Abstract
Cancer is one of the most important causes of death all over the world, which has not yet been treated efficiently. Although several therapeutic approaches have been used, some side effects such as toxicity and drug resistance have been observed in patients, particularly with chemotherapy. The nanoparticle-mediated drug delivery systems (DDS) have a great potential to improve cancer treatment by transferring therapeutic factors directly to the tumor site. Such a treatment significantly decreases the adverse effects associated with cancer therapy on healthy tissues. Two main strategies, including passive and active methods, have been considered to be effective techniques which can target the drugs to the tumor sites. The current review sheds some light on the place of nanotechnology in cancer drug delivery, and introduces nanomaterials and their specific characteristics that can be used in tumor therapy. Moreover, passive and active targeting approaches focus on antibodies, particularly single chain variable fragments (scFv), as a novel and important ligand in a drug delivery system.
Introduction
Cancer has been considered as the second cause of death after heart disease, accounting for around 23% of all deaths in the United States (CitationJemal et al. 2008). Breast and prostate cancers are the most common cancers in women and men, with the probability of 29% and 28% respectively (CitationHajdu 2011). The precise etiology of cancer is unknown. Although the genetic factor is involved in 5–10% of cancers, other factors including the use of tobacco, poor diet, certain infections, lack of physical activity, obesity, and pollution may also directly damage genes and ultimately lead to carcinogenic mutations (CitationAnand et al. 2008).
Surgery, as a primary therapeutic approach to treat solid tumors, can lead to pain relief and patients’ survival (CitationMiller et al. 1981). Radiation and chemotherapy, which destroy cancerous cells, are other therapeutic approaches for tumors (CitationBrannon-Peppas and Blanchette 2012). The tumor-specific therapy is the main objective in the treatment of different cancers, which has not been addressed in previously mentioned tumor therapeutic approaches (CitationPerez 2005). As these drugs do not act selectively against the cancer cells, toxic effects of the chemotherapeutic drugs are expected (CitationBenita 2005). Moreover, non-specific distribution of drugs results in the need for administration of higher drug volumes, which increases the cost and toxicity (CitationPerez 2005).
The improvements in cancer therapy are developing not only in the production of new agents against cancer but also in designing new drug delivery methods for both old and new agents. It is expected that such an advancement can be effective in decreasing the required doses of therapeutic drugs in cancer therapy (CitationBrannon-Peppas and Blanchette 2012). Drug delivery systems (DDS) have recently been developed to reduce the side effects of chemotherapy and to improve the efficiency of the existing drugs. In an organized DDS, the drug is carried to the target and therefore its influence on non-targeted tissues and unwanted side effects can be avoided. Moreover, a DDS reduces the rapid degradation or clearance of the drug and increases drug concentration in the target tissues (CitationNevozhay et al. 2006). Among these systems, it seems that nanocarriers are a promising option in the field of drug delivery (CitationPark et al. 2009). The unique physicochemical and biological features of nanoparticles make them excellent candidates in cancer therapy (CitationBrannon-Peppas and Blanchette 2012).
In this review, we have tried to discuss the nanoparticles as DDS and their great potential to destroy tumor cells. However, we will describe single chain variable fragment (scFv)-targeted nanoparticles in more detail compared to other nanoparticles.
Nanoparticles and drug delivery
Nanoparticles have various physicochemical characteristics which make them interesting in the treatment of cancer. Since the size of the nanomaterials is smaller than the size of a cell, they can transport large amounts of agents, multiple drugs, or probes onto the surface or to the inside of the cell, without disturbing its function. The small size of such particles enables them to cross through small vessels and penetrate tissues rapidly. These particles improve the therapeutic index and half-life of the drug in blood circulation, with reduced side effects and no platelet activation. Additionally, nanoparticles are able to increase the bioavailability of water-insoluble drugs and protect the therapeutic agents from physiological barriers. Such small particles provide sufficient surface area and strategies for rapid drug release (CitationConti et al. 2006, CitationHu et al. 2010, CitationSwami et al. 2012). Therapeutic drugs can be adsorbed, covalently attached, or even encapsulated in nanoparticles. When the drug-loaded nanocarrier reaches the site of action, the therapeutic agents will be released (CitationWilczewska et al. 2012).
An important issue in the production of nanoparticles is their stability and rapid clearance in blood circulation. Therefore, particular parameters, including physicochemical properties which can affect the distribution of nanomaterials, have to be investigated precisely (CitationShenoy and Amiji 2005). Generally, nanocarriers ranging in size from 10 to 100 nm have desirable pharmacokinetic properties in vivo. Smaller nanoparticles are removed through tissue extravasations and renal clearance, whereas larger particles are rapidly opsonized with plasma proteins and captured by phagocytes. It has been shown that the particle size plays a crucial role in the blood bio-distribution of nanoparticles (CitationCole et al. 2011). Moreover, the surface charge of the nanocarriers may affect their uptake by macrophages in the liver and spleen. It was found that neutrally charged particles have lower clearance rates than charged particles (CitationSwami et al. 2012). However, the cationic surface enhances the interaction of the nanoparticles with the cells and improves the rate and extent of internalization (CitationShenoy and Amiji 2005). Prolonged circulation half-life can be achieved by the conjugation of nanoparticles with hydrophilic polymers such as polyethylene glycol (PEG), which has favorable properties including low toxicity, immunogenicity, high flexibility, and hydrophobicity (CitationSwami et al. 2012). PEGylation results in the creation of a cloud of chains around the nanoparticles, which ultimately repels plasma proteins and inhibits the opsonization process (CitationOwens III and Peppas 2006).
Liposomes, solid lipid nanoparticles, dendrimers, polymers, silicon, carbon, and magnetic particles are the various nanocarriers assessed as DDS. Although several nanoparticle-based chemotherapeutic delivery systems are available in the market, few nanoparticles are currently used in various stages of clinical or preclinical studies (as shown in ).
Table I. Examples of nanoparticles being marketed or undergoing clinical studies.
Liposomes
Liposomes (80–300 nm) are the first particles designed as nanocarriers (CitationSunderland et al. 2006) which contain amphiphilic lipid molecules (phosphatidyl ethanolamine and phosphatidyl choline) with cholesterol to enhance their stability and rigidity accumulated into bilayer vesicles. External energy from sonication, homogenization, shaking, or heating is required for the assembly of these particles (CitationCouvreur and Vauthier 2006, CitationHu et al. 2010). It has been demonstrated that liposomes increase the solubility of drugs and enhance their in vitro and in vivo anticancer activity, reduce the undesirable side effects, and increase pharmacokinetic properties such as the therapeutic index of chemotherapeutic agents (CitationSantos Giuberti et al. 2011). The drug release in liposomes, by adsorption, fusion, endocytosis and lipid transfer, depends on the liposome's features such as pH and osmotic gradient, and the surrounding environment (CitationSantos Giuberti et al. 2011, CitationWilczewska et al. 2012).
Various drugs including anti-cancer drugs, antibiotics, anti-inflammatory drugs, neurotransmitters (serotonin), and anti-rheumatic drugs can be encapsulated in liposomes (CitationWilczewska et al. 2012). Recent liposomal formulations such as Doxil, DaunoXome®, DepoCyt® and ONCO-TCS are in the world market, and are loaded with Doxorubicin, Danarubicin, Cytarabine and Vincristine, respectively (CitationHaley and Frenkel 2008, CitationHu et al. 2010). In addition, they have a particular structure which can simultaneously load hydrophilic drugs in the aqueous core and hydrophobic drugs in the lipid membrane (CitationHu et al. 2010).
The main problem of liposomes as DDS is their non-specific accumulation outside the target tissues, which may lead to the death of normal cells (CitationDaemen et al. 1995). Modified liposomes containing specific proteins, antigens, or other biological substances can be applied to selectively deliver drugs for a particular tissue. Due to these unique characteristics, they have attracted great interest from the researchers. Furthermore, cationic liposomes (CLs) would be a great choice in gene delivery compared to natural or anionic liposomes (CitationWilczewska et al. 2012).
