Abstract
Context: RNA interference (RNAi)-based therapeutics rely upon safe and efficient delivery of small interfering RNA (siRNA) molecules. Objective: This review explores various dimensions of RNAi with emphasis on the development of nanoparticle-based delivery vectors for safe and efficient siRNA delivery. Methods: An exhaustive database search has been done regarding studies done to investigate the potential of siRNA delivery employing nanoparticles has been cited in the present review. Results and conclusion: With the current challenges, there is a need for collaborative work allowing for the successful development of nanoparticle/siRNA complexes as health-promoting biotherapeutics.
Introduction
Since the discovery of RNA interference (RNAi), a tremendous amount of effort has been directed toward the use of this technology for the development of therapeutics. A plethora of studies have appeared citing applications of RNAi in studies defining gene silencing and gene function using mammalian cells. RNAi is a highly conserved process of selective post-transcriptional down-regulation of target-specific genes in the cells. RNAi is mediated by small double-stranded RNA (dsRNA) sequences of 21–23 nucleotides, known as small interfering RNA (siRNA). These siRNAs, in conjunction with multi-protein complexes, exhibit the potential to degrade the gene-coding messenger RNA (mRNA) molecules which are complementary to one of the siRNA strands. dsRNA-mediated gene silencing was reported for the first time by Fire et al. in Caenorhabditis elegans (CitationFire et al. 1998). Since then, RNAi has evolved as a gold standard in studies related to the elucidation of gene function in basic biological research.
Though small-molecule inhibitors and monoclonal antibodies have achieved considerable success in therapies for deadly diseases such as cancer, they are based on the concept of traditional drug design, where the therapeutic intervention occurs at the protein level (i.e., after a disease-causing gene has already been translated into protein) (CitationDruker et al. 2006, CitationSmith et al. 2007). These traditional drugs were discovered through cumbersome processes of high-throughput screening followed by hit and trial in chemical modifications for lead compounds. Although several important and revolutionizing therapeutics have been successfully developed using the traditional approaches, there remain additional challenging diseases where the success of traditional drugs remains inefficient. However, RNAi based-therapeutics, by regulating the post-transcriptional levels of a disease-causing protein in a highly sequence-specific manner, should outshine traditional drug design. Moreover, RNAi-based therapeutics can allow for the exploration of new avenues of protein-targeting, which appears intractable and time-consuming with traditional drug design strategies.
Although RNAi holds great potential, its therapeutic applications are hindered by poor cellular uptake of siRNA molecules and their rapid enzymatic degradation (CitationZhang et al. 2007). Owing to their large molecular weight (∼13 kDa) and strong anionic charge due to the presence of a phosphodiester backbone (∼40 negative phosphate charges), naked siRNA is incapable of freely crossing the cell membrane. The electrostatic repulsion from the anionic cell membrane surface results in the failure of siRNA to passively diffuse through the cell membrane appears to be one of the major barriers to RNAi success. In diseases such as cancer, it is highly desirable that siRNAs reach specific diseased organs via systemic delivery routes. Moreover, naked siRNA has a very short half-life of < 1 h in human plasma; the administered siRNAs are rapidly cleared by the reticuloendothelial system (RES) composed of macrophages and Kupffer cells (CitationSeow and Wood 2009). Unfortunately, for successful RNAi, the siRNA must reach the cytoplasm of the target cells. Hence, there arises an urgent need for a delivery vector to administer siRNA safely, effectively, and repeatedly to target tissues. The vector is expected to provide protection against enzymatic degradation, possess target specificity, enhance intracellular uptake, and escape from the endosome or lysosome into the cytosol, all resulting in efficient gene knockdown. Viral vectors originally emerged as a choice for siRNA delivery. However, safety issues related to oncogenicity, inflammation, and immunogenicity pose serious challenges toward their clinical application (CitationXu et al. 2005, CitationZaiss and Muruve 2005). The search for safer alternatives led to nanotechnology-based delivery vectors such as liposomes, nanotubes, and nanoparticles for efficient and target-specific siRNA delivery.
Nanoparticles are sub-microscopic colloidal particles in the nanometer-size range, with at least one dimension ≤ 100 nm that can entrap or adsorb drug molecules, proteins, or nucleic acids (CitationTempleton and Lasic 1999). The small size facilitates nanoparticle movement in the thin capillary beds of tissues, thereby leading to delivery of the entrapped therapeutic molecule to the different tissues of the body (CitationGaumet et al. 2008). Moreover, nanoparticles can be easily engulfed by the cells, and thus can be used to deliver drugs to intracellular targets such as the cytoplasm, mitochondria, or nucleus (CitationPanyam and Labhasetwar 2003). This review explores various dimensions related to the therapeutic potential of RNAi, that is, the mechanism of action, existing challenges, and future perspectives. Focus will be given toward nanotechnology-based approaches for the delivery of exogenous, synthetic siRNA employed for in vitro and in vivo studies. The final part of the article will revolve around the vision and expert opinion for the development of RNAi as a therapeutic entity.
Mechanism of RNA interference
The mechanism of RNAi mediated via siRNA takes place in the cytoplasm of the cells (). RNAi is a well-orchestrated multi-step process which is initiated with the binding of dsRNA molecules to a specific endonuclease (a cytoplasmic ribonuclease III (RNase III)-like protein) called Dicer, which is capable of cleaving long dsRNAs (CitationBernstein et al. 2001). The conjugation of Dicer is followed by cleavage of long dsRNA into smaller duplexes with 19 paired nucleotides and two nucleotide overhangs at both 3′-ends; these small dsRNAs hence generated are called siRNAs (CitationElbashir et al. 2001). The generated siRNA molecules further bind to a nuclease-containing multi-protein complex called RNA-induced silencing complex (RISC) (CitationHammond et al. 2000). The RISC complex possesses RNA helicase activity which facilitates unwinding of the double-stranded siRNA molecule and removal of the sense strand, which is further degraded by cellular nucleases (CitationNykanen et al. 2001). The antisense strand of siRNA remains engaged with the RISC complex and directed toward the target mRNA sequence, where it anneals complementarily by Watson–Crick base pairing. In the following step, the RISC complex cleaves the target mRNA by endonucleolytic activity, thereby preventing its translation into protein (CitationMartinez and Tuschl 2004). The endonucleolytic activity of the RISC complex is due to the presence of a cleavage enzyme called Argonaute 2 (AGO2) (CitationLiu et al. 2004). Relative to the 5′ end of the siRNA guide strand, the targeted mRNA is cleaved between bases 10 and 11 (CitationElbashir et al. 2001). Finally, the cleaved products are released and degraded by cellular nucleases which liberate the RISC complex, and this allows further search for more target mRNAs to proceed with its interference activity (CitationLeung and Whittaker 2005).
