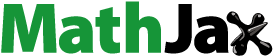
Abstract
The aim of this research was to prepare and optimize calcium carbonate (CaCO3) nanoparticles as carriers for gentamicin sulfate. A chemical precipitation method was used to prepare the gentamicin sulfate-loaded CaCO3 nanoparticles. A 3-factor, 3-level Box–Behnken design was used for the optimization procedure, with the molar ratio of CaCl2: Na2CO3 (X1), the concentration of drug (X2), and the speed of homogenization (X3) as the independent variables. The particle size and entrapment efficiency were considered as response variables. Mathematical equations and response surface plots were used, along with the counter plots, to relate the dependent and independent variables. The results indicated that the speed of homogenization was the main variable contributing to particle size and entrapment efficiency. The combined effect of all three independent variables was also evaluated. Using the response optimization design, the optimized Xl–X3 levels were predicted. An optimized formulation was then prepared according to these levels, resulting in a particle size of 80.23 nm and an entrapment efficiency of 30.80%. It was concluded that the chemical precipitation technique, together with the Box–Behnken experimental design methodology, could be successfully used to optimize the formulation of drug-incorporated calcium carbonate nanoparticles.
Introduction
Pharmaceutical nanoparticles are fine drug-loaded particulates with sizes ranging between 10–1000 nm, and properties that are different from those of their original materials (CitationAdibkia et al. 2007, CitationLu et al. 2009, CitationHallan et al. 2014, CitationDaraee et al. 2014). These nanosystems can advantageously improve the therapeutic efficacy of a drug by enhancing its bioavailability (CitationMohammadi et al. 2011, CitationLotfipour et al. 2011, CitationAdibkia et al. 2012, CitationChaudhary et al. 2015) and serum stability (CitationBowman and Leong 2006).
Gentamicin, an aminoglycoside, inhibits protein synthesis by binding to the 30 S ribosomal subunit of bacterial cells (CitationYoshizawa et al. 1998). Similar to other aminoglycosides, gentamicin has a relatively short half-life and low bioavailability, and may result in side effects such as nephrotoxicity (CitationTange et al. 1995). Different kinds of gentamicin-loaded nanoparticles have been previously prepared using some polymers such as poly(lactide-co-glycolide) (PLGA) (CitationAbdelghany et al. 2012), chitosan (CitationJi et al. 2011), and caroboxymethyl dextran-b-poly(ethylene glycols) (CitationSoliman et al. 2010).
Bone infectious disease—known as osteomyelitis—is initiated by a variety of pathogens, most commonly Staphylococcus aureus. This pathogen can penetrate endothelial, epithelial, and osteoblastic cells (CitationAlmeida et al. 1996, CitationWesson et al. 1998) and provide a reservoir of bacteria in the infected area. Considering this, targeting the pathogen using the antibiotic may be more important for treating chronic bone infection than physically eliminating only colonized pathogens (CitationBost et al. 1999). Local application of antibiotics could eradicate the microorganisms more effectively, without systemic toxicity (CitationRichards et al. 2006).
Therapeutic applications of calcium carbonate (CaCO3) nanoparticles in modern medical systems have attracted the attention of researchers, due to their great potential and capabilities. This material is low-cost, biocompatible, safe, and accessible. According to a research by Combes et al., CaCO3-based cements showed no cytotoxic effect on the osteoprogenitor cells of human bone marrow (CitationCombes et al. 2006). The use of CaCO3 nanoparticles as a controlled release system for different drugs has been reported by different investigators (CitationQian et al. 2011, CitationWang et al. 2006). According to research studies, CaCO3 materials can be suitable to increase the rate of resorption of biomedical cement and initiate its replacement by bone tissue (CitationFujita et al. 1991). Therefore, a dual therapeutic system (drug release and bone substitution) could be obtained by development of the antibiotic-loaded resorbable bone-filling materials using CaCO3. A direct contact between the bone and calcite CaCO3 without interposition of soft tissue at the interface has been reported as well (CitationOhgushi et al. 1992). Aragonite CaCO3 has also been used for designing anticancer drug carriers and scaffolds for bone repair and tissue engineering (CitationIslam et al. 2012).