Nanoparticles based on solid lipids
The solid lipid matrix-based nanoparticles are types of nanocarriers applied in ocular, pulmonary, rectal, dermal, per-oral and parenteral delivery (CitationWilczewska et al. 2012). Some examples of these structures are SLN (solid lipid nanoparticles), LDC (lipid drug conjugates) and NLC (nanostructured lipid carriers) (CitationWissing et al. 2004). SLNs, or lipospheres or nanospheres, are made from solid lipids consisting of highly purified triglycerides, waxes or complex glyceride mixtures stabilized by various surfactants which have specific properties such as suitable physical stability and tolerability, controlled drug release, site-specific targeting, and protection of labile drugs from degradation. However, there are some disadvantages regarding these nanoparticles, including their limited loading capacity and drug leakage after SLN crystallization (CitationKovacevic et al. 2011, CitationWilczewska et al. 2012, CitationWissing et al. 2004).
LDC and NLC are modified lipids in which some SLN restrictions are resolved. The main purpose in the development of LDC is to improve the applicability of lipid-based carriers to lipophobic molecules. NLCs are lipid carriers containing a certain structure for the enhancement of payload and the prevention of drug expulsion. As a result, most of the applications of such nanolipids are topical (Davis 2008).
Polymeric nanoparticle
Polymeric nanoparticles (PNPs) have a diameter ranging from 10 to 100 nm, containing natural (albumin, gelatin, chitosan and polysaccharide) or synthetic (polyacrylamide, e-poly caprolactone and polyacrylate) polymers (CitationHu et al. 2010, CitationWilczewska et al. 2012). Some polymers, such as poly (lacticoglycolic acid) (PLGA) and polycaprolactone (PCL), have also been approved by the Food and Drug Administration (FDA) to be used as synthetic polymers (CitationHu et al. 2010).
Drug-loaded polymeric nanocarriers bear a significant impact on the clinical approach by improving the pharmaceutical efficacy, controllable drug-release profiles, and high loading capacity for relatively insoluble drugs. Due to these features, polymeric nanoparticles have attracted academic, clinical, and industrial interest, although they still are in the early stages of development (CitationDavisand Shin 2008, CitationHu et al. 2010).
PNPs are classified as biodegradable (poly (L-lactide) and polyglycolide) and non-biodegradable (CitationWilczewska et al. 2012). Biodegradable nanocarriers have numerous advantages, such as high stability and less immunogenicity (Citationdes Rieux et al. 2006). Although a minimal systemic toxicity has been assigned by Kumari et al. (CitationKumari et al. 2010) for using PLGA in drug delivery applications, it has been shown that PLGA is a suitable choice for the delivery of hydrophobic drugs, since they can degrade in the body and produce biodegradable metabolite monomers, such as lactic acid and glycolic acid (CitationHu et al. 2010, CitationWilczewska et al. 2012).
Magnetic nanoparticles
Among the wide range of nanomaterials, magnetic nanoparticles (MNPs) have attracted considerable attention in biomedical applications due to their ability to be handled by an external magnetic field, their great potential in imaging, high uptake by the target tissue, and improving the treatment in therapeutically optimal doses (CitationArruebo et al. 2007, CitationSun et al. 2008).
Normally, MNPs are composed of a magnetically active core covered by various shells to anchor target ligands or implement extra imaging modalities. Therapeutic drugs can then be set in the shell structure or chemically attached to their surface (CitationVeiseh et al. 2010).
Based on their magnetic features, MNPs can be divided into pure metals (e.g. manganese, iron, cobalt, and nickel), their alloys, and oxides (CitationWilczewska et al. 2012). Iron oxide nanoparticles have desirable properties such as chemical stability and probable chemical modification by coating the iron oxide cores with various shells (e.g. polymeric, gold, silane) and are also considered as the only type of MNPs that have been approved by the FDA for clinical applications (CitationFidalgo et al. 2009, CitationWilczewska et al. 2012). Furthermore, iron oxide can be produced naturally in the human heart, spleen, and liver (CitationGrassi-Schultheiss et al. 1997) which indicates its non-toxicity and biocompatibility in the body.
The effect of iron oxide on cellular function, and its toxicity, have been also investigated. Depending on the injection route, MNPs can interact with extracellular agents, macrophage cell membrane, endothelial cells, skin epithelium, and respiratory tracts (CitationWilczewska et al. 2012). Studies have shown that iron oxide nanoparticles may induce oxidative stress by increasing ROS production, which results in augmented expression of hemoxygenase-1 and superoxide dismutase as antioxidant enzymes, followed by the release of proinflammatory components. These events can lead to apoptosis and mutagenesis (CitationNaqvi et al. 2010).
Silica materials
Silica materials are divided into two categories, including xerogels and mesoporous silica nanoparticles (MSN) (CitationHu et al. 2010). Among inorganic nanomaterials, silica particles are the most promising choice in biological applications. These nanoparticles contain several advantages, such as biocompatibility, a highly porous structure, and ease in terms of functionalization (CitationAmato 2010).
Silica xerogels include an amorphous structure with high porosity. The shape and size of the pores are variable, depending on the parameters of synthesis (CitationEcheverría et al. 2010). For example, sol-gel techniques are commonly applied to form silica xerogels embedded with drugs, therefore a change in production conditions, including the ratio of reagents, temperature, and concentration of catalyst, may alter the properties of xerogels (CitationWilczewska et al. 2012).
MSN, on the other hand, form homogenous structures with higher surface area, which is favorable for the adsorption of therapeutic drugs (CitationDi Pasqua et al. 2009). These particles possess the ability to load large and small molecules, adsorption of DNA, and gene transfer (CitationWilczewska et al. 2012). A variety of drugs including antibiotics (CitationLi et al. 2010), anticancer drugs (CitationDi Pasqua et al. 2009), and heart disease drugs (CitationPopovici et al. 2011) are loaded into MSNs via chemical or physical adsorption mechanisms (CitationDi Pasqua et al. 2009).
Although several studies have indicated that silica particles are biocompatible and have favorable diagnostic and therapeutic properties, some studies have however shown in vitro and in vivo toxicity of silica nanoparticles (CitationWilczewska et al. 2012). Depending on the type of cells and the size of the nanoparticles, silica nanoparticles can induce oxidative stress through the generation of reactive oxygen species (CitationLiu and Sun 2010), reduction of glutathione levels (CitationKyung et al. 2009), and induction of anti-oxidant enzymes (superoxide dismutase (SOD) and hemooxygenase-1 (OH-1)) (CitationPark and Park 2009), which ultimately disturbs the cell membrane (CitationLin et al. 2006).
Dendrimer Nanocarriers
Dendrimers are unique polymers with repetitively branched structures. Glycogen, amylopectin, and proteoglycans are examples of such structures (CitationSvenson and Tomalia 2012). Dendrimers are spherical compounds and are generally symmetrical around their core. The biocompatibility and biochemical properties of the tree-shaped structure (dendron), which contains a chemical group called the focal group, depend on the surface dynamic groups (CitationCaminade et al. 2005). The amount of drug loaded on dendrimers can also be varied, based on the drug properties. Toxic, unstable, or poorly soluble agents encapsulated in a carrier will enable the possibility of controlling the quantity of drugs on the nanoparticle surface through the control of the number of covalent bonds (CitationSingh et al. 2008).
Polyamidoamine (PAMAM) is one of the most important dendrimers, and has a great potential to carry small drug particles (CitationWilczewska et al. 2012). The cationic form of PAMAM is a very potent vehicle for the delivery of macromolecular agents such as DNA, through cellular and subcellular barriers (CitationPatri et al. 2005, CitationYellepeddi et al. 2009).
Carbon nanomaterials
Carbon nanoparticles used in DDS are divided into two categories, including carbon nanotubes (CNTs) and carbon nanohorns (CNH). Nanotubes have a special structure composed of one or more layers of graphite tubes (SWNCTs – single-walled carbon nanotubes, or MWCNTs – multi-walled carbon nanotubes) with an extended surface area and exceptional electronic and thermal conductivity (CitationBeg et al. 2011). The biocompatibility of nanotubes could be improved by chemical modification of their surface (CitationFoldvari and Bagonluri 2008). PAMAM dendrimers can covalently attach to the surface area of nanotubes and amplify their functions (CitationZhang et al. 2010).
