Abstract
The biological synthesis of metal nanoparticles is of great interest in the field of nanotechnology. The present work highlights the extracellular biological synthesis of silver nanoparticles using Pseudomonas deceptionensis DC5. The particles were synthesized in the culture supernatant within 48 h of incubation. Extracellular synthesis of silver nanoparticles in the culture supernatant was confirmed by ultraviolet–visible spectroscopy, which showed the absorption peak at 428 nm, and also under field emission transmission electron microscopy which displayed the spherical shape. In addition, the particles were characterized by X-ray diffraction spectroscopy, which corresponds to the crystalline nature of nanoparticles, and energy-dispersive X-ray analysis which exhibited the intense peak at 3 keV, resembling the silver nanoparticles. Further, the synthesized nanoparticles were examined by elemental mapping which displayed the dominance of the silver element in the synthesized product, and dynamic light scattering which showed the distribution of silver nanoparticles with respect to intensity, volume, and number of particles. Moreover, the silver nanoparticles have been found to be quite active in antimicrobial activity and biofilm inhibition activity against pathogenic microorganisms. Thus, the present work emphasized the prospect of using the P. deceptionensis DC5 to achieve the extracellular synthesis of silver nanoparticles in a facile and environmental manner.
Introduction
Nanotechnology refers broadly to the synthesis of nanoparticles and the analysis of their applicability in the physical, chemical, and biological fields. Nanoparticles have been well applied in the medical, clinical, and biological fields as biosensors, drug and gene delivery vehicles, for photo imaging and photothermal therapy, etc. (CitationKogan et al. 2007, CitationMody et al. 2010, CitationLiao et al. 2006). The distinctive properties of nanoparticles which make them interesting are the tunable physiochemical properties, strong optical absorption, and localized surface plasmon resonance. These properties enable the applications of nanoparticles for the development of novel nanomaterials and biomaterials (CitationMody et al. 2010). Numerous approaches are available for the synthesis of silver nanoparticles by physical and chemical methods, such as thermal decomposition, electrochemical and microwave-assisted processes, etc. (CitationGutierrez-Wing et al. 2012). However, many of the approaches involve the use of hazardous solvents, chemicals, and toxic reagents, and are characterized by low material conversion and high energy consumption (CitationMakarov et al. 2014, CitationSingh et al. 2015b). Thus, the development of facile, reliable, and green procedures for the synthesis of nanomaterials is a significant aspect of nanotechnology. One approach that displays immense potential is based on the green synthesis of nanoparticles using biological resources such as plant extracts, fungi, bacteria, and yeast (CitationGajbhiye et al. 2009, CitationMourato et al. 2011, CitationMishra et al. 2011). Many previous studies have demonstrated the relationship between microbes and metals, such as bioremediation, biomineralization, microbial corrosion, and bioleaching. In addition to this, several microorganisms have been explored for their potential to synthesize metallic nanoparticles (CitationSamadi et al. 2009, CitationSunkar and Nachiyar 2012). The methodologies were found to be reliable, efficient, and eco-friendly, and avoid the disadvantage of chemical and physical synthesis. Following the green methodology, the present work is focused on the development of a process for extracellular biosynthesis of silver nanoparticles using P. deceptionensis DC5, isolated from a soil sample. The development of antimicrobial resistance in pathogenic microorganisms and the ability of hazardous microorganisms to form biofilms are of major concern. Previously, silver nanoparticles have been studied as antibacterial, antiviral, antifungal, anticancer, anti-inflammatory, and antiangiogenic agents (CitationNaqvi et al. 2013). In the present study, the synthesized silver nanoparticles have been applied against pathogenic and biofilm-forming microorganisms, to explore the applicability of silver nanoparticles in the clinical and medical fields, against human pathogenic microorganisms (CitationSingh et al. 2015b).
Materials and methods
Materials
Silver nitrate was purchased from Sigma-Aldrich Chemicals, USA. All the media were purchased from Difco, MB Cell, Seoul, Republic of Korea. The bacterial strains used were Staphylococcus aureus [ATCC 6538], Salmonella enterica [ATCC 13076], Vibrio parahaemolyticus [ATCC 33844], Bacillus anthracis [NCTC 10340], Pseudomonas aeruginosa [ATCC 27853], and Candida albicans [KACC 30062]. The bacterial strains were cultured on nutrient agar media at 37°C and preserved at − 70°C in glycerol stock vials for further study. C. albicans was cultured on Sabouraud dextrose agar (SDA) at 28°C and preserved at − 70°C in glycerol stock vials containing Glucose yeast peptone (GYP) broth.
Isolation and molecular identification of bacteria
A soil sample was obtained from the campus of Kyung Hee University. The soil sample was serially diluted in sterile 0.8% NaCl and then spread onto Tryptic soy agar (TSA) media, to obtain isolated colonies. The plate was further incubated at 30°C for 48 h and observed for bacterial growth. The isolated colonies were subcultured and obtained in the pure form for further experiments. Molecular identification of the isolated strain was carried out using a 16S rRNA sequencing-based method. The genomic DNA was extracted using a commercial genomic DNA extraction kit (Core Bio System). The 16S rRNA gene was amplified from the chromosomal DNA of the isolated strain using the universal bacterial primer set 27F, 518F, 800R, and 1512R. The purified PCR products were sequenced by Genotech (Daejeon, Korea). The nearly complete sequence (1466 bp) of the 16S rRNA was compiled using SeqMan software (version 4.1). The 16S rRNA gene sequences of related taxa were obtained from the GenBank database and the EzTaxon-e server (CitationKim et al. 2012).