Response surface design is a collection of statistical procedures to analyze the effects of several independent variables. It mostly involves the central composite design and the Box–Behnken design (CitationGhanbarzadeh et al. 2014, CitationThompson 1982). Compared to the central composite design, Box–Behnken designs contain fewer design points, and they can be less expensive to implement than central composite designs with the same number of factors (CitationThompson 1982).
The optimization of preparation methods for drug-loaded CaCO3 nanoparticles to be used as biodegradable and biocompatible material was investigated in this study. Considering the great potential of CaCO3 in drug delivery, its bone-related advantages, as well as the benefits of nanoparticle-based drug delivery systems, this study may be extended to the delivery of gentamicin sulfate into bone infections such as osteomyelitis. The effect of three independent variables on the entrapment efficiency and particle size of gentamicin sulfate–CaCO3 nanoparticles, that is, the molar ratio of CaCl2:Na2CO3, drug concentration, and the speed of homogenization, was evaluated using a 3-level, 3-factor Box–Behnken experimental design.
Materials and methods
Materials
Gentamicin sulfate was supplied by Zahravi Co (Tabriz, Iran). Calcium chloride, sodium carbonate, and ninhydrin were purchased from Merck Company (Darmstadt, Germany).
Preparation of gentamicin sulfate–CaCO3 nanoparticles
The chemical precipitation method was used to entrap gentamicin sulfate into CaCO3 nanoparticles (CitationUeno et al. 2005). Concentrations were selected based on previous studies for the development of CaCO3 nanoparticles (CitationUeno et al. 2005, CitationQian et al. 2011, CitationHaruta et al. 2003) (). To prepare the gentamicin sulfate–CaCO3 nanoparticles by the precipitation method, 100 μl of aqueous solution of the drug and 100 μl of aqueous solution of CaCl2 were moderately mixed for 20 min, followed by the addition of 100 μl of aqueous solution of Na2CO3, and the solutions were stirred together vigorously for 15 min. Next, 5 ml of distilled water was added into the system and the large particles were discarded. The final suspension was centrifuged (Eppendorf AG 5810R, Germany) at 12,000 rpm for 5 min, to separate the nanoparticles. Based on the experimental design, the responses for fifteen formulations were obtained ().
Table I. Factor-setting for the experimental design.
Table II. Particle size (PS) and percent entrapment efficiency (EE %) values for fifteen formulations.
Characterization of the nanoparticles
Particle size
The values for mean particle size for all the formulations were determined by using a particle size analyzer (SALD 2101, Shimadzu, Japan). All measurements were performed in triplicate. Mean particle sizes for the samples are shown in .
Entrapment efficiency
After dissolving 100 mg of the prepared gentamicin sulfate–CaCO3 nanoparticles in EDTA 0.5 M (pH 7.5) (CitationUeno et al. 2005, CitationQian et al. 2011), the amount of gentamicin loaded was detected by the colorimetric method (using ninhydrin as the reagent, in 1.25% w/v aqueous ninhydrin solution) using the UV spectrophotometer (UV1800 Shimadzu, Japan) (CitationFrutos et al. 2000). A previously prepared standard curve (linear in the range of 5–80 mcg/ml, y = 0.0121x + 0.0154, R2 = 0.9992) was used to determine the entrapment efficiency, based on the following equation:
Each set of data for entrapment efficiency was reported as an average of three calculations ().
Experimental design
A 3-factor, 3-level Box–Behnken design was utilized in the present study (). The molar ratio of CaCl2:Na2CO3 (X1), the concentration of drug (X2), and the speed of homogenization (X3) were chosen as the independent variables. The size of the nanoparticles and their entrapment efficiency were considered as dependent variables. The experimental design set for all fifteen formulations predicted is shown in . The effects of the independent variables on responses were investigated by means of mathematical equations, counter plots, and surface plots. Analysis of variance (ANOVA) was used to evaluate the results, and the final equations were shortened to include only significant parameters (P < 0.05). The response optimization approach was also applied to obtain gentamicin-loaded CaCO3 nanoparticles with desired particle size and entrapment efficiency. Response optimization helps to identify the combination of variables to optimize a single response or a set of responses.