Drugs can be loaded through different methods, such as encapsulation, chemical adsorption on the surface or in the space between the nanotubes, and attachment of active elements to functionalized carbon nanotubes. Drug encapsulation in a carbon nanotube is more applicable compared to the other methods, since it protects the drug from degradation during its crossing to the cells, and the drug will be released only in specific conditions (CitationWilczewska et al. 2012). Furthermore, in order to prevent the undesirable drug release, the tips of CNTs can be closed with polypyrrole (PPy) films (CitationLuo et al. 2011).
Nanohorns (a type of single-layer nanotube) have properties similar to those of nanotubes; however, they can be produced with lower cost and higher purity (CitationShiba et al. 2006). Drugs can be loaded on these carbon nanoparticles through surface adsorption (CitationMurakami et al. 2008) or nano-precipitation. It has been demonstrated that nano-precipitation is more effective compared to the adsorption method, with respect to the nanohorns (a 3-fold increase in the number of molecules was observed in nanohorns) (CitationAjima et al. 2008).
The toxicity of carbon nanoparticles depends on their exceptional geometric structure, which is well-defined (CitationJia et al. 2005). For instance, the toxicity of nanotubes depends on the length to diameter ratio of the particle, and also the toxicity of the graphite. Additionally, some impurities, like residual metal and amorphous carbon, contribute to the increased levels of reactive oxygen species (ROS) and induced oxidative stress in cells (CitationDobrovolskaia and McNeil 2007).
Targeted drug delivery
As mentioned earlier, conventional chemotherapeutic drugs are distributed nonspecifically in the human body where they affect both cancerous and normal tissues, which ultimately lead to inadequate treatment and high toxicity. Targeted therapy has proved to be an effective method in overcoming the lack of specificity of conventional chemotherapeutic drugs (CitationRoss et al. 2004).
The strategy of nanoparticle targeting is of great importance in targeted therapy. In order to maximize drug delivery in cancer therapy, the administered drugs are required to pass through the body barriers with minimal loss in their activity and volume in the blood circulation. After reaching the desired region, the drugs should be able to selectively destroy the tumor cells, without affecting normal cells, in a controlled release manner (CitationCho et al. 2008).
Cell-specific targeting with nanocarriers may be accomplished by using active or passive mechanisms (CitationAllen 2002, CitationCho et al. 2008, CitationMaeda 2001). In the next section, we will discuss passive and active mechanisms of drug delivery.
Passive targeting strategy
The optimization of the size and surface characteristics of nanoparticles will increase their ability to circulate in the bloodstream for a longer time, and consequently, provide greater chance for reaching specific tumor sites. The unique pathophysiologic features of tumor vessels enable macromolecules, including nanocarriers, to selectively accumulate in tumor tissues, which is the main goal of a passive targeting strategy (CitationCho et al. 2008).
One of the critical features of tumors is the inherent leaky vasculature present in cancerous tissues (CitationBrannon-Peppas and Blanchette 2012). Fast-growing cancer cells require the employment of new vessels (neovascularization) or rerouting of existing vessels near the tumor mass, to supply necessary materials. The imbalance of angiogenic regulators can cause the tumor vessels to appear disorganized, with enlarged gap junctions between endothelial cells, and compromised lymphatic drainage (CitationCarmeliet and Jain 2000). These features mentioned are expected to enhance the permeability and retention effect (EPR effect), which establish an important mechanism by which nanocarriers can extravagate into the tumor tissues rapidly by the EPR effect. Moreover, poor lymphatic drainage in tumors retains the accumulated nanomaterials and allows the release of toxic agents into the vicinity of the tumor cells (CitationPeer et al. 2007a). By means of these features, concentrations of drug-loaded nanoparticles in tumor tissue can reach levels 10 to 100 times higher compared to the levels achieved in the administration of the free drug (CitationSinha et al. 2006).
The unique tumor microenvironment, which is different from that of normal cells, is also another major contributor to passive targeting. Some drugs can be administered as pro-drugs or inactive forms and remain inactive until they reach the tumor. The tumor environment is then able to convert the drug into an active form, which is called tumor-activated pro-drug therapy. Furthermore, fast-growing cancer cells show a high metabolic rate, for which oxygen supply and sufficient nutrients are not usually satisfactory. Consequently, tumor cells use glycolysis to obtain extra energy, resulting in an acidic environment (CitationPelicano et al. 2006, CitationSinha et al. 2006). The pH-sensitive nanoparticles such as liposomes are designed to remain stable at a physiologic pH (7.4), but degrade in the acidic environment of tumor cells and release the active form of drugs (CitationYatvin et al. 1980). In addition, most linkers in nanocarrier-conjugated drugs are peptidase-sensitive or acid-labile (CitationSuzawa et al. 2002). For example, the overexpression of the matrix metalloproteinase-2 in melanoma has been observed in preclinical and clinical studies. Matrix metalloproteinase-2 plays a critical role in the degradation of basement membranes and the extracellular matrix (CitationSinha et al. 2006). Based on the studies reported by Mansour et al., a water-soluble maleimide derivative of doxorubicin, which is an important chemotherapeutic drug, incorporates a matrix metalloproteinase-2-specific octapeptide sequence between the drug and the carrier. This polymer-drug conjugate was found to have a high affinity for the cysteine-34 position of circulating albumin. The albumin-bound form was efficiently and specifically cleaved by the matrix metalloproteinase- 2 that led to the release of free doxorubicin (CitationMansour et al. 2003).
Another approach that allows the passive delivery of therapeutic agents through nanocarriers, is the localized administration of drug-loaded nanoparticles. Various methods have been observed to improve the tumor delivery of anticancer drugs, like intrapretoneal and intravesical injections, which directly transfer the drug to the tumor tissue, without circulating throughout the body. Direct inoculation of the drug into tumors is also an attractive approach that has been examined (CitationYockman et al. 2003). For instance, direct administration of mitomycin into target tissue resulted in an enhanced concentration of the drug at the tumor site and declined toxicity (CitationNomura et al. 1998).
The disadvantage of localized drug delivery through intratumoral administration is that such approaches may result in exposure to higher concentrations of therapeutic agents, which is not always feasible and can be highly invasive. Additionally, sometimes the site of the tumor is not clear, and access to some cancers such as lung cancer would be complicated in such cases (CitationSinha et al. 2006, CitationYockman et al. 2003).
In addition, although passive targeting strategies incorporate the basis of clinical therapy, they have several limitations that may not bring about desirable clinical outcomes. Moreover, targeting cells within a tumor is not always possible. Since some drugs cannot diffuse efficiently, the random nature of the approach makes it difficult to control the process, which ultimately leads to the encouragement of multiple-drug resistance (MDR). It has been also observed that certain tumors do not show the EPR effect, and the permeability of tumoral vessels may not be homogenized in a single tumor. A few limitations were also observed due to the lower potency of some drugs after being linked to target moieties when the targeting portion is not cleaved correctly (CitationPeer et al. 2007a, CitationSuzawa et al. 2002, CitationYockman et al. 2003).
Active targeting strategy
In order to overcome the limitations of passive drug delivery, various active strategies have been developed. In such techniques, nanocarriers will actively bind to specific cells after extravasation, which can be accomplished by conjugating the surface of the nanoparticle to a targeting moiety (CitationFerrari 2005, CitationTorchilin 2005). Nanocarriers can identify target cells through a receptor-ligand binding (as shown in ) and the internalization of bound carrier occurs prior to the drug separation, allowing a selective accumulation of the drug in the tumor tissues (CitationSinha et al. 2006). In the preparation of nanoparticles with a ternary structure (a targeting ligand, a polymer or lipid carrier, and a chemotherapeutic agent), some aspects must also be considered to achieve more efficient delivery systems (CitationCho et al. 2008).