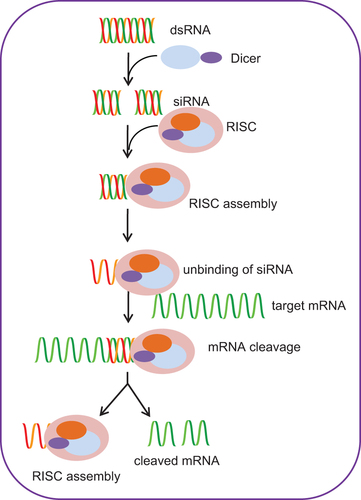
Barriers to siRNA therapeutics
Delivery vectors play a crucial role in defining the success of siRNA-based gene silencing. The timely and efficient delivery of siRNA to the target site is the decisive factor for success. However, numerous challenges are being faced by siRNA during their delivery process, hampering their efficacy. Based on the mode of study, the barriers can be divided into two broad categories—in vitro and in vivo.
In vitro barriers
Though the site of action for siRNA is quite different from that of DNA, the former being functional in the cytoplasm and the latter in the nucleus, the barriers to be overcome by the two appear similar, from cell targeting to internalization and endosomal escape (CitationGrayson et al. 2006, CitationKatas and Alpar 2006). During the complexation process, siRNA is condensed due to electrostatic interaction between amino groups present in the polycationic polymers and phosphate groups of siRNA. Various factors like siRNA concentration, pH, buffer type, and charge ratio of polymer to DNA (N/P ratio), define the size of the complexes formed. The vector/siRNA complexes, in the 50–200 nm size range, mostly enter the cells via endocytosis or pinocytosis (CitationGao et al. 2005, CitationAoki et al. 2004). The first major obstacle that has to be overcome involves preventing the aggregation of complexes in the extracellular environment and obtaining a size that can be internalized. Incorporation of poly (ethylene glycol) (PEG) or sugar molecules, for example, cyclodextrin and hyaluronic acid (HA) at the surface of nanoparticles, is performed to avoid aggregation (CitationBartlett et al. 2007, CitationLee et al. 2007). The large size (∼13 kDa) and presence of polyanionic charges on siRNA hamper their efficient uptake by cells. In order to enhance the uptake of siRNA, the initial modifications reported the use of cell-penetrating peptides (CPPs) and cholesterol (CitationMoschos et al. 2007, CitationMuratovska and Eccles 2004). Various polycationic formulations comprising chitosan, polyarginine, polylysine, histidylated polylysine peptides, polyethylenimine (PEI), and polyamidoamine dendrimers have been used to deliver siRNA (CitationMalhotra et al. 2009, CitationMalhotra et al. 2011, CitationMalhotra et al. 2013a, CitationMalhotra et al. 2013b, CitationMalhotra et al. 2013c).
The complexes, once internalized, become fused complexes bearing intracellular vesicles with the endocytic vesicles. The complexes escape endosomal degradation, for which various hypotheses have been proposed. The most accepted hypothesis of endosomal escape involves the presence of a cationic polymer, for example, polyamidoamine dendrimers or polylysine, leading to the physical disruption of the negatively charged endosomal membrane because of its direct interaction with the cationic polymer (CitationZhang and Smith 2000). Other strategies exploit the usage of either reduction-sensitive or pH-responsive polymers. PEI has ionizable amino groups, and therefore, by proton sponge effect, favors the release of endocytosed polyplexes (CitationBoussif et al. 1995, CitationThomas et al. 2005, CitationThomas and Klibanov 2002, CitationAkinc et al. 2005). Kim et al. reported that the polyaspartamide derivative (poly(PAsp(DET)) undergoes efficient endosomal escape because of a pH-dependent protonation of N-(2-aminoethyl)-2-aminoethyl groups in the PAsp(DET) side chain (CitationKim et al. 2010). Block copolymers of dimethylaminoethyl methacrylate (DMAEMA) and propylacrylic acid (PAA), which undergo structural rearrangements due to the acidic pH of endosomes, are also being investigated for siRNA delivery (CitationConvertine et al. 2009). The block consists of PAA and a cationic complexation component which mediates a hydrophilic-to-hydrophobic transition at the endosomal pH, causing disruption of the membrane. Thermosensitive cationic polymeric nanocapsules are also employed to deliver siRNA. These nanocapsules can cause physical disruption of the endosome, due to temperature-induced swelling (∼119 nm at 37°C and ∼412 nm at 15°C) (CitationLee et al. 2008).
The stability of nanoparticles is highly desirable for extracellular siRNA protection, but at the same time, in order to mediate gene silencing, it is required to decomplex and release siRNA. Hence, for efficient silencing by siRNA complexed with nanoparticles, a subtle balance between protection and release of siRNA should exist (CitationZhang et al. 2009, CitationZhang et al. 2010). To facilitate this process, bioresponsive nanoparticles have been used, where release of siRNA occurs in response to an intracellular stimulus. For example, incorporation of acid-labile ketal linkages to PEI has been found to enhance transfection and RNAi. Ketalized PEI/siRNA polyplexes resulted in higher gene silencing efficiency than unmodified linear PEI (CitationShim and Kwon, 2009), because of selective cytoplasmic localization of the polyplexes and the efficient disassembly of siRNA from the polyplexes. Polyplexes containing reducible polycations are also being studied for their capability as siRNA delivery vehicles. These polycations degrade in response to cytoplasmic redox conditions. Under physiological conditions, the reducible poly(amido ethylenimine) efficiently condenses siRNA to form stable complexes, and in presence of a reducing environment, results in complete release of siRNA (CitationHoon Jeong et al. 2007). A hundred-fold higher siRNA transfection efficacy was observed with the polyion complex (PIC) micelle prepared with a disulfide crosslinked core through the assembly of iminothiolane-modified poly(ethylene glycol)-block-poly(L-lysine) [PEG-b-(PLL-IM)] and siRNA, in comparison to non-crosslinked PICs (CitationMatsumoto et al. 2009). On one hand, siRNA is well protected from degradation and non-specific clearance by PICs in the extracellular milieu, and on the other hand, owing to the selective release of siRNA in the intracellular milieu, higher transfection efficiency is observed. Also, siRNA grafted to poly(aspartic acid) [PAsp(-SS-siRNA)] via a disulfide linkage was found to give a higher siRNA efficiency due to efficient siRNA release from the PIC under intracellular reductive conditions (CitationTakemoto et al. 2010).