Results and discussion
Effect of the factors on particle size
The particle size is one of the most important parameters that is strongly considered in the development of nanoparticulate drug delivery systems. The particle sizes of the different batches of nanoparticles prepared in this research were in the range of 80.0–300.12 nm. The relationship between the independent variables and particle size was clarified using Equation 1, contour plots, and response surface plots. Equation 1 shows the relationship between the different variables and particle size as the first response:
where PS denotes the particle size, and X1, X2, and X3 represent the molar ratios of CaCl2:Na2CO3, the drug concentration, and the homogenization speed respectively.
The equation clearly indicates that the particle size values are strongly dependent on the independent variables selected. The particle size is mostly affected by the homogenization speed (X3). The negative sign for coefficient of homogenization speed (X3) indicates that the particle size decreases noticeably following an increase of homogenization speed (p = 0.0001).
The graphs, which evaluate the effects of independent variables on the particle size, are shown in . As seen in , an increase in the homogenization speed from 2000 rpm up to 12,000 rpm caused a decrease in the particle size from 300.12 nm to 80.0 nm. Enhancing the homogenization speed increased both mechanical and hydraulic shear, which in turn could have effectively reduced the particle size (CitationNakashima et al. 2009). In a research study by Ueno et al., the particle size of betamethasone phosphate-loaded CaCO3 nanoparticles was decreased when the stirring speed was multiplied (CitationUeno et al. 2005). Similar observations were also reported by other researchers (CitationMaa and Hsu 1996).
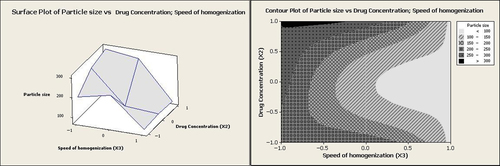
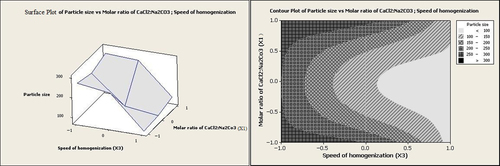
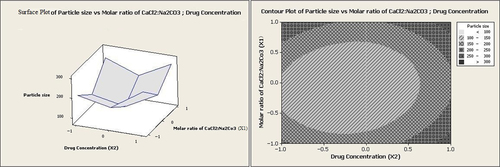
The effect of drug concentration is also illustrated in . The result shows that the size of the particles was enlarged when the drug concentration was enhanced. As reported in previous research studies, the viscosity of the media is enhanced by increasing the drug concentration, and the effect of viscosity on the particle size is obviously in contradiction to the effect of shear stress (CitationMasson et al. 2011, CitationNoori Koopaei et al. 2014, CitationKheradmandnia et al. 2010).
The ratio of Ca2+:CO32− has been reported to affect the particle size significantly (CitationChen et al. 2011). Nevertheless, in the present research, the molar ratio of CaCl2:Na2CO3 showed no reasonable effect on the particle size. However, according to both graphs in and , in addition to other factors such as homogenization speed and drug concentration, the minimum particle sizes were obtained at the intermediate molar ratios of CaCl2:Na2CO3.
Effect of the variables on entrapment efficiency
The entrapment efficiency for all the formulations was in the range of 20.0–42.1% ().
Where EE stands for entrapment efficiency
According to Equation 2 and , homogenization speed had the effect of lowering entrapment efficiency. Considering a number of reports, this effect could be attributed to drug diffusion out of the particles during the size reduction at the high homogenization speeds (CitationYoncheva et al. 2003, CitationPatel et al. 2013). Conversely, the drug concentration had a positive effect on entrapment efficiency. At low homogenization speeds, increasing the drug concentration from 1 g/dl to 5 g/dl led to improvement in the entrapment efficiency from 20.0% to 42.1% (). As revealed previously, an increase in the drug concentration leads to enhanced viscosity of the reaction media, thereby decreasing the shear stress. A decrease in the shear stress could lengthen the diffusion pathway, which in turn may cause an increase in the drug content (CitationGörner et al. 1999).
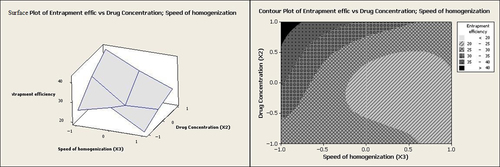
It can be seen from Equation 2 that the molar ratio of CaCl2:Na2CO3 (X1) had no significant effect on entrapment efficiency individually (p > 0.05). However, at low homogenization speeds and high values of the molar ratio of CaCl2:Na2CO3, entrapment efficiency was increased to levels above 35% (as illustrated in and ).
