V, Anderson D, Dhawan A. 2012. Zinc oxide nanoparticles induce oxidative DNA damage and ROS-triggered mitochondria mediated apoptosis in human liver cells (HepG2). Apoptosis 17:852–70
- Shaw BJ, Al-Bairuty G, Handy RD. 2012. Effects of waterborne copper nanoparticles and copper sulphate on rainbow trout, (Oncorhynchus mykiss): physiology and accumulation. Aquat Toxicol 116:90–101
- Shaw SY, Westly EC, Pittet MJ, Subramanian A, Schreiber SL, Weissleder R. 2008. Perturbational profiling of nanomaterial biologic activity. Proc Natl Acad Sci USA 105:7387–92
- Shi JP, Ma CY, Xu B, Zhang HW, Yu CP. 2012. Effect of light on toxicity of nanosilver to Tetrahymena pyriformis. Environ Toxicol Chem 31:1630–8
- Shvedova AA, Kagan VE, Fadeel B. 2010. Close encounters of the small kind: Adverse effects of man-made materials interfacing with the nano-cosmos of biological systems. Annu Rev Pharmacol 50:63–88
- Slaveykova VI, Wilkinson KJ. 2005. Predicting the bioavailability of metals and metal complexes: critical review of the biotic ligand model. Environ Chem 2:9–24
- Sotiriou GA, Meyer A, Knijnenburg JTN, Panke S, Pratsinis SE. 2012. Quantifying the origin of released Ag+ ions from nanosilver. Langmuir 28:15929–36
- Sotiriou GA, Pratsinis SE. 2010. Antibacterial activity of nanosilver ions and particles. Environ Sci Technol 44:5649–54
- Tardito S, Bassanetti I, Bignardi C, Elviri L, Tegoni M, Mucchino C, et al. 2011. Copper binding agents acting as copper ionophores lead to caspase inhibition and paraptotic cell death in human cancer cells. J Am Chem Soc 133:6235–42
- Thomas CR, George S, Horst AM, Ji Z, Miller RJ, Peralta-Videa JR, et al. 2011. Nanomaterials in the environment: from materials to high-throughput screening to organisms. ACS Nano 5:13–20
- Valko M, Morris H, Cronin MTD. 2005. Metals, toxicity and oxidative stress. Curr Med Chem 12:1161–208
- Visnapuu M, Joost U, Juganson K, Künnis-Beres K, Kahru A, Kisand V, Ivask A. 2013. Dissolution of silver nanowires and nanospheres dictates their toxicity to Escherichia coli. BioMed Res Int 2013:819252
- von Moos N, Slaveykova VI. 2013. Oxidative stress induced by inorganic nanoparticles in bacteria and aquatic microalgae – state of the art and knowledge gaps. Nanotoxicology. DOI: 10.3109/17435390.2013.809810
- Wang Z, Chen J, Li X, Shao J, Peijnenburg WJGM. 2012a. Aquatic toxicity of nanosilver colloids to different trophic organisms: contributions of particles and free silver ion. Environ Toxicol Chem 31:2408–13
- Wang ZY, Li N, Zhao J, White JC, Qu P, Xing BS. 2012b. CuO nanoparticle interaction with human epithelial cells: Cellular uptake, location, export, and genotoxicity. Chem Res Toxicol 25:1512–21
- Weissleder R, Kelly K, Sun EY, Shtatland T, Josephson L. 2005. Cell-specific targeting of nanoparticles by multivalent attachment of small molecules. Nat Biotechnol 23:1418–23
- Wiench K, Wohlleben W, Hisgen V, Radke K, Salinas E, Zok S, Landsiedel R. 2009. Acute and chronic effects of nano- and non-nano-scale TiO2 and ZnO particles on mobility and reproduction of the freshwater invertebrate Daphnia magna. Chemosphere 76:1356–65
- Wong SWY, Leung PTY, Djurisic AB, Leung KMY. 2010. Toxicities of nano zinc oxide to five marine organisms: influences of aggregate size and ion solubility. Anal Bioanal Chem 396:609–18
- Xia T, Kovochich M, Liong M, Maedler L, Gilbert B, Shi H, et al. 2008. Comparison of the mechanism of toxicity of zinc oxide and cerium oxide nanoparticles based on dissolution and oxidative stress properties. ACS Nano 2:2121–34
- Xie Y, He Y, Irwin PL, Jin T, Shi X. 2011. Antibacterial activity and mechanism of action of zinc oxide nanoparticles against Campylobacter jejuni. Appl Environ Microb 77:2325–31
- Xiong D, Fang T, Yu L, Sima X, Zhu W. 2011. Effects of nano-scale TiO2, ZnO and their bulk counterparts on zebrafish: acute toxicity, oxidative stress and oxidative damage. Sci Total Environ 409:1444–52
- Xiu Z-M, Zhang Q-B, Puppala HL, Colvin VL, Alvarez PJJ. 2012. Negligible particle-specific antibacterial activity of silver nanoparticles. Nano Lett 12:4271–5
- Yang X, Gondikas AP, Marinakos SM, Auffan M, Liu J, Hsu-Kim H, Meyer JN. 2012. Mechanism of silver nanoparticle toxicity is dependent on dissolved silver and surface coating in Caenorhabditis elegans. Environ Sci Technol 46:1119–27
- Yu L-P, Fang T, Xiong D-W, Zhu W-T, Sima X-F. 2011. Comparative toxicity of nano-ZnO and bulk ZnO suspensions to zebrafish and the effects of sedimentation, (OH)-O-center dot production and particle dissolution in distilled water. J Environ Monitor 13:1975–82
- Zhang H, Ji Z, Xia T, Meng H, Low-Kam C, Liu R, et al. 2012. Use of metal oxide nanoparticle band gap to develop a predictive paradigm for oxidative stress and acute pulmonary inflammation. ACS Nano 6:4349–68
- Zhao C-M, Wang W-X. 2011. Comparison of acute and chronic toxicity of silver nanoparticles and silver nitrate to Daphnia magna. Environ Toxicol Chem 30:885–92
- Zhao J, Wang Z, Liu X, Xie X, Zhang K, Xing B. 2011. Distribution of CuO nanoparticles in juvenile carp (Cyprinus carpio) and their potential toxicity. J Hazard Mater 197:304–10
- Zhu X, Zhu L, Duan Z, Qi R, Li Y, Lang Y. 2008. Comparative toxicity of several metal oxide nanoparticle aqueous suspensions to Zebrafish (Danio rerio) early developmental stage. J Environ Sci Heal A 43:278–84
- Zook J, Halter M, Cleveland D, Long S. 2012. Disentangling the effects of polymer coatings on silver nanoparticle agglomeration, dissolution, and toxicity to determine mechanisms of nanotoxicity. J Nanopart Res 14:1–9