In vivo barriers
The route of siRNA administration, whether intravenous, intranasal, intratracheal, subcutaneous, intratumoral, intramuscular, or oral, depending on the targeted disease, defines the in vivo barriers of siRNA therapeutics. Degradation by nuclease activity in plasma is one of the most significant biological barriers encountered by systemically administered siRNA. The major degradative enzymatic activity that occurs in the plasma is that of 3′ exonuclease; although on a smaller scale, cleavage of internucleotide bonds can also take place. Thus, one major challenge is to protect siRNA in serum. For this purpose, several chemical modifications have been proposed, including modifications of the sugars or the backbone of siRNA by 2′-O-methyl and 2′-deoxy-2′-fluoro (OMe/F) or phosphorothioate linkages (CitationAkhtar and Benter 2007). However, polymeric nanoparticle-complexed siRNA can easily bypass this barrier as it has no available sites for 3′ nuclease binding and cleavage. Efficient siRNA delivery faces another major problem in the form of rapid clearance by the RES. Endocytosed siRNA, as well as their carriers, are engulfed by Kupffer cells in the liver and spleen macrophages (CitationAlexis et al. 2008). Opsonins, which are composed of immunoglobulins, complement system proteins, and other serum proteins recognize the nanoparticles used to deliver siRNA as foreign particles in the body. Once opsonized, these particles are then taken up by a variety of receptors present on the cell surface of macrophages. Immunoglobulin G-opsonized particles are recognized by Fc receptors and complement-opsonized particles are internalized through complement receptors, leading to their degradation. RES-associated clearance can be overcome by the modifications of nanoparticle surfaces with hydrophilic polymers such as PEG that reduce the adsorption of opsonins and clearance by phagocytosis (Citationvan Vlerken et al. 2007). One of the most crucial steps involved in efficient gene silencing via siRNA is the specific recognition and binding to the target mRNA in the cytosol. Nanoparticle-complexed siRNA delivery is not free of adverse effects, and some of the off-target effects observed include toxicity, stimulation of immune response, inflammation, and undesirable effects on other genes (CitationAigner 2006). Chemical modifications of siRNA where uridine bases are replaced by their 2′-fluoro-, 2′-deoxy-, or 2′-O-methyl-modified counterparts have been reported to block immune recognition of siRNAs by toll-like Receptors (TLR) (CitationSioud 2006). Hence, another strategy has been proposed that involves the use of polymers which avoid the delivery into the endosomes or block TLR signaling to deliver siRNA. In principle, various factors should be taken into consideration both at the cellular and at the whole-organism level to design and develop efficient siRNA delivery vectors.
Nanoparticle-mediated siRNA delivery
Several polymers like PEI, chitosan, and PEG have been used to develop nanoparticles that can be further employed to deliver siRNA. In order to mediate targeted delivery via receptor-mediated endocytosis, surface ligands are used to modify the polymers that possess derivable functional groups. Chitosan is an aminoglucopyran that possesses randomly distributed N-acetylglucosamine and glucosamine residues. Chitosan has emerged as a promising candidate due to its versatile biological activity, biocompatibility, and biodegradability, along with low toxicity (CitationRinaudo 2006). Chitosan has largely become an efficient vector, and its efficacy in the delivery of siRNA is governed by several structural and experimental parameters such as molecular weight (MW) and degree of deacetylation (DDA). Katas et al. seems to be the first group to have used chitosan in vitro as a delivery vehicle for siRNA (CitationKatas and Alpar, 2006). To investigate the gene-silencing efficiency of chitosan nanoparticles, two different types of cell lines, namely, Chinese hamster ovary cells (CHO K1) and human embryonic kidney cells (HEK 293) were used (). The study revealed that the silencing effect of siRNA was in direct correlation with the method of association of chitosan with siRNA. When compared, it was observed that nanoparticles of chitosan/tripolyphosphate (TPP)-entrapping siRNA were better vectors for siRNA delivery than simply chitosan, possibly due to their high binding capacity and loading efficiency. Another group, Howard et al. engineered a novel chitosan-based siRNA nanoparticle delivery system for in vitro and in vivo RNAi. The study showed nanoparticle-mediated knockdown of endogenous Enhanced Green Fluorescent Protein (EGFP) in both human lung carcinoma cells (H1299) and murine peritoneal macrophages, with 77.9% and 89.3% fluorescence reduction, respectively (CitationHoward et al. 2006). When chitosan/siRNA formulations where nasally administered in bronchiole epithelial cells of transgenic EGFP mice, efficient in vivo RNAi was achieved (37% and 43% reduction compared to mismatch and untreated control, respectively) (CitationHoward et al. 2006). These findings highlight the novel chitosan-based system for its potential therapeutic application in RNAi-mediated therapy of systemic and mucosal disease.
Table I. Nanoparticles employed to deliver siRNA.
Using a coacervation method, size-controlled chitosan nanoparticles were prepared in the presence of polyguluronate (PG)-encapsulating siRNA (CitationLee et al. 2009). The siRNA-loaded chitosan nanoparticles formed were found to possess a mean diameter in the range of 110–430 nm, depending on the weight ratio between chitosan and siRNA. These nanoparticles efficiently delivered siRNA to the cell lines, HEK 293 T and HeLa, with reduced cytotoxicity (). In another study done on chitosan/siRNA nanoparticles, it was observed that these complexes possess irregular lamellar and dendritic structures with a hydrodynamic radius size of about 148 nm and a net positive charge, with zeta potential value of 58.5 mV (CitationJi et al. 2009). Gene knockdown studies done with chitosan/siRNA nanoparticles showed that chitosan/siRNA nanoparticles silence FHL2 gene expression by 69.6% (FHL2 is an oncogene highly expressed in various types of cancer cells, such as epithelial ovarian cancer, hepatoblastoma, colon cancer cell line—SW480, cervical cancer cell line—HeLa, and some breast cancer cells). It was also observed that in the human colon adenocarcinoma LoVo cells, siRNA-mediated reduction in FHL2 expression inhibited the growth and proliferation of human colorectal cancer cells. These results, therefore, suggest chitosan/siRNA nanoparticles as being efficient in vitro delivery systems.