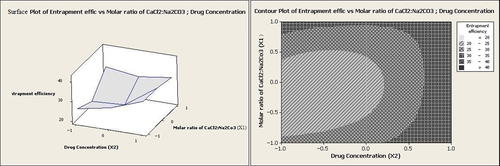
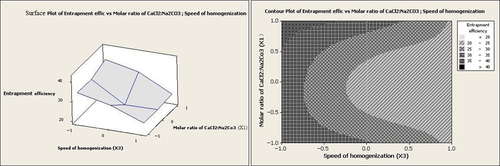
The correlation coefficients (R2) and adjusted R2 were calculated to be 0.95 and 0.87 for YPS, and 0.94 and 0.84 for YEE% respectively. The lack of fit was non-significant for YPS (p = 0.213) and YEE% (p = 0.412), representing that the models could properly explain the variability of the observed responses.
Response optimization of the nanoparticles
The optimization plot with appropriate particle size and acceptable entrapment efficiency is shown in . Coded units for the optimum point were defined as − 0.52, 0.89, and 1, which gives the values of 2:1.25, 4.89 g/dl, and 12,000 rpm for the molar ratio of CaCl2:Na2CO3, concentration of drug and the homogenization speed, respectively. Drug entrapment efficiency and particle size for the optimized point were predicted as 26.49% and 90.12 nm respectively.

Validation of the model
To evaluate the validation of the model, three more experiments were carried out in duplicate as a test set. The percentage error (PE) between calculated and observed values for each response was calculated by means of Equation 3. Details of the three formulations as test sets are summarized in the .
Table III. Percent error (PE) and mean percent error (MPE) between calculated and observed values for each response.
Consequently, optimized formulations were prepared according to the optimized point (particle size: 90.12 nm, entrapment efficiency: 26.49), and the PE between observed and calculated amounts was determined. Particle size and entrapment efficiency for optimized formulation were 80.23 nm (PE = 12.32%) and 30.80% (PE = 13.99%) respectively, which are considered to be acceptable (CitationActon 2012). According to our results, the observed values were close to the calculated ones, and hence the fit of the model was confirmed. Particle size distribution for the optimized formulation actually prepared is shown in .
Conclusion
Particle size and entrapment efficiency are important characteristics to be considered in the formulation of nanoparticles. The present study focused on the preparation of gentamicin sulfate–CaCO3 nanoparticles using the Box–Behnken statistical method. The optimized formulation was obtained with a relatively limited number of experimental runs, using the proper experimental design and an optimization approach. The combined effect of all factors considered led to the development of an optimized formulation, with a drug entrapment efficiency of 30.80% and a particle size of 80.23 nm. It was concluded that the chemical precipitation technique could be used along with the Box–Behnken experimental design methodology to determine the significant variables and optimum conditions for preparation of drug-incorporated calcium carbonate nanoparticles.
Acknowledgement
The authors would like to thank the Research Vice Chancellor of Tabriz University of Medical Sciences, for financial support provided for the study. This article is a part of a thesis (No. 96) submitted for the PhD degree in the Faculty of Pharmacy, Tabriz University of Medical Sciences.
Declaration of interest
The authors report no declarations of interest. The authors alone are responsible for the content and writing of the paper.
References
- Abdelghany SM, Quinn DJ, Ingram RJ, Gilmore BF, Donnelly RF, Taggart CC, Scott CJ. 2012. Gentamicin-loaded nanoparticles show improved antimicrobial effects towards Pseudomonas aeruginosa infection. Int J Nanomed. 7:4053–4063.
- Acton QA. 2012. Immunosuppressive Agents: Advances in Research and Application ,Atlanta, Georgia: ScholarlyBrief, Scholarly Editions.
- Adibkia K, Alaei-Beirami M, Barzegar-Jalali M, Mohammadi G, Ardestani MS. 2012. Evaluation and optimization of factors affecting novel diclofenac sodium-eudragit RS100 nanoparticles. African J Pharm Pharmacol. 6:941–947.
