Abstract
Hemoglobin (Hb) derivatization for blood substitute purposes often involves multi-step processes including redox reagents such as borohydride and periodate, with possible subsequent side effects. Disuccinimidyl suberate (DSS) allows protein cross-linking without toxic side-products, forming one-step peptide bonds with the lysine residues. Here, we report that Hb polymers were obtained using DSS, making this the first report of a single-step polymerization for blood substitutes. The increase in autooxidation rate incurred by this polymerization is completely reversed when BSA is copolymerized with Hb. Copolymerization of Hb with BSA appears to be beneficial for alleviating pro-oxidant effects, regardless of the polymerizing agent employed.
A safe and effective blood substitute is expected to carry and deliver oxygen to tissues without the need for matching blood types before use and with increased stability for long-time storage as well as with decreased ricks of bacterial or viral contamination (Alayash Citation2004, Chen et al. Citation2009). Most of the proposed blood substitutes are hemoglobin-based oxygen carriers (HBOCs); however, none of them are currently accepted for general clinical use. Side effects noted for HBOCs have been linked to extravasation (due to low apparent molecular volumes), increased oxygen affinity, and reactivity toward oxidative and nitrosative stress agents. Alleviation of these side effects has been sought via chemical and/or genetic manipulation of hemoglobin (Hb), to increase its apparent volume via cross-linking and/or modulate its reactivity toward stress agents (Alayash Citation2004, Winslow Citation2004, Doherty et al. Citation1998, Reeder et al. Citation2008, Portoro et al. Citation2008, Chang Citation2009, Citation2004). We have shown that some of these strategies inherently incur increases in the autooxidation rate at the globin iron (Deac et al. Citation2009, Citation2011, Citation2010). This autooxidation tendency may to some extent be expected to be inevitably linked to the rigidity brought about by chemical cross-linking; however, a second potential source of undesirable reactivity are the redox processes involved as distinctive steps in some of the derivatization procedures. Thus, glutaraldehyde (GL) cross-linking requires a subsequent reaction with the strong redox reagent borohydride; other strategies involve the use of a known oxidizer, periodate (Deac et al. Citation2011, Harris and Palmer Citation2008). We have recently shown that Hb may be derivatized with polyethyleneglycol (PEG) on the lysine residues employing a single non-redox step that yields a stable peptide bond, via reaction with a commercially available activated PEG derivative featuring an N-hydroxysuccinimide ester group and thus avoiding any redox reactivity during cross-linking (Zolog et al. Citation2011). On a different note, we have also shown that whereas cross-linking of Hb with periodate-oxidized adenosine triphosphate (oATP) may incur increased autooxidation rates, copolymers of Hb and bovine serum albumin (BSA) obtained with oATP show essentially no change in autooxidation rates compared to native Hb (Lacob et al. Citation2011). One may in principle expect that BSA would have a similar beneficial effect in other Hb polymerization products.
Disuccinimidyl suberate (DSS, ) is a homobifunctional N-hydroxysuccinimide ester (NHS ester). Its reactivity toward primary amines allows it to serve as a cross-linking agent for proteins, without toxic side-products and forming peptide bonds with the lysine residues in one single step (Pilch and Czech Citation1979). DSS was shown to cross-link Hb intramolecularly, yielding a relatively stable protein, whose oxygen affinity was almost halved compared to that of native Hb; in preliminary tests on rats and guinea pigs, no notable toxicity of this product was reported (Lu et al. Citation2005). Here, we report that DSS may be employed for obtaining Hb polymers as well; as such polymers show increased autooxidation rates, we also report that copolymers Hb and BSA with DSS show very little change in autooxidation compared to native Hb.