PEI, designated as the gold standard of gene transfection, has high cation density (a positive charge per 43 Da, the monomer's MW), and is one of the most widely explored cationic polymers for gene delivery. Since the first successful application by Behr et al. of PEI-mediated oligonucleotide delivery, it has been further derivatized to improve the physicochemical and biological properties of polyplexes (CitationBoussif et al. 1995, CitationNeu et al. 2005). It is used for the formation of highly condensed particles by interacting with nucleic acids. PEI/siRNA complexes are more stable and uniform-sized particles as compared to DNA (CitationGrzelinski et al. 2006). The interaction of PEI and siRNA is non-covalent in nature. The complexation of synthetic siRNA with low MW PEI efficiently stabilizes siRNAs and delivers it into cells, with no toxicity and complete bioactivity (CitationUrban-Klein et al. 2005). Moreover, subcutaneous administration of these complexes in a mouse tumor model showed the delivery of intact siRNAs into the tumors. siRNA downregulated HER-2 expression, and a marked reduction in tumor growth was observed with PEI-complexed siRNAs targeting the c-erbB2/neu (HER-2) receptor that was injected intraperitoneally. Various targeting ligands have been attached to PEI to achieve targeted delivery. PEI that is PEGylated with PEG having an Arg-Gly-Asp (RGD) peptide ligand attached at the distal end allows complexation with siRNA as self-assembling nanoparticles (). These self-assembled nanoparticles then act as a means to target tumor neovasculature-expressing integrins and deliver siRNA that inhibits expression of vascular endothelial growth factor receptor-2 (VEGF R2) and tumor angiogenesis (CitationSchiffelers et al. 2004). Intravenous administration of complexes in tumor-bearing mice showed selective uptake of siRNA at the tumor site, sequence-specific reduction of protein expression by siRNA, and inhibition of both tumor angiogenesis and growth rate. In one of our studies, we prepared an ionic complex of PEI (750 kDa) and alginic acid to amalgamate the properties of cationic PEI with the polysaccharide alginate (CitationPatnaik et al. 2006). The PEI/alginate (6.26% amino groups derivatized by alginate) nanoparticles efficiently delivered siRNA into COS-1 cells, and about 80% suppression of GFP expression was observed. In another published siRNA delivery study, we prepared nanoparticles of PEI by acylating PEI with propionic anhydride followed by crosslinking with polyethylene glycol-bis(phosphate) (). When siRNA nanoparticles were delivered into HEK 293 cells, up to 85% inhibition of GFP expression was shown, comparable to that for siRNA/Lipofectin transfection (81% inhibition) (CitationNimesh and Chandra 2009). Hobel et al. used PEI nanoparticles to deliver siRNA against VEGF, and they reported the success of these nanoparticles in effective knockdown of VEGF in pancreatic and prostate cancer models (CitationHöbel et al. 2010). Further, they derivatized PEIs with maltose, maltotriose, or maltoheptaose (OM/PEI) to deliver siRNA in vitro and in vivo (CitationHobel et al. 2011). On systemic application in vivo, the OM/PEI/siRNA complexes show marked differences in the siRNA biodistribution profile, that is, substantially decreased siRNA levels in the liver and increased siRNA levels in the muscle.
Recently, cyclodextrin (CD) and CD-containing polymers (CDP) have been employed for siRNA delivery (CitationPun et al. 2004). In a study conducted in a murine model of metastatic Ewing's sarcoma, CDP-mediated siRNA delivery targeted against the EWS-FLI1 gene significantly inhibited tumor growth () (CitationHu-Lieskovan et al. 2005). These results coincided with minimal adverse effects, and long term tail vein administrations of these conjugates showed no abnormalities in interleukin-12 and interferon (IFN)-α, liver and kidney function tests, complete blood counts, or pathology of major organs. Positron emitting tomography (PET) demonstrated that both non-targeted and transferrin (TF)-targeted siRNA nanoparticles exhibited a similar biodistribution and tumor localization. siRNA nanoparticles targeting TF had tumor luciferase activity reduced by approximately 50% compared to non-targeted siRNA nanoparticles, 1 day after injection (CitationBartlett et al. 2007). Bartlett et al. conducted a study where siRNA/CDP nanoparticles were administered intravenously in A/J mice bearing subcutaneous Neuro2A tumors. Following three consecutive daily doses of TF-targeted nanoparticles carrying 2.5 mg/kg of two different siRNA sequences targeting RRM2, inhibition in tumor growth was observed (CitationBartlett and Davis 2008). When tested in non-human primates, it was found that TF-targeted siRNA/CDP nanoparticles were well-tolerated in cynomolgus monkeys at doses of 3 and 9 mg siRNA/kg by intravenous administration (CitationHeidel et al. 2007). Recently, the first in-human phase I clinical trial for siRNA/CDP has been reported. The study involved the systemic administration of siRNA to patients with solid cancers using targeted CDP nanoparticles. Nanoparticles were shown to be intracellularly localized in melanoma patients, in the results of tumor biopsies that were done for those patients (CitationDavis et al. 2010). Additionally, in comparison to pre-dosing tissues, reduction in the levels of RRM2-specific mRNA and protein were observed.
Recently, biodegradable polymers have emerged as attractive candidates for the design of siRNA-loaded polyplexes. Han et al. modified biocompatible hyaluronic acid (HA) with the cationic polymer PEI and demonstrated increased cellular uptake of siRNA with cationic PEI derivatives of HA (HA-PEI) in B16F1, A549, HeLa, and Hep3B tumor cells, as compared to PEI alone (). Further, HA-PEI used to deliver a survivin-specific siRNA resulted in significant reduction in the expression of mRNA of the target gene in all of the aforementioned cell lines (CitationHan et al. 2009). A new series of biodegradable poly(amido amine)s with disulfide linkages in the backbone has been developed by Vader et al. (CitationVader et al. 2011), out of N,N′-cystaminebisacrylamide (CBA), 4-amino-1-butanol (ABOL) and ethylene diamine (EDA). They designed siRNA against epidermal growth factor receptor (EGFR) and delivered with these polymers containing 25% or 50% EDA in 14 C cells. The results showed more than 80% gene knockdown with low cytotoxicity. In another study, Varkouhi et al. investigated two biodegradable cationic polymers for siRNA delivery—pHPMA-MPPM (poly((2-hydroxypropyl) methacrylamide 1-methyl-2-piperidine methanol)) and TMC (O-methyl-free N,N,N-trimethylated chitosan) (CitationVarkouhi et al. 2011). In H1299 human lung cancer cells, expression of the luciferase gene was reduced by 30–40% when transfected with polyplexes formulated from pHPMA-MPPM and TMC, comparable to the results obtained with Lipofectamine. Further, application of photochemical internalization (PCI), along with addition of diINF-7 peptide to the formulations, increased their silencing activity up to 70–80%. Recently, Mao et al. reported a triblock copolymer consisting of monomethoxy poly(ethylene glycol), poly(e-caprolactone), and poly(2-aminoethyl ethylene phosphate). These nanoparticles, in aqueous solution, are self-assembled into micellar nanoparticles (MNPs) with an average diameter of 60 nm and a zeta potential of 48 mV (CitationMao et al. 2011). Efficient gene knockdown effect was observed in BT474 breast cancer cells once the micelleplex formed by the interaction of MNPs and siRNA and was internalized by cells followed by siRNA release. Furthermore, siRNA targeting the acid ceramidase gene, when condensed as a micelleplex, induced significant apoptosis and reduced proliferation of cancer cells. An in vivo study showed promising results as systemic delivery of these micelleplex not only efficiently inhibited tumor growth in a BT474 xenograft murine model with suppressed acid ceramidase expression, but also showed no activation of the innate immune response.