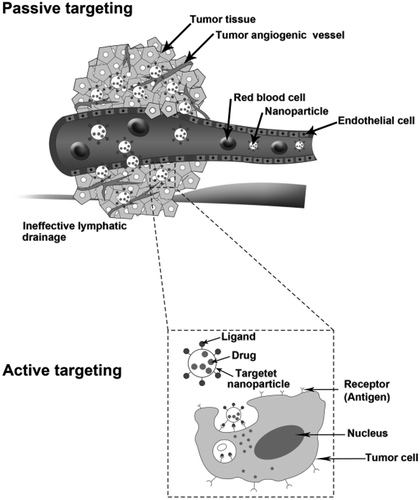
First, cell-surface antigens and receptors should be expressed only on tumor cells and not on host cells (CitationCho et al. 2008), or overexpressed on target tissues compared to normal tissues (CitationPeer et al. 2007a). Although most of the receptors are beneficial for tumor targeting and are often expressed on various types of cells (CitationSinha et al. 2006), the folate receptor is a recognized tumor marker that is highly overexpressed on the surface of different cancers, such as breast, kidney, lung, brain and ovarian cancers. These receptors bind to folate (vitamin B9), and then the folate-targeted nanoparticle transfers via receptor-mediated endocytosis (CitationLeamon and Reddy 2004). Cho et al., (CitationCho et al. 2008) showed the expression of the folate receptor in 53% of human head and neck primary and metastatic tumor tissues compared to bone marrow tissues as normal cells which never expressed this receptor. Transferrin is another active targeting approach, because transferrin receptors are overexpressed in various tumor cells (CitationCho et al. 2008, CitationQian et al. 2002). It would be ideal if the regulation of the target marker or receptor happens through its attached ligands. Hence, endocytosis occurs quickly and thoroughly, by which the receptors will return to the surface of the cancer cells as soon as the active form of drug is released (CitationSinha et al. 2006).
Moreover, active targeting is particularly critical for the delivery of bio macromolecules such as DNA and siRNA, in which intracellular delivery for bioactivity is needed (CitationFarokhzad and Langer 2009). Therefore, internalization of the drug-carrier complex can be achieved through the interaction of certain ligands and their surface receptors (CitationAllen 2002, CitationPastan et al. 2006, CitationPeer et al. 2007b). The drug release may occur in lysosomes by lysosomal enzymes, causing the release of free drug into the cytoplasm. The receptors or antigens released from their ligands should return to the cell membrane to start the second cycle of transport by binding with new ligand-targeted nanoparticles (CitationSinha et al. 2006). It has been shown that immunoliposomes targeted to B-cell lymphoma, labeled by an internalizing anti-CD19 ligand, have more significant therapeutic effects in comparison with a non-internalizing anti-CD20 ligand. However, it should be noted that targeting non-internalizing receptors to nanocarriers may be valuable in solid tumors, where cells lacking the target receptor can be eradicated via drug release in the area of the neighboring cells (bystander effect) (CitationAllen 1994, CitationSapra and Allen 2002). To reach the optimal conditions, some variables such as cell specificity, ligand biocompatibility, binding affinity, and avidity should be considered (CitationAllen 2002). Ligands used in drug-carrier conjugates require high specificity and avidity for cell surface receptors. Kato et al. (CitationKato et al. 1996) reported that ligands should have high affinity for their receptors in order to be effective and greatly stimulate the internalization of polymeric particles.
Although high binding affinity will increase the targeting specificity, there is evidence which shows that the higher binding affinity may result in reduced penetration due to the ‘binding-site barrier’ which is created when the nanocarrier binds to its target so firmly (CitationAdams et al. 2001, CitationAllen 2002). There are other targeting ligands such as proteins (mostly antibodies and their fragments), nucleic acids (aptamers), and others agents (carbohydrates, vitamins, and peptides) (CitationPeer et al. 2007a). Among these ligands, antibodies and antibody fragments contain specific sites incorporated into drug carrier systems with high specificity for cellular receptors and a broad range of binding affinities, for more effective and less intrusive cancer therapy (CitationTorchilin 2008). Other features, including immunogenicity, biodegradability, internalization rate, intracellular routing, and physiochemical properties, have also been studied for antibody-dependent drug delivery systems. Thus, the effective antibodies for optimum-targeted immunotherapy should easily integrate into a nanoparticle, specifically bind to tumor cells, have the ability to efficiently lead to internalization of the drug, and most importantly, be biodegradable (CitationSinha et al. 2006).
The antibody and its single chain Fv fragment in drug delivery
The first report regarding the tumor-targeting ability of monoclonal antibodies was published in 1975 (CitationKöhler and Milstein 1975). However, the application of antibodies in cancer therapy was determined 20 years later (CitationAbou-Jawde et al. 2003). During the past decade, the possibility of antibody targeting has been revealed clinically with the number of monoclonal antibodies (mAbs) approved by the FDA (CitationAbou-Jawde et al. 2003, CitationGlennie and van de Winkel 2003). Recent developments in antibody engineering have led to the development of chimeric, humanized, and fully human mAbs to reduce their immunogenicity (CitationWang et al. 2008).
On the other hand, mAb-conjugated nanoparticles show some limitations, since mAbs are complex and large molecules (∼150 kDa), which particularly restrict their penetration into solid tumors and require significant engineering at the molecular level to be suitable for drug delivery (CitationBrennan et al. 2004, CitationWeinberg et al. 2005). Consequently, mAbs have been engineered to improve their therapeutic abilities, which has led to the production of antibody fragments such as Fab (fragments of antigen binding), Fab'2, single chain Fv fragments (scFv), diabodies, and minibodies (CitationPavlinkova et al. 2001). They are smaller in size and have lower molecular weight and complement activation potential, while they have potent antigen-binding specificity (CitationCarter 2001). Examples of antibody fragments used in nanoparticle structures as cancer therapy are shown in .
Table II. Tumor-targeted nanoparticles with antibody fragments in clinical applications.
At first, scFv fragments were mainly obtained from hybridoma cells of immunized animals, with amplification of the mRNA of VH and VL domains to couple with each other through a polypeptide linker, and inserted in a certain vector (CitationTassew et al. 2009). Recently, in vitro display technologies such as phage and yeast have been considered to surpass the traditional hybridoma technique, with better scFv binding properties in a great diversity of population (CitationBradbury et al. 2011, CitationSidhu and Fellouse 2006). These in vitro exhibitions could be achieved from natural and synthetic libraries of antibodies (CitationSidhu and Fellouse 2006). Synthetic libraries especially remove the need to make animals suffer in order to obtain antibodies through immunization, and also provide excessive diversity in scFv construction by manipulating the CDR regions of synthetic DNA (CitationBradbury et al. 2011, CitationSidhu and Fellouse 2006). The Phage display technique as a successful method has been developed to display recombinant antibodies at the tips of the phage, and consequently, facilitates the generation of scFv antibodies (Wu et al. 2010) easily and inexpensively just by infection of the E. coli (Wang et al. 1995). Phage display as a well-established and widely used method can generate mutants of scFv with higher affinity by manipulating their genes (CitationAhmad et al. 2012).
Advantages and disadvantages of single chain Fv fragments (scFvs)
The scFv fragments have provided great potential compared to full length antibodies in diagnostic and therapeutic applications, with 35% of antibody fragments used in clinical trials (CitationMonnier et al. 2013). ScFv fragments are single polypeptide chains with low functional antigen-binding regain of an antibody (∼30 kDa), in which the variety of heavy (VH) and light (VL) chains are linked together by a flexible peptide (CitationMaynard and Georgiou 2000). Minimized fragments have several advantages over the full-length antibody in clinical applications. The scFv fragments show better penetration through solid tumors, reduced retention time in non-targeted tissues, faster renal clearance, and more importantly, lower immunogenicity. ScFv antibodies can be constructed easily and their functions can be improved by manipulation of their structures which leads to the production of fragments with low commercial cost in large-scale yields. Furthermore, scFv fragments could be genetically manipulated to generate multivalent and multifunctional derivatives with more avidity, like diabodies, triabodies and tetrabodies (CitationBrannon-Peppas and Blanchette 2012). Owning to their small size, scFv antibodies have lower half-life in circulation, resulting in restricted exposure to radionuclide or drug molecules, which is desirable in imaging applications (CitationAhmad et al. 2012, CitationMonnier et al. 2013). In addition, the Fc domain of a mAb can also bind to the Fc receptor-expressing normal cells. This may be due to the undesirable activation of the Fc receptor on non-target cells and increased toxicity through cytokine release (CitationHolliger and Hudson 2005). Recombinant fragments like scFv fragments lacking the Fc domain have another advantage over the intact antibodies (CitationAhmad et al. 2012).