Materials and methods
Bovine Hb was purified following a general protocol of Antonini and Brunori (1917). Hb concentrations in the text are given per heme rather than per tetramer. Polymerized Hb (polyHb) was obtained by mixing a native Hb solution with DSS under stirring at room temperature (Pierce Biotechnology, USA) or GL at 4°C (from Sigma, Germany) with or without BSA (BSA, fraction V, from Sigma, Germany) at concentrations indicated in the text. Generally, these concentrations were between 1 and 8 mM; a sample labeled 1 Hb 2BSA 5DSS was prepared using 1 mM Hb, 2 mM BSA, and 5 mM DSS, while a sample labeled 1Hb 8GL contained 1 mM Hb and 8 mM GL. On selected samples, GL polymerization was also applied on DSS-polymerized samples. A 2-fold excess of NaBH4 was added in the case of samples polymerized with GL, in a vessel for 30 min to quench the reaction. After the reaction, polyHb was dialyzed against pH 7.4 phosphate-buffered saline (PBS) or Tris buffer to remove the side-products and excess of NaBH4, respectively.
The polymerized sample was analyzed using 15% SDS–PAGE and gel filtration size exclusion chromatography on Superdex 200 5/150 GL column (GE Healtcare, Suedia), 0.25 mL/min flow rate with a mobile phase of 20 mM Tris buffer, and 150 mM NaCl at pH 7.4. The absorbance was monitored at 280 nm. Molecular weights were determined based on a calibration curve employing a molecular weight standard kit (Sigma-Aldrich) containing carbonic anhydrase (29 kDa), BSA (66 kDa), alcohol dehydrogenase (150 kDa), amylase (200 kDa), apoferritin (443 kDa), thyroglobulin (669 kda), and blue dextran (void volume marker).
Autooxidation experiments for polyHb in PBS solution (pH, 7.4; temperature, 37°C) were carried out in the dark for 4 h. The change in absorbance at 630 nm was used to determine the rate of autoxidation. UV–vis spectra were recorded on Cary 50 (Varian, Inc) instruments.
For electron paramagnetic resonance (EPR) spectra, a Bruker EMX microspectrometer with a liquid nitrogen cooling system was employed. Instrument conditions were as follows: microwave frequency, 9.43 GHz; microwave power, 15.89 mW; modulation frequency, 100 kHz; modulation amplitude, 5 G; sweep rate, 22.6 G/s; time constant, 81.92 ms, average of 3 sweeps for each spectrum; and temperature, 100 K.
The reaction between oxy-Hb and nitric oxide was performed in a stopped-flow UV–vis system. A concentration of 13.3 μM of Hb, in 50 mM pH 10 borate, was mixed with nitric oxide from an anaerobic stock solution of 177.66uM in 20 mM pH 7 phosphate; where needed, the NO solution was pre-diluted using the sequential mixing capability of the instrument, using anaerobic 100 mM pH 10 borate. Stopped-flow spectra were collected on a Biologic SFM-300 system equipped with 3 syringes and capable of sequential mixing, with a high-speed diode array detector. Stopped-flow data were analyzed within the SPECFIT32 software package (BioLogic Science Instruments, France) using singular value decomposition (SVD) and global multi-exponential fitting of the SVD-treated data, with the spectra fitted to simple kinetic models using Levenberg–Marquardt or simplex algorithms.
Results and discussion
shows SDS–PAGE data obtained upon copolymerization of Hb with BSA using DSS, GL, or both. It may be seen that some, but not all the monomers in Hb, have been cross-linked (as is often the case, as only 1 monomer within the tetramer is sufficient for cross-linking with another tetramer).
Figure 2. SDS–PAGE of polyHb. 1: native Hb; 2: 1Hb 8GL; 3: 1Hb 2BSA 8GL; 4: 1Hb 2BSA 5DSS; 6: 1Hb 2BSA 5DSS 8GL; and M: marker.