Advantages and limitations of nanoparticles
Several advantages are attributed to the nanoparticles prepared from polymers, such as ease of manipulation with further scope to change the molecular weight (MW), geometry (linear and branched), stability, safety, low cost, and high flexibility regarding the size of the transgene delivered. Various targeting moieties such as RGD peptides or transferrin can be used to tag nanoparticles to achieve target-specific siRNA delivery (CitationSchiffelers et al. 2004, CitationDavis, 2009). Owing to their submicroscopic size (usually 10–200 nm), nanoparticles can readily penetrate the cells and interact with biomolecules on the surface or inside the cell. Also, the small size of nanoparticles allows their penetration to the tumor tissues, in depth and with a high level of specificity, thereby improving the targeted delivery of drug/gene (CitationCuenca et al. 2006). Limitations are still associated with polymeric nanoparticles, despite their advantages and successful implications in numerous studies. The destabilization and ultimate rupture of the cell membrane occurs due to strong electrostatic interactions between the polycationic polymers constituting the nanoparticles and plasma membrane proteins (CitationFischer et al. 2003). The polycationic polymers were found to have the highest toxicity followed by neutral and anionic ones in a comparative study done between polycationic, neutral, and polyanionic polymers (CitationJevprasesphant et al. 2003). In order to circumvent this problem, several strategies based on the reduction of surface charge by coating with HA or PEG have been investigated (CitationJiang et al. 2008, CitationDuan et al. 2010). Branched PEIs have been observed to be more toxic and less suitable for transfection, particularly at higher N/P ratio, than the linear ones (CitationWightman et al. 2001). A major disadvantage attached to PEI is the fact that it is a non-biodegradable polymer, and hence its accumulation within the cells could cause interference with vital intracellular processes (CitationGodbey et al. 1999, CitationGodbey et al. 2001). PEI/DNA complexes with a higher ratio of cation/anion can activate the complement system, but the extent of activation is lowered as the PEI/DNA complexes approach neutrality (CitationFerrari et al. 1997, CitationPlank et al. 1996). Chemical modifications where small MW PEIs are coupled to generate higher MW molecules using degradable bi-functional linkages have been found to reduce toxicity. Chitosan possesses minimal toxicity and is found to be an efficient in vitro and in vivo siRNA delivery vector capable of mediating gene silencing (CitationHoward et al. 2006, CitationPille et al. 2006). However, conditions for transfection using chitosan nanoparticles must be well-elucidated before their clinical applications, as chitosan suffers from low transfection efficiency and optimum conditions for transfection are not clear. Moreover, non-specific stimulation of innate immune inflammatory responses such as type I IFN production associated with RNA duplex interaction with endosomal toll-like receptors (TLR) can be potentiated with nanoparticle/siRNA delivery into the endosome (CitationMarques et al. 2006). However, TLR-7 interaction and associated non-specific effects can be disrupted to an extent when 2′-O-methyl nucleotides is incorporated into the siRNA duplex strand (CitationJudge et al. 2006).
Conclusion
RNAi discovery has become a milestone in the area of novel therapeutic approach research. However, RNAi, specifically siRNA application, has its own limitations due to low cellular uptake, short half-life in vivo, rapid clearance, and quick enzymatic degradation. All these associated disadvantages have forced advancement leading to the development of novel nanoparticle/siRNA delivery systems. Several delivery systems have been designed so far, and each has its own associated advantages and disadvantages. Various modifications that have been introduced, such as usage of PEG— done to increase residence time, and the use of cell-targeting peptides with the delivery systems, have greatly contributed to enhancing the efficacy of these delivery systems. Still, despite the advancements in this field of research, the major challenges to combat in order to ensure efficiency include the fate of the nanomaterials in vivo, their long-term side effects on gene expression and toxicity, as well the conventional methods of ADME/Tox. The efficacy and biosafety of nanoparticle/siRNA formulations need to be well monitored, with properly designed pre-clinical and clinical studies. A review of the recent advances in research suggested the use of polymeric nanoparticles to deliver functional siRNA to the target cells. Several studies have been conducted with nanoparticles on various tumor models to deliver siRNA for antitumor treatments. Though these successful research studies on animal models for systemic delivery to non-human primates have been a much awaited boost for scientists in the field, a number of hurdles and concerns must be overcome before RNAi can be harnessed as a new therapeutic modality (CitationZimmermann et al. 2006). The nanoparticles must possess long circulation time, low immunogenicity, good biocompatibility, selective targeting, and efficient penetration to barriers such as the vascular endothelium and the blood–brain barrier, self-regulating release without clinical side effects. Several nano-based vectors have been used for in vivo siRNA delivery to a variety of tumor xenograft models, with a view that they possess minimal antigenicity and high transfection efficiency. The results have shown a promising future for the technology, as remarkable suppression of tumor growth via siRNA/vector complexes was observed. However, lack of understanding of the mechanism and off-target interference by the complexes have posed a major obstacle toward development of siRNA-based therapeutics. These issues have thus raised the cry for more exhaustive and detailed planning for successful implications of siRNA complexes with efficacy and for pharmacokinetics of siRNA/vector systems in clinical applications. In this era of developing genetic therapeutics, there is a call for collaborative work by academicians and industry groups in order to develop methods for preparation of more stable polymeric nanoparticle/siRNA complexes and robust analytical methods to characterize formulations during formation and storage.
Acknowledgements
This work was supported by Science & Engineering Research Board (SERB), Department of Science and Technology (SERC Fast Track Proposals for Young Scientists Scheme), and Government of India.
Declaration of interest
The authors report no declaration of interest. The authors alone are responsible for the content and writing of the paper.