However, one disadvantage of the in vivo application of these fragments is low retention in target tissues due to their monovalent binding (CitationHolliger and Hudson 2005). Although the small size of scFv fragments confer several properties in tissue penetration resulting in short half-life circulation and rapid off rate, the exposure of the scFv fragment to the target is ultimately limited. To solve these problems, multivalent and multifunctional fragments derived from scFv have been engineered. Genetic manipulation has led to the design of diabodies, triabodies and tetrabodies that have higher avidity and increased maintenance of circulation, thus promoting tumor uptake for a long time without compromising tissue penetration properties (CitationHudson 1998). It has been demonstrated that the optimal structure which has both high tissue penetration and enough retention with rapid clearance would be a diabody (55 kDa), which is constructed by non-covalently linking two scFv fragments (CitationRobinson et al. 2005, CitationSundaresan et al. 2003).
scFv fragments in targeted cancer therapy
The scFv fragments have gained significant attention in both diagnosis and therapy due to their advantages over conventional antibodies (CitationWan et al. 2013). Therefore, most clinical research studies in the United States, China, and the United Kingdom are focused on scFv fragments, particularly cancer therapy (CitationPucca et al. 2011).
The ScFv antibodies, with an ability to bind a specific antigen which is exclusively expressed on tumor cells, can be conjugated to toxins, small interfering RNA (siRNA), and nanoparticles, and therefore can be considered as a promising approach in DDS (CitationMonnier et al. 2013). For instance, in 2007, Zhou and coworkers demonstrated that the liposome, coupled with single-chain antibody fragment against epidermal growth factor receptor (EGFR), can specifically recognize cancer cells overexpressing EGFR and increase the uptake of these anti-EGFR immunoliposomes (CitationZhou et al. 2007). Yang et al. obtained similar results by using functionalized quantum dots or magnetic iron oxide nanocarriers targeted with scFv directed against EGFR (scFv EGFR) into an orthotropic pancreatic cancer (CitationYang et al. 2009). In another study, the scFv antibody (scFv-CM6) has been isolated against an extracellular part of a protein expressed on newly formed blood vessels, cancer cells, and tumor endothelial marker 1 (TEM1), by the phage display technique. ScFv-CM6 was coupled to a liposomal nanoparticle loaded with N4- octadecyl-1-beta-d-arabinofuranosylcytosine-(5′-5′)-3′-Cethynylcytidine as a cytotoxic drug. The result shown was an increased toxicity in the TEM1-expressing IMR32 tumor cell, compared to the control liposome (CitationMarty et al. 2006). Similarly, Lu and colleagues identified a scFv fragment binding specifically with the c-Met protein, the receptor of hepatocyte growth factor (HGF), that is aberrantly expressed and implicated in malignancy and human lung cancer. The anti-c-Met scFv conjugated with PEGylated liposomes carrying doxorubicin was proved to be an efficient DDS in tumor cells which induces the apoptotic effects. Moreover, better tumor accumulation of cytotoxic drugs and anticancer properties have been estimated through the delivery of anti-c-met immunoliposomes directed by scFv in a tumor xenograft model (CitationLu et al. 2011).
Angiogenesis plays an important role in tumor invasion and metastasis. Cancer cells can produce vascular endothelial growth factor (VEGF), which binds to the receptors on the surface of the endothelial cell, resulting in biological effects and tumor progression. The study of poly(lactic acid) poly(ethylene glycol) (PEG-PLA)-conjugated scFv against the VEGF-2 receptor which was loaded with As2O3 showed that scFv can significantly inhibit angiogenesis and also prevent the progression of the liver cancer xenograft (CitationXiangbao et al. 2014).
In another study, it has been demonstrated that hydroxyethyl chitosan modified with anti-human death domain 5 (DR5) scFv antibodies led to cell death in various types of tumor cells, and tumor growth suppression, both in vivo and in vitro (CitationYang et al. 2014).
In general, patients with Her2-overexpressing (Her2+) breast cancers do not have a certain prognosis due to the high resistance to chemotherapy. In recent years, for the delivery of small interfering RNA (siRNA) against polo-like kinase 1 gene (Plk1 or siPlk1) to Her2+ breast cancer through anti-Her2 single-chain variable fragment antibody (ScFvHer2), PEG-PLA nanoparticles along with siPlk1 encapsulation (ScFvHer2-NPsi Plk1) have been designed. Based on the current investigations, ScFvHer2-NPsi Plk1 is able to promote the cellular uptake, achieve efficient Plk1 silencing and increased cancer cell apoptosis, in comparison with non-targeted NPsiPlk1 in Her2+ breast cancer (CitationDou et al. 2014). It seems that scFv-targeted nanoparticles have great potential for a broad application in cancer therapy.
Conclusion
Nanoparticles applied in DDS are able to improve the therapeutic index of drugs which are used in cancer therapy. Various types of nanocarriers have been designed with different features, conjugating with drug molecules to accumulate such molecules at the site of action via passive and active targeting, to decrease the toxicity or other undesirable effects in healthy tissues, as well as to protect them from clearance or deletion in blood circulation. Single-chain fragment antibodies have been a subject of interest in novel target therapy, particularly in cancer. Also, over the past few years, an increasing demand for the application of these fragments is being observed.
Declaration of interest
The authors report no declarations of interest. The authors alone are responsible for the content and writing of the paper.
References
- Abou-Jawde R, Choueiri T, Alemany C, Mekhail T. 2003. An overview of targeted treatments in cancer. Clin Ther. 25:2121–2137.
- Adams GP, Schier R, McCall AM, Simmons HH, Horak EM, Alpaugh RK, et al. 2001. High affinity restricts the localization and tumor penetration of single-chain fv antibody molecules. Cancer Res. 61:4750–4755.
- Ahmad ZA, Yeap SK, Ali AM, Ho WY, Alitheen NBM, Hamid M. 2012. scFv antibody: principles and clinical application. Clin Dev Immunol. 2012:980250.
- Ajima K, Murakami T, Mizoguchi Y, Tsuchida K, Ichihashi T, Iijima S, Yudasaka M. 2008. Enhancement of in vivo anticancer effects of cisplatin by incorporation inside single-wall carbon nanohorns. Acs Nano. 2:2057–2064.
- Allen TM. 2002. Ligand-targeted therapeutics in anticancer therapy. Nat Rev Cancer. 2:750–763.
- Allen TM. 1994. Long-circulating (sterically stabilized) liposomes for targeted drug delivery. Trends Pharmacol Sci. 15:215–220.
- Amato G. 2010. Silica-encapsulated efficient and stable Si quantum dots with high biocompatibility. Nanoscale Res Lett. 5:1156–1160.
- Anand P, Kunnumakara AB, Sundaram C, Harikumar KB, Tharakan ST, Lai OS, et al. 2008. Cancer is a preventable disease that requires major lifestyle changes. Pharmaceutical Research. 25:2097–2116.
- Arruebo M, Fernández-Pacheco R, Ibarra MR, Santamaría J. 2007. Magnetic nanoparticles for drug delivery. Nano Today. 2:22–32.
- Beg S, Rizwan M, Sheikh AM, Hasnain MS, Anwer K, Kohli K. 2011. Advancement in carbon nanotubes: basics, biomedical applications and toxicity. J Pharm Pharmacol. 63:141–163.
- Benita S. 2005. Microencapsulation: methods and industrial applications. CRC Press.
- Bradbury AR, Sidhu S, D bel S, McCafferty J. 2011. Beyond natural antibodies: the power of in vitro display technologies. Nature biotechnology, 29, 245–254.
- Brannon-Peppas L, Blanchette JO. 2012. Nanoparticle and targeted systems for cancer therapy. Adv Drug Deliv Rev. 64:206–212.
- Bray F, Møller B. 2006. Predicting the future burden of cancer. Nat Rev Cancer. 6:63–74.
- Brennan FR, Shaw L, Wing MG, Robinson C. 2004. Preclinical safety testing of biotechnology-derived pharmaceuticals. Mol Biotechnol. 27:59–74.
- Cabral H, Kataoka K. 2014. Progress of drug-loaded polymeric micelles into clinical studies. Journal of Controlled Release, 190, 465–476.
- Caminade A-M, Laurent R, Majoral J-P. 2005. Characterization of dendrimers. Adv Drug Deliv Rev. 57:2130–2146.
- Carmeliet P, Jain RK. 2000. Angiogenesis in cancer and other diseases. Nature. 407:249–257.
- Carter P. 2001. Improving the efficacy of antibody-based cancer therapies. Nat Rev Cancer. 1:118–129.