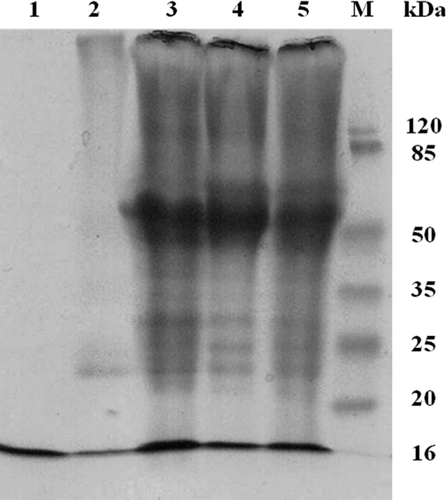
shows gel filtration data illustrating the changes in apparent volume of the Hb induced by polymerization. Based on the calibration curves, the range of molecular weights for the main peaks are the following: native Hb, 64kDa; 1Hb, 8GL: 91–1050kDa; 1Hb, 2BSA 8GL: 146–480kDa; 1Hb, 2BSA 5DSS: 122–354kDa; 1Hb, 2BSA 5DSS 2GL: 92–218kDa.
shows UV–vis spectra of the polymers, as well as their spectra after an autooxidation experiment. The 630-nm features indicate a slightly increased autooxidation rate in the Hb–GL polymer. lists autooxidation rates, dioxygen affinity (in terms of changes in P50), and Hill coefficients. It can be seen that in the copolymers, these changes are reduced compared to the control. Notably, the autooxidation rate for one of the copolymers (Hb–BSA–GL) is even lower than that for the native Hb. The oxygen affinity of Hb–BSA–DSS was found to be 2-fold lower than that of the native bovine Hb, and its cooperativity was lost while for Hb–GL the oxygen affinity is ˜ 9-fold lower than that for the native Hb. For both Hb–BSA–GL and Hb–BSA–DSS–GL, the values of Kd are similar but slower than the native one, while the cooperativity was lost.
Table I. Autooxidation rates (values compared with native Hb), rate constants (Kd), and Hill coefficients (n) for polyHb and native Hb.
shows time courses for the reactions of poly- and copolymerized Hbs with hydrogen peroxide, monitored at 425 nm – a wavelength diagnostic for the high-valent ferryl species known as Compound II and responsible for oxidative damage on proteins and other biomolecules in vitro and in vivo (Reeder et al. Citation2004). It may be seen that the Hb–GL and Hb–BSA–GL (panel A) accumulate less of this potentially dangerous species, but also allow the ferryl to decay faster – while the Hb–BSA–DSS and Hb–BSA–DSS–GL (panel B) accumulate more ferryl species than the native Hb.
Figure 5. The reaction of GL-polymerized Hb (A) and DSS-polymerized Hb (B) with H2O2. Conditions: room temperature, PBS 10 μM protein, 80 μM H2O2.
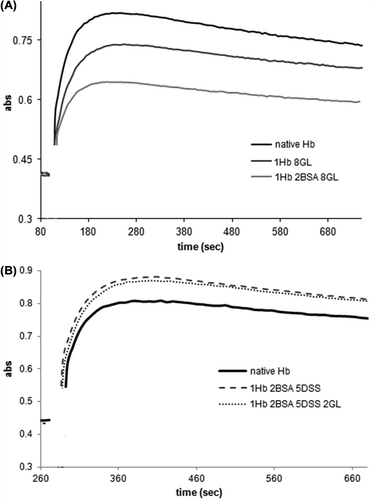
The reaction of oxy-hemoglobin toward nitric oxide rapidly generates met-Hb and can be a problem in transfusions involving acellular HBOCs, where the derivatized Hb can come in close contact with the NO generated in the endothelium (Doherty et al. Citation1998). Upon examining a range of derivatized Hbs and Hb–BSA copolymers (including some with oATP or GL), we found that copolymerization with BSA, as well as cross-linking, did not affect the rate of oxidation of oxy-Hb to met-Hb by more than 5% (data not shown).
shows electron paramagnetic spectra illustrating that copolymers have slightly lower amounts of free radicals generated upon reaction with hydrogen peroxide. The shapes of the free radical signals were not affected by the polymerization procedures, suggesting that the peroxide-induced radical still has the same location – mostly tyrosine and tryptophan (Svistunenko Citation2005).
Figure 6. EPR spectra of 200 μM protein treated with 500 μM H2O2 in PBS and frozen at 30 s after mixing.