References
- Aigner A. 2006. Gene silencing through RNA interference (RNAi) in vivo: Strategies based on the direct application of siRNAs. J Biotechnol. 124:12–25.
- Akhtar S, Benter IF. 2007. Nonviral delivery of synthetic siRNAs in vivo. J Clin Invest. 117:3623–3632.
- Akinc A, Thomas M, Klibanov AM, Langer R. 2005. Exploring polyethylenimine-mediated DNA transfection and the proton sponge hypothesis. J Gene Med. 7:657–663.
- Alexis F, Pridgen E, Molnar LK, Farokhzad OC. 2008. Factors affecting the clearance and biodistribution of polymeric nanoparticles. Mol Pharm. 5:505–515.
- Aoki H, Satoh M, Mitsuzuka K, Ito A, Saito S, Funato T, et al. 2004. Inhibition of motility and invasiveness of renal cell carcinoma induced by short interfering RNA transfection of beta 1,4GalNAc transferase. FEBS Lett. 567:203–208.
- Bartlett DW, Davis ME. 2008. Impact of tumor-specific targeting and dosing schedule on tumor growth inhibition after intravenous administration of siRNA-containing nanoparticles. Biotechnol Bioeng. 99:975–985.
- Bartlett DW, Su H, Hildebrandt IJ, Weber WA, Davis ME. 2007. Impact of tumor-specific targeting on the biodistribution and efficacy of siRNA nanoparticles measured by multimodality in vivo imaging. Proc Natl Acad Sci U S A. 104:15549–15554.
- Bernstein E, Caudy AA, Hammond SM, Hannon GJ. 2001. Role for a bidentate ribonuclease in the initiation step of RNA interference. Nature. 409:363–366.
- Boussif O, Lezoualc'h F, Zanta MA, Mergny MD, Scherman D, Demeneix B, Behr JP. 1995. A versatile vector for gene and oligonucleotide transfer into cells in culture and in vivo: polyethylenimine. Proc Natl Acad Sci U S A. 92:7297–7301.
- Convertine AJ, Benoit DS, Duvall CL, Hoffman AS, Stayton PS. 2009. Development of a novel endosomolytic diblock copolymer for siRNA delivery. J Control Release. 133:221–229.
- Cuenca AG, Jiang H, Hochwald SN, Delano M, Cance WG, Grobmyer SR. 2006. Emerging implications of nanotechnology on cancer diagnostics and therapeutics. Cancer. 107:459–466.
- Davis ME. 2009. The first targeted delivery of siRNA in humans via a self-assembling, cyclodextrin polymer-based nanoparticle: from concept to clinic. Mol Pharm. 6:659–668.
- Davis ME, Zuckerman JE, Choi CHJ, Seligson D, Tolcher A, Alabi CA, et al. 2010. Evidence of RNAi in humans from systemically administered siRNA via targeted nanoparticles. Nature. 464: 1067–1070.
- Druker BJ, Guilhot F, O'brien SG, Gathmann I, Kantarjian H, Gattermann N, et al. 2006. Five-year follow-up of patients receiving imatinib for chronic myeloid leukemia. N Engl J Med. 355: 2408–2417.
- Duan Y, Yang C, Zhang Z, Liu J, Zheng J, Kong D. 2010. Poly(ethylene glycol)-grafted polyethylenimine modified with G250 monoclonal antibody for tumor gene therapy. Hum Gene Ther. 21:191–198.
- Elbashir SM, Harborth J, Lendeckel W, Yalcin A, Weber K, Tuschl T. 2001. Duplexes of 21-nucleotide RNAs mediate RNA interference in cultured mammalian cells. Nature. 411:494–498.
- Ferrari S, Moro E, Pettenazzo A, Behr JP, Zacchello F, Scarpa M. 1997. ExGen 500 is an efficient vector for gene delivery to lung epithelial cells in vitro and in vivo. Gene Ther. 4:1100–1106.
- Fire A, Xu SQ, Montgomery MK, Kostas SA, Driver SE, Mello CC. 1998. Potent and specific genetic interference by double-stranded RNA in Caenorhabditis elegans. Nature. 391:806–811.
- Fischer D, Li Y, Ahlemeyer B, Krieglstein J, Kissel T. 2003. In vitro cytotoxicity testing of polycations: influence of polymer structure on cell viability and hemolysis. Biomaterials. 24:1121–1131.
- Gao H, Shi W, Freund LB. 2005. Mechanics of receptor-mediated endocytosis. Proc Natl Acad Sci U S A. 102:9469–9474.
- Gaumet M, Vargas A, Gurny R, Delie F. 2008. Nanoparticles for drug delivery: the need for precision in reporting particle size parameters. Eur J Pharm Biopharm. 69:1–9.
- Godbey WT, Wu KK, Hirasaki GJ, Mikos AG. 1999. Improved packing of poly(ethylenimine)/DNA complexes increases transfection efficiency. Gene Ther. 6:1380–1388.
- Godbey WT, Wu KK, Mikos AG. 2001. Poly(ethylenimine)-mediated gene delivery affects endothelial cell function and viability. Biomaterials. 22:471–480.
- Grayson AC, Doody AM, Putnam D. 2006. Biophysical and structural characterization of polyethylenimine-mediated siRNA delivery in vitro. Pharm Res. 23:1868–1876.
- Grzelinski M, Urban-Klein B, Martens T, Lamszus K, Bakowsky U, Hobel S, et al. 2006. RNA interference-mediated gene silencing of pleiotrophin through polyethylenimine-complexed small interfering RNAs in vivo exerts antitumoral effects in glioblastoma xenografts. Hum Gene Ther. 17:751–766.
- Hammond SM, Bernstein E, Beach D, Hannon GJ. 2000. An RNA-directed nuclease mediates post-transcriptional gene silencing in Drosophila cells. Nature. 404:293–296.
- Han SE, Kang H, Shim GY, Kim SJ, Choi HG, Kim J, et al. 2009. Cationic derivatives of biocompatible hyaluronic acids for delivery of siRNA and antisense oligonucleotides. J Drug Target. 17:123–132.
- Heidel JD, Yu Z, Liu JY-C, Rele SM, Liang Y, Zeidan RK, et al. 2007. Administration in non-human primates of escalating intravenous doses of targeted nanoparticles containing ribonucleotide reductase subunit M2 siRNA. Proc Natl Acad Sci U S A. 104:5715–5721.
- Höbel S, Koburger I, John M, Czubayko F, Hadwiger P, Vornlocher HP, Aigner A. 2010. Polyethylenimine/small interfering RNA-mediated knockdown of vascular endothelial growth factor in vivo exerts anti-tumor effects synergistically with Bevacizumab. J Gene Med. 12:287–300.