- Cho K, Wang X, Nie S, Shin DM. 2008. Therapeutic nanoparticles for drug delivery in cancer. Clin Cancer Res. 14:1310–1316.
- Cole AJ, Yang VC, David AE. 2011. Cancer theranostics: the rise of targeted magnetic nanoparticles. Trends Biotechnol. 29:323–332.
- Conti M, Tazzari V, Baccini C, Pertici G, Serino LP, De Giorgi U. 2006. Anticancer drug delivery with nanoparticles. In Vivo. 20:697–701.
- Couvreur P, Vauthier C. 2006. Nanotechnology: intelligent design to treat complex disease. Pharm Res. 23:1417–1450.
- Daemen T, Hofstede G, Ten Kate MT, Bakker‐Woudenberg IA, Scherphof GL. 1995. Liposomal doxorubicin‐induced toxicity: depletion and impairment of phagocytic activity of liver macrophages. Int J Cancer. 61:716–721.
- Davis ME, Shin DM. 2008. Nanoparticle therapeutics: an emerging treatment modality for cancer. Nat Rev Drug Discov. 7:771–782.
- des Rieux A, Fievez V, Garinot M, Schneider Y-J, Préat V. 2006. Nanoparticles as potential oral delivery systems of proteins and vaccines: a mechanistic approach. J Control Release. 116:1–27.
- Di Pasqua AJ, Wallner S, Kerwood DJ, Dabrowiak JC. 2009. Adsorption of the PtII anticancer drug carboplatin by mesoporous silica. Chem Biodivers. 6:1343–1349.
- Dobrovolskaia MA, McNeil SE. 2007. Immunological properties of engineered nanomaterials. Nat Nanotechnol. 2:469–478.
- Dou S, Yang XZ, Xiong MH, Sun CY, Yao YD, Zhu YH, Wang J. 2014. ScFv‐decorated PEG‐PLA‐based nanoparticles for enhanced siRNA delivery to Her2 + breast cancer. Adv Healthc Mater. 3: 1792–1803.
- Douer D, Estey E, Santillana S, Bennett JM, Lopez-Bernstein G, Boehm K, Williams T. 2001. Treatment of newly diagnosed and relapsed acute promyelocytic leukemia with intravenous liposomal all-transretinoic acid. Blood. 97:73–80.
- Echeverría JC, Estella J, Barbería V, Musgo J, Garrido JJ. 2010. Synthesis and characterization of ultramicroporous silica xerogels. J Non-Cryst Solids. 356:378–382.
- Farokhzad OC, Langer R. 2009. Impact of nanotechnology on drug delivery. ACS Nano. 3:16–20.
- Ferrari M. 2005. Cancer nanotechnology: opportunities and challenges. Nat Rev Cancer. 5:161–171.
- Fidalgo A, Lopez TM, Ilharco LM. 2009. Wet sol–gel silica matrices as delivery devices for phenytoin. J Sol-Gel Sci Technol. 49:320–328.
- Foldvari M, Bagonluri M. 2008. Carbon nanotubes as functional excipients for nanomedicines: II. Drug delivery and biocompatibility issues. Nanomedicine NBM. 4:183–200.
- Gaillard PJ, Appeldoorn CC, Dorland R, Van Kregten J, Manca F, Vugts DJ, et al. 2014. Pharmacokinetics, brain delivery, and efficacy in brain tumorbearing mice of glutathione pegylated liposomal doxorubicin (2B3-101). PloS one. 9:e82331.
- Glennie MJ, van de Winkel JG. 2003. Renaissance of cancer therapeutic antibodies. Drug Discov Today. 8:503–510.
- Grassi-Schultheiss P, Heller F, Dobson J. 1997. Analysis of magnetic material in the human heart, spleen and liver. Biometals. 10:351–355.
- Guo S, Huang L. 2013. Nanoparticles containing insoluble drug for cancer therapy. Biotechnology advances.
- Hajdu SI. 2011. A note from history: landmarks in history of cancer, part 1. Cancer. 117:1097–1102.
- Haley B, Frenkel E. 2008. Nanoparticles for drug delivery in cancer treatment. In: Urologic Oncology: Seminars and original investigations. Elsevier: 57–64.
- Holliger P, Hudson PJ. 2005. Engineered antibody fragments and the rise of single domains. Nat Biotechnol. 23:1126–1136.
- Hu C-MJ, Aryal S, Zhang L. 2010. Nanoparticle-assisted combination therapies for effective cancer treatment. Therapeu Deliv. 1:323–334.
- Hudson PJ. 1998. Recombinant antibody fragments. Curr Opin Biotechnol. 9:395–402.
- Jain S, Hirst D, O’Sullivan J. 2014. Gold nanoparticles as novel agents for cancer therapy.
- Jemal A, Siegel R, Ward E, Hao Y, Xu J, Murray T, Thun MJ. 2008. Cancer statistics, 2008. CA Cancer J Clin. 58:71–96.
- Jia G, Wang H, Yan L, Wang X, Pei R, Yan T, Zhao Y, Guo X. 2005. Cytotoxicity of carbon nanomaterials: single-wall nanotube, multi-wall nanotube, and fullerene. Environ Sci Technol. 39:1378–1383.
- Kato Y, Seita T, Kuwabara T, Sugiyama Y. 1996. Kinetic analysis of receptor-mediated endocytosis (RME) of proteins and peptides: use of RME as a drug delivery system. J Controlled Release. 39:191–200.
- Köhler G, Milstein C. 1975. Continuous cultures of fused cells secreting antibody of predefined specificity. Nature. 256:495–497.
- Kovacevic A, Savic S, Vuleta G, Müller R, Keck C. 2011. Polyhydroxy surfactants for the formulation of lipid nanoparticles (SLN and NLC). effects on size, physical stability and particle matrix structure. Int J Pharm. 406:163–172.
- Kumari A, Yadav SK, Yadav SC. 2010. Biodegradable polymeric nanoparticles based drug delivery systems. Colloids Surf B: Biointerfaces. 75:1–18.
- Kyung OY, Grabinski CM, Schrand AM, Murdock RC, Wang W, Gu B, et al. 2009. Toxicity of amorphous silica nanoparticles in mouse keratinocytes. J Nanopart Res. 11:15–24.
- Leamon CP, Reddy JA. 2004. Folate-targeted chemotherapy. Adv Drug Deliv Rev. 56:1127–1141.
- Li Z, Su K, Cheng B, Deng Y. 2010. Organically modified MCM-type material preparation and its usage in controlled amoxicillin delivery. J Colloid Interface Sci. 342:607–613.
- Li C, Wallace S. 2008. Polymer-drug conjugates: recent development in clinical oncology. Advanced drug delivery reviews, 60, 886–898.
- Lin W, Huang Y-W, Zhou X-D, Ma Y. 2006. In vitro toxicity of silica nanoparticles in human lung cancer cells. Toxicol Appl Pharmacol. 217:252–259.
- Liu X, Sun J. 2010. Endothelial cells dysfunction induced by silica nanoparticles through oxidative stress via JNK/P53 and NF-κB pathways. Biomaterials. 31:8198–8209.
- Lu R-M, Chang Y-L, Chen M-S, Wu H-C. 2011. Single chain anti-c-Met antibody conjugated nanoparticles for in vivo tumor-targeted imaging and drug delivery. Biomaterials. 32:3265–3274.
- Luo X, Matranga C, Tan S, Alba N, Cui XT. 2011. Carbon nanotube nanoreservior for controlled release of anti-inflammatory dexamethasone. Biomaterials. 32:6316–6323.
- Maeda H. 2001. The enhanced permeability and retention (EPR) effect in tumor vasculature: the key role of tumor-selective macromolecular drug targeting. Adv Enzyme Regul. 41:189–207.
- Mamot C, Ritschard R, Wicki A, Stehle G, Dieterle T, Bubendorf L, et al. 2012. Tolerability, safety, pharmacokinetics, and efficacy of doxorubicin-loaded anti-EGFR immunoliposomes in advanced solid tumours: a phase 1 dose-escalation study. Lancet oncol. 13:1234–1241.
- Manchester M, Singh P. 2006. Virus-based nanoparticles (VNPs): platform technologies for diagnostic imaging. Adv Drug Deliv Rev. 58:1505–1522.