- Adibkia K, Shadbad MRS, Nokhodchi A, Javadzedeh A, Barzegar-Jalali M, Barar J, et al. 2007. Piroxicam nanoparticles for ocular delivery: physicochemical characterization and implementation in endotoxin-induced uveitis. J Drug Target. 15:407–416.
- Almeida RA, Matthews KR, Cifrian E, Guidry AJ, Oliver SP. 1996. Staphylococcus aureus Invasion of Bovine Mammary Epithelial Cells. J Dairy Sci. 79:1021–1026.
- Bost KL, Ramp WK, Nicholson NC, Bento JL, Marriott I, Hudson MC. 1999. Staphylococcus aureus infection of mouse or human osteoblasts induces high levels of interleukin-6 and interleukin-12 production. J Infect Dis. 180:1912–1920.
- Bowman K, Leong KW. 2006. Chitosan nanoparticles for oral drug and gene delivery. Int J Nanomed. 1:117–128.
- Chaudhary S, Garg T, Rath G, Murthy RR, Goyal AK. 2015. Enhancing the bioavailability of mebendazole by integrating the principles solid dispersion and nanocrystal techniques, for safe and effective management of human echinococcosis. Artif Cells Nanomed Biotechnol. 1–6.
- Chen S, Li F, Zhuo R-X, Cheng S-X. 2011. Efficient non-viral gene delivery mediated by nanostructured calcium carbonate in solution-based transfection and solid-phase transfection. Mol BioSys. 7:2841–2847.
- Combes C, Bareille R, Rey C. 2006. Calcium carbonate–calcium phosphate mixed cement compositions for bone reconstruction. J Biomed Mat Res A. 79:318–328.
- Daraee H, Eatemadi A, Abbasi E, Fekri Aval S, Kouhi M, Akbarzadeh A. 2014. Application of gold nanoparticles in biomedical and drug delivery. Artif Cells Nanomed Biotechnol.1–13.
- Frutos P, Torrado S, Perez-Lorenzo M, Frutos G. 2000. A validated quantitative colorimetric assay for gentamicin. J Pharm Biomed Anal. 21:1149–1159.
- Fujita Y, Yamamuro T, Nakamura T, Kotani S, Ohtsuki C, Kokubo T. 1991. The bonding behavior of calcite to bone. J Biomed Mater Res. 25:991–903.
- Ghanbarzadeh S, Khorrami A, Arami S. 2014. Preparation of optimized Naproxen nano liposomes using response surface methodology. J Pharm Inv. 44:33–39.
- Görner T, Gref R, Michenot D, Sommer F, Tran M, Dellacherie E. 1999. Lidocaine-loaded biodegradable nanospheres, Optimization of the drug incorporation into the polymer matrix. J Controll Release. 57:259–268.
- Hallan SS, Kaur P, Kaur V, Mishra N, Vaidya B. 2014. Lipid polymer hybrid as emerging tool in nanocarriers for oral drug delivery. Artif Cells Nanomed Biotechnol. 1–16.
- Haruta S, Hanafusa T, Fukase H, Miyajima H, Oki T. 2003. An effective absorption behavior of insulin for diabetic treatment following intranasal delivery using porous spherical calcium carbonate in monkeys and healthy human volunteers. Diabetes Technol Therap. 5:1–9.
- Islam KN, Zuki A, Ali M, Hussein MZB, Noordin M, Loqman M, et al. 2012. Facile synthesis of calcium carbonate nanoparticles from cockle shells. J Nanomat. 2012:1687–1610.
- Ji J, Hao S, Wu D, Huang R, Xu Y. 2011. Preparation, characterization and in vitro release of chitosan nanoparticles loaded with gentamicin and salicylic acid. Carbohydr Polym. 85:803–808.
- Kheradmandnia S, Vasheghani-Farahani E, Nosrati M, Atyabi F. 2010. Preparation and characterization of ketoprofen-loaded solid lipid nanoparticles made from beeswax and carnauba wax. Nanomedicine. 6:753–759.
- Lotfipour F, Hallaj-Nezhadi S, Valizadeh H, Dastmalchi S, Baradaran B, Jalali MB, Dobakhti F. 2011. Preparation of chitosan-plasmid DNA nanoparticles encoding interleukin-12 and their expression in CT-26 colon carcinoma cells. J Pharm Pharm Sci. 14:181–195.