- Hobel S, Loos A, Appelhans D, Schwarz S, Seidel J, Voit B, Aigner A. 2011. Maltose- and maltotriose-modified, hyperbranched poly(ethylene imine)s (OM-PEIs): Physicochemical and biological properties of DNA and siRNA complexes. J Control Release. 149:146–158.
- Hoon Jeong J, Christensen LV, Yockman JW, Zhong Z, Engbersen JF, Jong Kim W, et al. 2007. Reducible poly(amido ethylenimine) directed to enhance RNA interference. Biomaterials. 28:1912–1917.
- Howard KA, Rahbek UL, Liu XD, Damgaard CK, Glud SZ, Andersen MO, et al. 2006. RNA interference in vitro and in vivo using a chitosan/siRNA nanoparticle system. Mol Ther. 14:476–484.
- Hu-Lieskovan S, Heidel JD, Bartlett DW, Davis ME, Triche TJ. 2005. Sequence-Specific Knockdown of EWS-FLI1 by Targeted, Nonviral Delivery of Small Interfering RNA Inhibits Tumor Growth in a Murine Model of Metastatic Ewing's Sarcoma. Cancer Res. 65: 8984–8992.
- Jevprasesphant R, Penny J, Jalal R, Attwood D, Mckeown NB, D'emanuele A. 2003. The influence of surface modification on the cytotoxicity of PAMAM dendrimers. Int J Pharm. 252:263–266.
- Ji AM, Su D, Che O, Li WS, Sun L, Zhang ZY, et al. 2009. Functional gene silencing mediated by chitosan/siRNA nanocomplexes. Nanotechnology. 20:405103.
- Jiang G, Park K, Kim J, Kim KS, Oh EJ, Kang H, et al. 2008. Hyaluronic acid-polyethyleneimine conjugate for target specific intracellular delivery of siRNA. Biopolymers. 89:635–642.
- Judge AD, Bola G, Lee AC, Maclachlan I. 2006. Design of noninflammatory synthetic siRNA mediating potent gene silencing in vivo. Mol Ther. 13:494–505.
- Katas H, Alpar HO. 2006. Development and characterisation of chitosan nanoparticles for siRNA delivery. J Control Release. 115:216–225.
- Kim HJ, Ishii A, Miyata K, Lee Y, Wu S, Oba M, et al. 2010. Introduction of stearoyl moieties into a biocompatible cationic polyaspartamide derivative, PAsp(DET), with endosomal escaping function for enhanced siRNA-mediated gene knockdown. J Control Release. 145:141–148.
- Lee DW, Yun KS, Ban HS, Choe W, Lee SK, Lee KY. 2009. Preparation and characterization of chitosan/polyguluronate nanoparticles for siRNA delivery. J Control Release. 139:146–152.
- Lee H, Mok H, Lee S, Oh YK, Park TG. 2007. Target-specific intracellular delivery of siRNA using degradable hyaluronic acid nanogels. J Control Release. 119:245–252.
- Lee SH, Choi SH, Kim SH, Park TG. 2008. Thermally sensitive cationic polymer nanocapsules for specific cytosolic delivery and efficient gene silencing of siRNA: Swelling induced physical disruption of endosome by cold shock. J Control Release. 125:25–32.
- Leung RK, Whittaker PA. 2005. RNA interference: From gene silencing to gene-specific therapeutics. Pharmacol Ther. 107: 222–239.
- Liu J, Carmell MA, Rivas FV, Marsden CG, Thomson JM, Song JJ, et al. 2004. Argonaute2 is the catalytic engine of mammalian RNAi. Science. 305:1437–1441.
- Malhotra M, Kulamarva A, Sebak S, Paul A, Bhathena J, Mirzaei M, Prakash S. 2009. Ultrafine chitosan nanoparticles as an efficient nucleic acid delivery system targeting neuronal cells. Drug Dev Ind Pharm. 35:719–726.
- Malhotra M, Lane C, Tomaro-Duchesneau C, Saha S, Prakash S. 2011. A novel method for synthesizing PEGylated chitosan nanoparticles: strategy, preparation, and in vitro analysis. Int J Nanomedicine. 6:485–494.
- Malhotra M, Tomaro-Duchesneau C, Prakash S. 2013a. Synthesis of TAT peptide-tagged PEGylated chitosan nanoparticles for siRNA delivery targeting neurodegenerative diseases. Biomaterials. 34:1270–1280.
- Malhotra M, Tomaro-Duchesneau C, Saha S, Kahouli I, Prakash S. 2013b. Development and characterization of chitosan-PEG-TAT nanoparticles for the intracellular delivery of siRNA. Int J Nanomedicine. 8:2041–2052.
- Malhotra M, Tomaro-Duchesneau C, Saha S, Prakash S. 2013c. Systemic siRNA Delivery via Peptide-Tagged Polymeric Nanoparticles, Targeting PLK1 Gene in a Mouse Xenograft Model of Colorectal Cancer. Int J Biomater. 2013:252531.
- Mao CQ, Du JZ, Sun TM, Yao YD, Zhang PZ, Song EW, Wang J. 2011. A biodegradable amphiphilic and cationic triblock copolymer for the delivery of siRNA targeting the acid ceramidase gene for cancer therapy. Biomaterials. 32:3124–3133.
- Marques JT, Devosse T, Wang D, Zamanian-Daryoush M, Serbinowski P, Hartmann R, et al. 2006. A structural basis for discriminating between self and nonself double-stranded RNAs in mammalian cells. Nat Biotech. 24:559–565.
- Martinez J, Tuschl T. 2004. RISC is a 5′ phosphomonoester-producing RNA endonuclease. Genes Dev. 18:975–980.
- Matsumoto S, Christie RJ, Nishiyama N, Miyata K, Ishii A, Oba M, et al. 2009. Environment-responsive block copolymer micelles with a disulfide cross-linked core for enhanced siRNA delivery. Biomacromolecules. 10:119–127.
- Moschos SA, Jones SW, Perry MM, Williams AE, Erjefalt JS, Turner JJ, et al. 2007. Lung delivery studies using siRNA conjugated to TAT(48–60) and penetratin reveal peptide induced reduction in gene expression and induction of innate immunity. Bioconjug Chem. 18:1450–1459.
- Muratovska A, Eccles MR. 2004. Conjugate for efficient delivery of short interfering RNA (siRNA) into mammalian cells. FEBS Lett. 558:63–68.