- Mansour AM, Drevs J, Esser N, Hamada FM, Badary OA, Unger C, et al. 2003. A new approach for the treatment of malignant melanoma: enhanced antitumor efficacy of an albumin-binding doxorubicin prodrug that is cleaved by matrix metalloproteinase 2. Cancer Res. 63:4062–4066.
- Marty C, Langer-Machova Z, Sigrist S, Schott H, Schwendener RA, Ballmer-Hofer K. 2006. Isolation and characterization of a scFv antibody specific for tumor endothelial marker 1 (TEM1), a new reagent for targeted tumor therapy. Cancer Lett. 235:298–308.
- Mao Y, Triantafillou G, Hertlein E, Towns W, Stefanovski M, Mo X, Jarjoura D, Phelps M, Marcucci G, Lee LJ. 2013. Milatuzumab-conjugated liposomes as targeted dexamethasone carriers for therapeutic delivery in CD74+ B-cell malignancies. Clinical Cancer Research, 19, 347–356.
- Matsumura Y, Gotoh M, Muro K, Yamada Y, Shirao K, Shimada Y, et al. 2004. Phase I and pharmacokinetic study of MCC-465, a doxorubicin (DXR) encapsulated in PEG immunoliposome, in patients with metastatic stomach cancer. Ann Oncol. 15:517–525.
- Maynard J, Georgiou G. 2000. Antibody engineering. Annu Rev Biomed Eng. 2:339–376.
- Mellet CO, Fern ndez JMG, Benito JM. 2011. Cyclodextrin-based gene delivery systems. Chemical Society Reviews, 40, 1586–1608.
- Miele E, Spinelli GP, Miele E, Tomao F, Tomao S. 2009. Albumin-bound formulation of paclitaxel (Abraxane ABI-007) in the treatment of breast cancer. International journal of nanomedicine, 4, 99.
- Miller A, Hoogstraten B, Staquet M, Winkler A. 1981. Reporting Results of cancer treatment. Cancer. 47:207–214.
- Monnier PP, Vigouroux RJ, Tassew NG. 2013. In vivo applications of single chain Fv (variable domain)(scFv) fragments. Antibodies. 2:193–208.
- Murakami T, Sawada H, Tamura G, Yudasaka M, Iijima S, Tsuchida K. 2008. Water-dispersed single-wall carbon nanohorns as drug carriers for local cancer chemotherapy. Nanomedicine (Lond). 3:453–63.
- Naqvi S, Samim M, Abdin M, Ahmed FJ, Maitra A, Prashant C, Dinda AK. 2010. Concentration-dependent toxicity of iron oxide nanoparticles mediated by increased oxidative stress. Int J Nanomedicine. 5:983–989.
- Nellis DF, Giardina SL, Janini GM, Shenoy SR, Marks JD, Tsai R, et al. 2005. Preclinical Manufacture of Anti‐HER2 Liposome‐Inserting, scFv‐PEG‐Lipid Conjugate. 2. Conjugate Micelle Identity, Purity, Stability, and Potency Analysis. Biotechnol prog. 21:221–232.
- Nevozhay D, Kańska U, Budzyńska R, Boratyński J. 2006. [Current status of research on conjugates and related drug delivery systems in the treatment of cancer and other diseases]. Postepy Hig Med Dosw (Online). 61:350–360.
- Nomura T, Saikawa A, Morita S, Yamashita F, Honda K, Takakura Y, Hashida M. 1998. Pharmacokinetic characteristics and therapeutic effects of mitomycin C-dextran conjugates after intratumoural injection. J Control Release. 52:239–252.
- O’Brien S, Schiller G, Lister J, Damon L, Goldberg S, Aulitzky W, et al. 2013. High-dose vincristine sulfate liposome injection for advanced, relapsed, and refractory adult Philadelphia chromosome–negative acute lymphoblastic leukemia. Journal of clinical oncology, 31, 676–683.
- Owens DE III, Peppas NA. 2006. Opsonization, biodistribution, and pharmacokinetics of polymeric nanoparticles. Int J Pharm. 307:93–102.
- Park E-J, Park K. 2009. Oxidative stress and pro-inflammatory responses induced by silica nanoparticles in vivo and in vitro. Toxicol Lett. 184:18–25.
- Park K, Lee S, Kang E, Kim K, Choi K, Kwon IC. 2009. New generation of multifunctional nanoparticles for cancer imaging and therapy. Adv Funct Mater. 19:1553–1566.
- Pastan I, Hassan R, Fitzgerald DJ, Kreitman RJ. 2006. Immunotoxin therapy of cancer. Nat Rev Cancer. 6:559–565.
- Patri AK, Kukowska-Latallo JF, Baker Jr. 2005. Targeted drug delivery with dendrimers: comparison of the release kinetics of covalently conjugated drug and non-covalent drug inclusion complex. Adv Drug Deliv Rev. 57:2203–2214.
- Pavlinkova G, Colcher D, Booth BJ, Goel A, Wittel UA, Batra SK. 2001. Effects of humanization and gene shuffling on immunogenicity and antigen binding of anti‐tag‐72 single‐chain Fvs. Int J Cancer. 94:717–726.
- Peer D, Karp JM, Hong S, Farokhzad OC, Margalit R, Langer R. 2007a. Nanocarriers as an emerging platform for cancer therapy. Nat Nanotechnol. 2:751–760.
- Peer D, Zhu P, Carman CV, Lieberman J, Shimaoka M. 2007b. Selective gene silencing in activated leukocytes by targeting siRNAs to the integrin lymphocyte function-associated antigen-1. Proc Natl Acad Sci. 104:4095–4100.
- Pelicano H, Martin D, Xu R, Huang P. 2006. Glycolysis inhibition for anticancer treatment. Oncogene. 25:4633–4646.
- Perez E. 2005. American Pharmaceutical Partners announces presentation of Abraxane survival data. In: 22nd annual Miami Breast Cancer Conference; Miami: FL.
- Petrelli NJ, Winer EP, Brahmer J, Dubey S, Smith S, Thomas C, et al. 2009. Clinical Cancer Advances 2009: major research advances in cancer treatment, prevention, and screening—a report from the American Society of Clinical Oncology. J Clin Oncol. 27:6052–6069.
- Pillai G, Ceballos-Coronel ML. 2013. Science and technology of the emerging nanomedicines in cancer therapy: A primer for physicians and pharmacists. SAGE Open Medicine, 1, 2050312113513759.
- Popovici R, Seftel E, Mihai G, Popovici E, Voicu V. 2011. Controlled drug delivery system based on ordered mesoporous silica matrices of captopril as angiotensin‐converting enzyme inhibitor drug. J Pharm Sci. 100:704–714.
- Pucca MB, Bertolini TB, Barbosa JE, Galina SVR, Porto GS. 2011. Therapeutic monoclonal antibodies: scFv patents as a marker of a new class of potential biopharmaceuticals. Braz J Pharm Sci. 47:31–38.
- Qian ZM, Li H, Sun H, Ho K. 2002. Targeted drug delivery via the transferrin receptor-mediated endocytosis pathway. Pharmacol Rev. 54:561–587.
- Riggio C, Pagni E, Raffa V, Cuschieri A. 2011. Nano-oncology: clinical application for cancer therapy and future perspectives. Journal of Nanomaterials, 2011, 17.
- Rivera, E. 2003. Liposomal anthracyclines in metastatic breast cancer: clinical update. The oncologist, 8, 3–9.
- Robinson MK, Doss M, Shaller C, Narayanan D, Marks JD, Adler LP, et al. 2005. Quantitative immuno-positron emission tomography imaging of HER2-positive tumor xenografts with an iodine-124 labeled anti-HER2 diabody. Cancer Res. 65:1471–1478.
- Ross JS, Schenkein DP, Pietrusko R, Rolfe M, Linette GP, Stec J, et al. 2004. Targeted therapies for cancer 2004. Am J Clin Pathol. 122: 598–609.
- Santos Giuberti CD, de Oliveira Reis EC, Ribeiro Rocha TG, Leite EA, Lacerda RG, Ramaldes GA, de Oliveira MC. 2011. Study of the pilot production process of long-circulating and pH-sensitive liposomes containing cisplatin. J Liposome Res. 21:60–69.