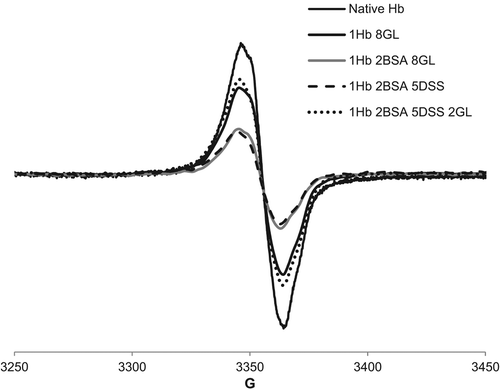
The results obtained for the Hb–albumin copolymers are expected to be useful in a blood substitute. It is known that serum albumin has various biological functions such as ligand binding, free radical-scavenging and anti-inflammatory activity, antioxidant, cell signaling, and inhibition of apoptosis. The antioxidant and pro-oxidant roles of albumin are due to its reduced thiol moieties and to its ability to redox cycle/recycle transition metal ions such as iron (from Hb), respectively (Caron et al. Citation1999, Kratz Citation2008, Trynda-Lemiesz and Luczkowski Citation2004, Yue et al. Citation2009, Tsuchida et al. Citation2009). Apparently, these properties converge toward reducing oxidative stress–related properties with a Hb-based copolymer – which correlates well with positive results obtained in vivo (Fedorov et al. Citation1978).
To conclude, we have shown that DSS may be employed for obtaining Hb polymers and that the increase in autooxidation rate incurred by this polymerization is completely reversed when BSA is copolymerized with Hb. The copolymers show very little change in autooxidation and oxygen affinity compared to the native Hb. BSA has a similar effect on the Hb–GL polymerization system. Together with our previously reported data on oATP–Hb–BSA copolymers, this suggests that copolymerization of Hb with BSA is an efficient strategy for drastically limiting pro-oxidant reactivity in blood substitutes.
Acknowledgements
OZ thanks the “Babes-Bolyai” University for a research scholarship.
Declaration of interest
The authors report no declarations of interest. The authors alone are responsible for the content and writing of the paper.
The work shown here has been supported by the Romanian Ministry for Education and Research (grant PCCE 140/2008) and by a PhD scholarship to FS (Contract POSDRU/88/1.5/S/60185 – “Innovative doctoral studies in a knowledge based society”).
References
- Alayash AI. 2004. Oxygen therapeutics: can we tame haemoglobin?. Nat Rev Drug Discov. 3:152–159.
- Antonini E, Brunori M. 1971. Hemoglobin and Myoglobin in Their Reaction with Ligands. Frontiers in Biology. North-Holland, Amsterdam.
- Caron A, Menu P, Faivre-Fiorina B, Labrude P, Alayash AI, Vigneron C. 1999. Cardiovascular and hemorheological effects of three modified human hemoglobin solutions in hemodiluted rabbits. J Appl Physiol. 86:541–548.
- Chang TM. 2004. Hemoglobin-based red blood cell substitutes. Artif Organs. 28:789–794.
- Chang TMS. 2009. Nanobiotechnology for hemoglobin based blood substitutes. Crit Care Clinics. 25:373–382.
- Chen JY, Scerbo M, Kramer G. 2009. A review of blood substitutes: examining the history, clinical trial results, and ethics of hemoglobin-based oxygen carriers. Clinics (Sao Paulo). 64:803–813.
- Deac F, Lacob B, Fischer-Fodor E, Damian G, Silaghi-Dumitrescu R. 2011. Derivatization of hemoglobin with periodate-generated reticulation agents: evaluation of oxidative reactivity for potential blood substitutes. J Biochem (Tokyo). 149:75–82.
- Deac F, Todea A, Silaghi-Dumitrescu R. 2009. Glutaraldehyde derivatization of hemoglobin: a potential blood substitute. In: Silaghi-Dumitrescu R, Garban G, Eds. Metal Elements in Environment, Medicine and Biology Tome IX. Cluj-Napoca, Romania: Cluj University Press, pp. 165–173.