- Lu E, Franzblau S, Onyuksel H, Popescu C. 2009. Preparation of aminoglycoside-loaded chitosan nanoparticles using dextran sulphate as a counterion. J Microencapsul. 26:346–354.
- Maa Y-F, Hsu C. 1996. Liquid-liquid emulsification by rotor/stator homogenization. J Control Release. 38:219–228.
- Masson LM, Rosenthal A, Calado V, Deliza R, Tashima L. 2011. Effect of ultra-high pressure homogenization on viscosity and shear stress of fermented dairy beverage. LWT-Food Sci Technol. 44:495–501.
- Mohammadi G, Nokhodchi A, Barzegar-Jalali M, Lotfipour F, Adibkia K, Ehyaei N, Valizadeh H. 2011. Physicochemical and anti-bacterial performance characterization of clarithromycin nanoparticles as colloidal drug delivery system. Colloid Surf B Biointerfaces. 88: 39–44.
- Nakashima S, Yoshie M, Sano H, Bahar A. 2009. Effect of a test dentifrice containing nano-sized calcium carbonate on remineralization of enamel lesions in vitro. J Oral Sci. 51:69–77.
- Noori Koopaei M, Khoshayand MR, Mostafavi SH, Amini M, Khorramizadeh MR, Jeddi Tehrani M, et al. 2014. Docetaxel loaded PEG-PLGA nanoparticles: optimized drug loading, in vitro cytotoxicity and in vivo antitumor effect. Iran J Pharm Res. 13:819–833.
- Ohgushi H, Okumura M, Yoshikawa T, Inboue K, Senpuku N, Tamai S, Shors EC. 1992. Bone formation processin porous calcium carbonate and hydroxyapatite. J Biomed Mater Res. 26:885–895.
- Patel BK, Parikh RH, Aboti PS. 2013. Development of Oral Sustained Release Rifampicin Loaded Chitosan Nanoparticles by Design of Experiment. J Drug Deliv. 2013:1–10.
- Qian K, Shi T, Tang T, Zhang S, Liu X, Cao Y. 2011. Preparation and characterization of nano-sized calcium carbonate as controlled release pesticide carrier for validamycin against Rhizoctonia solani. Microchimica Acta. 173:51–57.
- Richards RG, Harris LG, Schneider E, Haas N. 2006. Antiseptics and antibiotics on implants. Injury. 37:S113–S116.
- Soliman GM, Szychowski J, Hanessian S, Winnik FM. 2010. Robust polymeric nanoparticles for the delivery of aminoglycoside antibiotics using carboxymethyldextran-b-poly (ethyleneglycols) lightly grafted with n-dodecyl groups. Soft Matter. 6:4504–4514.
- Tange R, Dreschler W, Prins J, Buller H, Kuijper E, Speelman P. 1995. Ototoxicity and nephrotoxicity of gentamicin vs netilmicin in patients with serious infections. A randomized clinical trial. Clinical. Otolaryngol Allied Sci. 20:118–123.
- Thompson D. 1982. Response surface experimentation1. J Food Proc Preserv. 6:155–188.
- Ueno Y, Futagawa H, Takagi Y, Ueno A, Mizushima Y. 2005. Drug-incorporating calcium carbonate nanoparticles for a new delivery system. J Control Release. 103:93–98.
- Wang C, He C, Tong Z, Liu X, Ren B, Zeng F. 2006. Combination of adsorption by porous CaCO3 microparticles and encapsulation by polyelectrolyte multilayer films for sustained drug delivery. Int J Pharm. 308:160–167.
- Wesson CA, Liou LE, Todd KM, Bohach GA, Trumble WR, Bayles KW. 1998. Staphylococcus aureus Agr and Sar global regulators influence internalization and induction of apoptosis. Infect Immun. 66: 5238–5243.
- Yoncheva K, Vandervoort J, Ludwig A. 2003. Influence of process parameters of high-pressure emulsification method on the properties of pilocarpine-loaded nanoparticles. J Microencapsul. 20: 449–458.
- Yoshizawa S, Fourmy D, Puglisi JD. 1998. Structural origins of gentamicin antibiotic action. EMBO J. 17:6437–6448.