- Sapra P, Allen TM. 2002. Internalizing antibodies are necessary for improved therapeutic efficacy of antibody-targeted liposomal drugs. Cancer Res. 62:7190–7194.
- Senzer NN, Matsuno K, Yamagata N, Fujisawa T, Wasserman E, Sutherland W, Sharma S, Phan A. 2009. Abstract C36: MBP-426, a novel liposome-encapsulated oxaliplatin, in combination with 5-FU/leucovorin (LV): Phase I results of a Phase I/II study in gastro-esophageal adenocarcinoma, with pharmacokinetics. Molecular Cancer Therapeutics, 8, C36–C36.
- Senzer N, Nemunaitis J, Nemunaitis D, Bedell C, Edelman G, Barve M, et al. 2013. Phase I study of a systemically delivered p53 nanoparticle in advanced solid tumors. Mol Ther. 21:1096–1103.
- Shenoy DB, Amiji MM. 2005. Poly (ethylene oxide)-modified poly (ɛ-caprolactone) nanoparticles for targeted delivery of tamoxifen in breast cancer. Int J Pharma. 293:261–270.
- Shiba K, Yudasaka M, Iijima S. 2006. [Carbon nanohorns as a novel drug carrier]. Nihon Rinsho. 64:239–246.
- Sidhu SS, Fellouse FA. 2006. Synthetic therapeutic antibodies. Nature chemical biology, 2, 682–688.
- Silverman LB, Supko JG, Stevenson KE, Woodward C, Vrooman LM, Neuberg DS. et al. 2010. Intravenous PEG-asparaginase during remission induction in children and adolescents with newly diagnosed acute lymphoblastic leukemia. Blood, 115, 1351–1353.
- Singh P, Destito G, Schneemann A, Manchester M. 2006. Canine parvovirus-like particles, a novel nanomaterial for tumor targeting. J Nanobiotechnology. 4:1–11.
- Singh P, Gupta U, Asthana A, Jain NK. 2008. Folate and folate− PEG− PAMAM dendrimers: synthesis, characterization, and targeted anticancer drug delivery potential in tumor bearing mice. Bioconjug Chem. 19:2239–2252.
- Sinha R, Kim GJ, Nie S, Shin DM. 2006. Nanotechnology in cancer therapeutics: bioconjugated nanoparticles for drug delivery. Mol Cancer Ther. 5:1909–1917.
- Stewart S, Jablonowski H, Goebel FD, Arasteh K, Spittle M, Rios A, et al. 1998. Randomized comparative trial of pegylated liposomal doxorubicin versus bleomycin and vincristine in the treatment of AIDS-related Kaposi’s sarcoma. International Pegylated Liposomal Doxorubicin Study Group. Journal of Clinical Oncology, 16, 683–691.
- Sun C, Lee JS, Zhang M. 2008. Magnetic nanoparticles in MR imaging and drug delivery. Advanced Drug Deliv Rev. 60:1252–1265.
- Sundaresan G, Yazaki PJ, Shively JE, Finn RD, Larson SM, Raubitschek AA, et al. 2003. 124I-labeled engineered anti-CEA minibodies and diabodies allow high-contrast, antigen-specific small-animal PET imaging of xenografts in athymic mice. J Nucl Med. 44:1962–1969.
- Sunderland CJ, Steiert M, Talmadge JE, Derfus AM, Barry SE. 2006. Targeted nanoparticles for detecting and treating cancer. Drug Develop Res. 67:70–93.
- Suzawa T, Nagamura S, Saito H, Ohta S, Hanai N, Kanazawa J, et al. 2002. Enhanced tumor cell selectivity of adriamycin-monoclonal antibody conjugate via a poly (ethylene glycol)-based cleavable linker. J Controlled Release. 79:229–242.
- Svenson S, Tomalia DA. 2012. Dendrimers in biomedical applications—reflections on the field. Adv Drug Deliv Rev. 64:102–115.
- Swami A, Shi J, Gadde S, Votruba AR, Kolishetti N, Farokhzad OC. 2012. Nanoparticles for targeted and temporally controlled drug delivery. In: Multifunctional Nanoparticles for Drug Delivery Applications. Springer, pp. 9–29.
- Tassew NG, Charish J, Chestopalova L, Monnier PP. 2009. Sustained in vivo inhibition of protein domains using single-chain Fv recombinant antibodies and its application to dissect RGMa activity on axonal outgrowth. The Journal of Neuroscience, 29, 1126–1131.
- Torchilin V. 2008. Antibody-modified liposomes for cancer chemotherapy. Expert Opin Drug Deliv.2008. 5:1003–1025.
- Torchilin VP. 2005. Recent advances with liposomes as pharmaceutical carriers. Nat Rev Drug Discov. 4:145–160.
- Veiseh O, Gunn JW, Zhang M. 2010. Design and fabrication of magnetic nanoparticles for targeted drug delivery and imaging. Adv Drug Deliv Rev. 62:284–304.
- Visaria R, Bischof JC, Loren M, Williams B, Ebbini E, Paciotti G, et al. 2007. Nanotherapeutics for enhancing thermal therapy of cancer. International Journal of Hyperthermia, 23, 501–511.
- Wan L, Zhu S, Zhu J, Yang H, Li S, Li Y, et al. 2013. Production and characterization of a CD25-specific scFv-Fc antibody secreted from Pichia pastoris. Appl Microbiol Biotechnol. 97:3855–3863.
- Wang AZ, Gu F, Zhang L, Chan JM, Radovic-Moreno A, Shaikh MR, Farokhzad OC. 2008. Biofunctionalized targeted nanoparticles for therapeutic applications. Expert Opin Biol Ther.2008. 8:1063–1070.
- Weinberg WC, Frazier-Jessen MR, Wu WJ, Weir A, Hartsough M, Keegan P, Fuchs C. 2005. Development and regulation of monoclonal antibody products: challenges and opportunities. Cancer Metastasis Rev. 24:569–584.
- Wilczewska AZ, Niemirowicz K, Markiewicz KH, Car H. 2012. Nanoparticles as drug delivery systems. Pharmacol Rep. 64:1020–1037.
- Wissing S, Kayser O, Müller R. 2004. Solid lipid nanoparticles for parenteral drug delivery. Adv Drug Deliv Rev. 56:1257–1272.
- Xiangbao Y, Linquan W, Mingwen H, Fan Z, Kai W, Xin Y, et al. 2014. Humanized anti-VEGFR-2 ScFv-As2O3-stealth nanoparticles, an antibody conjugate with potent and selective anti-hepatocellular carcinoma activity. Biomed Pharmacother. 68: 597–602.
- Yang J, Huang X, Luo F, Cheng X, Cheng L, Liu B, Chen L, Hu R, Shi C, Zhuang G. 2014. Preparation and functional studies of hydroxyethyl chitosan nanoparticles loaded with anti-human death receptor 5 single-chain antibody. OncoTargets Ther. 7:779.
- Yang L, Mao H, Wang YA, Cao Z, Peng X, Wang X, et al. 2009. Single chain epidermal growth factor receptor antibody conjugated nanoparticles for in vivo tumor targeting and imaging. Small. 5: 235–243.
- Yatvin M, Kreutz W, Horwitz B, Shinitzky M. 1980. pH-sensitive liposomes. possible clinical implications. Science. 210:1253–1255.
- Yellepeddi VK, Kumar A, Palakurthi S. 2009. Surface modified poly (amido) amine dendrimers as diverse nanomolecules for biomedical applications. Expert Opin Drug Deliv.2009. 6:835–850.
- Yockman JW, Maheshwari A, Han S-O, Kim SW. 2003. Tumor regression by repeated intratumoral delivery of water soluble lipopolymers/p2CMVmIL-12 complexes. J Control Release. 87:177–186.
- Zhang B, Chen Q, Tang H, Xie Q, Ma M, Tan L, et al. 2010. Characterization of and biomolecule immobilization on the biocompatible multi-walled carbon nanotubes generated by functionalization with polyamidoamine dendrimers. Colloids Surf B Biointerfaces. 80:18–25.
- Zhou Y, Drummond DC, Zou H, Hayes ME, Adams GP, Kirpotin DB, Marks JD. 2007. Impact of single-chain Fv antibody fragment affinity on nanoparticle targeting of epidermal growth factor receptor-expressing tumor cells. J Mol Biol. 371:934–947.