- Deac FV, Bolfa AM, Magdas C, Sevastre B, Turc S, Silaghi-Dumitrescu R. 2010. Hemoglobin-based blood substitutes: which hemoglobin to use?. Rom J Biochem. 47:135–141.
- Doherty DH, Doyle MP, Curry SR, Vali RJ, Fattor TJ, Olson JS, Lemon DD. 1998. Rate of reaction with nitric oxide determines the hypertensive effect of cell-free hemoglobin. Nat Biotechnol 16:672–676.
- Fedorov AN, Iarochkin VS, Koziner VB, Hachaturian AA, Rozenberg GI. 1978. Support of respiration and hemodynamics by complete blood replacement with artificial oxygen carriers: polyhemoglobinalbumin and polyhemoglobin. Dokl Akad Nauk SSSR. 243:1324–1326.
- Harris DR, Palmer AF. 2008. Modern cross-linking strategies for synthesizing acellular hemoglobin-based oxygen carriers. Biotechnol Prog. 24:1215–1225.
- Kratz F. 2008. Albumin as a drug carrier: design of prodrugs, drug conjugates and nanoparticles. J Contr Release. 132:171–183.
- Lacob B, Deac F, Cioloboc D, Damian G, Silaghi-Dumitrescu R. 2011. Hemoglobin-albumin crosslinked copolymers: reduced prooxidant reactivity. Artif Cells Blood Substit Immobil Biotechnol 39:293–297.
- Lu X, Zheng C, Xu Y, Su Z. 2005. Disuccinimidyl suberate cross-linked hemoglobin as a novel red blood cell substitute Sci China C Life Sci. 48:49–60.
- Pilch PF, Czech MP. 1979. Interaction of crosslinking agents with the insulin effector system of isolated fat cells. J Biol Chem. 254: 3375–3381.
- Portoro I, Kocsis L, Herman P, Caccia D, Perrella M, Ronda L, et al. 2008. Towards a novel haemoglobin-based oxygen carrier: Euro-PEG-Hb, physico-chemical properties, vasoactivity and renal filtration. Biochim Biophys Acta. 1784:1402–1409.
- Reeder BJ, Grey M, Silaghi-Dumitrescu RL, Svistunenko DA, Bulow L, Cooper CE, Wilson MT. 2008. Tyrosine residues as redox cofactors in human hemoglobin: implications for engineering nontoxic blood substitutes. J Biol Chem. 283:30780–30787.
- Reeder BJ, Svistunenko DA, Cooper CE, Wilson MT. 2004. The radical and redox chemistry of myoglobin and hemoglobin: from in vitro studies to human pathology. Antioxid. Redox Sign. 6:954–966.
- Svistunenko DA. 2005. Reaction of haem containing proteins and enzymes with hydroperoxides: the radical view. Biochim Biophys Acta. 1707:127–155.
- Trynda-Lemiesz L, Luczkowski M. 2004. Human serum albumin: spectroscopies studies of the paclitaxel binding and proximity relationship with cisplatin and Adriamycin. J Inorg Biochem. 98:1851–1856.
- Tsuchida E, Sou K, Nakagawa A, Sakai H, Komatsu T, Kobayashi K. 2009. Artificial oxygen carriers, hemoglobin vesicles and albumin-hemes, based on bioconjugate chemistry. Bioconjug Chem. 20:1419–1440.
- Winslow RM. 2004. MP4, a new nonvasoactive polyethylene glycol-hemoglobin conjugate. Artif Organs. 28:800–806.
- Yue Y, Chen X, Qin J, Yao X. 2009. Spectroscopic investigation on the binding of antineoplastic drug oxaliplatin to human serum albumin and molecular modeling. Colloids Surfaces B. 69:51–57.
- Zolog O, Mot A, Deac F, Roman A, Fischer-Fodor E, Silaghi-Dumitrescu R. 2011. A new polyethyleneglycol-derivatized hemoglobin derivative with decreased oxygen affinity and limited toxicity. Protein J. 30:27–31.