- Neu M, Fischer D, Kissel T. 2005. Recent advances in rational gene transfer vector design based on poly(ethylene imine) and its derivatives. J Gene Med. 7:992–1009.
- Nimesh S, Chandra R. 2009. Polyethylenimine nanoparticles as an efficient in vitro siRNA delivery system. Eur J Pharm Biopharm. 73:43–49.
- Nykanen A, Haley B, Zamore PD. 2001. ATP requirements and small interfering RNA structure in the RNA interference pathway. Cell. 107:309–321.
- Panyam J, Labhasetwar V. 2003. Dynamics of endocytosis and exocytosis of poly(D,L-lactide-co-glycolide) nanoparticles in vascular smooth muscle cells. Pharm Res. 20:212–220.
- Patnaik S, Aggarwal A, Nimesh S, Goel A, Ganguli M, Saini N, et al. 2006. PEI-alginate nanocomposites as efficient in vitro gene transfection agents. J Control Release. 114:398–409.
- Pille JY, Li H, Blot E, Bertrand JR, Pritchard LL, Opolon P, et al. 2006. Intravenous delivery of anti-RhoA small interfering RNA loaded in nanoparticles of chitosan in mice: safety and efficacy in xenografted aggressive breast cancer. Hum Gene Ther. 17: 1019–1026.
- Plank C, Mechtler K, Szoka FC, Jr, Wagner E. 1996. Activation of the complement system by synthetic DNA complexes: a potential barrier for intravenous gene delivery. Hum Gene Ther. 7:1437–1446.
- Pun SH, Bellocq NC, Liu A, Jensen G, Machemer T, Quijano E, et al. 2004. Cyclodextrin-modified polyethylenimine polymers for gene delivery. Bioconjug Chem. 15:831–840.
- Rinaudo M. 2006. Chitin and chitosan: Properties and applications. Prog Polym Sci. 31:603–632.
- Schiffelers RM, Ansari A, Xu J, Zhou Q, Tang Q, Storm G, et al. 2004. Cancer siRNA therapy by tumor selective delivery with ligand-targeted sterically stabilized nanoparticle. Nucleic Acids Res. 32:e149.
- Seow Y, Wood MJ. 2009. Biological gene delivery vehicles: beyond viral vectors. Mol Ther. 17:767–777.
- Shim MS, Kwon YJ. 2009. Acid-Responsive Linear Polyethylenimine for Efficient, Specific, and Biocompatible siRNA Delivery. Bioconjug Chem. 20:488–499.
- Sioud M. 2006. Single-stranded small interfering RNA are more immunostimulatory than their double-stranded counterparts: a central role for 2′-hydroxyl uridines in immune responses. Eur J Immunol. 36:1222–1230.
- Smith I, Procter M, Gelber RD, Guillaume S, Feyereislova A, Dowsett M, et al. 2007. 2-year follow-up of trastuzumab after adjuvant chemotherapy in HER2-positive breast cancer: a randomised controlled trial. Lancet. 369:29–36.
- Takemoto H, Ishii A, Miyata K, Nakanishi M, Oba M, Ishii T, et al. 2010. Polyion complex stability and gene silencing efficiency with a siRNA-grafted polymer delivery system. Biomaterials. 31:8097–8105.
- Templeton NS, Lasic DD. 1999. New directions in liposome gene delivery. Mol Biotechnol. 11:175–180.
- Thomas M, Klibanov AM. 2002. Enhancing polyethylenimine's delivery of plasmid DNA into mammalian cells. Proc Natl Acad Sci U S A. 99:14640–14645.
- Thomas M, Lu JJ, Ge Q, Zhang C, Chen J, Klibanov AM. 2005. Full deacylation of polyethylenimine dramatically boosts its gene delivery efficiency and specificity to mouse lung. Proc Natl Acad Sci U S A. 102:5679–5684.
- Urban-Klein B, Werth S, Abuharbeid S, Czubayko F, Aigner A. 2005. RNAi-mediated gene-targeting through systemic application of polyethylenimine (PEI)-complexed siRNA in vivo. Gene Ther. 12:461–466.
- Vader P, Van Der Aa LJ, Engbersen JF, Storm G, Schiffelers RM. 2011. Disulfide-Based Poly(amido amine)s for siRNA Delivery: Effects of Structure on siRNA Complexation, Cellular Uptake, Gene Silencing and Toxicity. Pharm Res. 28:1013–1022.
- Van Vlerken LE, Vyas TK, Amiji MM. 2007. Poly(ethylene glycol)-modified nanocarriers for tumor-targeted and intracellular delivery. Pharm Res. 24:1405–1414.
- Varkouhi AK, Lammers T, Schiffelers RM, Van Steenbergen MJ, Hennink WE, Storm G. 2011. Gene silencing activity of siRNA polyplexes based on biodegradable polymers. Eur J Pharm Biopharm. 77:450–457.
- Wightman L, Kircheis R, Rossler V, Carotta S, Ruzicka R, Kursa M, Wagner E. 2001. Different behavior of branched and linear polyethylenimine for gene delivery in vitro and in vivo. J Gene Med. 3:362–372.
- Xu D, Mccarty D, Fernandes A, Fisher M, Samulski RJ, Juliano RL. 2005. Delivery of MDR1 small interfering RNA by self-complementary recombinant adeno-associated virus vector. Mol Ther. 11:523–530.
- Zaiss AK, Muruve DA. 2005. Immune responses to adeno-associated virus vectors. Curr Gene Ther. 5:323–331.
- Zhang K, Fang H, Wang Z, Li Z, Taylor JS, Wooley KL. 2010. Structure-activity relationships of cationic shell-crosslinked knedel-like nanoparticles: shell composition and transfection efficiency/cytotoxicity. Biomaterials. 31:1805–1813.
- Zhang K, Fang H, Wang Z, Taylor JS, Wooley KL. 2009. Cationic shell-crosslinked knedel-like nanoparticles for highly efficient gene and oligonucleotide transfection of mammalian cells. Biomaterials. 30:968–977.
- Zhang S, Zhao B, Jiang H, Wang B, Ma B. 2007. Cationic lipids and polymers mediated vectors for delivery of siRNA. J Control Release. 123:1–10.
- Zhang ZY, Smith BD. 2000. High-generation polycationic dendrimers are unusually effective at disrupting anionic vesicles: membrane bending model. Bioconjug Chem. 11:805–814.
- Zimmermann TS, Lee AC, Akinc A, Bramlage B, Bumcrot D, Fedoruk MN, et al. 2006. RNAi-mediated gene silencing in non-human primates. Nature. 441:111–114.