Extracellular synthesis of silver nanoparticles
For extracellular synthesis of silver nanoparticles, the previously described method was followed (CitationSingh et al. 2015a). Briefly, the selected bacterial isolate was inoculated into 100 ml of sterile Tryptic soy broth (TSB) in a 250 ml Erlenmeyer flask. The cultured flasks were incubated in a rotating shaker set at 120 rpm for 24 h and 37°C. After the incubation time, the culture was centrifuged at 8000 rpm for 10 min to remove the bacterial pellet. The supernatant obtained was used for extracellular production of silver nanoparticles with the addition of 1 mM filter-sterilized AgNO3 solution. Further, the culture supernatant with 1 mM AgNO3 was incubated in an orbital shaker for 48 h, at 200 rpm and 25°C. The synthesis of silver nanoparticles was monitored by visual inspection for a change in the color of the culture medium. After completion of the incubation period, the mixture was first centrifuged at 2000 rpm for 5 min to remove any components of the medium, and then the silver nanoparticles were collected by high speed centrifugation at 16000 rpm for 20 min. The obtained product was washed thoroughly in water. Then, the silver nanoparticles were collected in the form of a pellet and were used for characterization.
Characterization of silver nanoparticles
To analyze the extracellular synthesis of silver nanoparticles, the reaction mixture was scanned in the range of 300–700 nm in a UV–Vis spectrophotometer (UV–Vis) (Ultrospec 2100 Pro, Amersham, Biosciences), after the incubation period. The X-ray diffraction (XRD) analyses were performed using an X-ray diffractometer D8 Advance, (Bruker, Germany), operated at 40 kV, 40 mA, with CuKα radiation, at a scanning rate of 6°/min and a step size of 0.02, over the 2θ range of 20–80°. For XRD sample preparation, the purified nanoparticles were air-dried, and obtained in powder form. Field emission transmission electron microscopy (FE-TEM), energy dispersive X-ray spectroscopy (EDX), and elemental mapping with a JEM-2100F (JEOL) instrument operated at 200 kV were used to examine the shape, morphology, and elemental distribution of the nanoparticles. For FE-TEM analysis, the sample was prepared by placing a drop of the silver nanoparticles collected on a carbon-coated copper grid and subsequently drying in an oven at 60°C, before transferring to the microscope (CitationSingh et al. 2015a). The size distribution profile of the nanoparticles was studied using dynamic light scattering (DLS) (Photal, Otsuka Electronics, Japan). The hydrodynamic diameters and polydispersity index (PDI) were analyzed at 25°C. As a reference dispersive medium, pure water with a refractive index of 1.3328, viscosity of 0.8878, and a dielectric constant of 78.3 was used.
Antimicrobial activity of silver nanoparticles
The antimicrobial activity of nanoparticles against pathogenic microorganisms such as S. aureus, S. enterica, V. parahaemolyticus, C. albicans, and B. anthracis was measured on Muller-Hinton agar (MHA) plates using the well diffusion method. In this assay, the MHA medium plates were coated evenly with 100 μL of an overnight log culture of test organisms, and 100 μL of freshly prepared reaction mixture containing the silver nanoparticle solution was placed in the wells on the agar plates. Then, the plates were incubated at 37°C for 24 h. After the incubation period, the zones of inhibition around each well were measured with respect to the standard deviation (CitationSingh et al. 2015a).
Biofilm inhibition activity
The activity of silver nanoparticles in biofilm degradation was determined by the colorimetric method against S. aureus and P. aeruginosa (CitationGurunathan et al. 2014). Briefly, the wells of 96-well micro-titer plates were filled with 100 μL of the log phase of S. aureus and P. aeruginosa, grown overnight. After culturing for 24 h, different concentrations of silver nanoparticles (ranging from 1–7 μg) were added. The cell culture plates were then incubated for 4 h at 37°C. After incubation, the media were removed and the wells were washed three times with 200 μl of sterile water. Then, the microtiter plate was left to air-dry, for 45 min. Next, 200 μL of a 0.1% (v/v) crystal violet solution in water was added into each well and kept for 45 min. The wells were then washed three times with 300 μL of sterile water to remove excess stain. The dye incorporated by the adherent cells was solubilized with 200 μL of 95% (v/v) ethanol. The absorbance of each well was measured at 595 nm using a microtiter ELISA reader. The absorbance was measured and the percentage inhibition of biofilm activity was calculated using the following equation: [1 − (A595 of test/A595 of control)] × 100 (Wei et al. 2006). The experiments were performed in duplicate and data were interpreted in terms of mean ± SD.
Results and discussion
Screening and identification of bacteria
On the basis of molecular characterization of the bacterial isolate, DC5 showed 99.24% similarity with Pseudomonas deceptionensis. The 16S rRNA sequence has been submitted to NCBI, with accession number KR338996. The strain P. deceptionensis was isolated for the first time from the Antarctic area of the South Shetland Islands, and characterized on the basis of taxonomic studies as cold-adapted bacteria, Gram negative, psychrotolerant, aerobic, rod-shaped, catalase- and oxidase-positive bacteria, and bacteria with polar flagellar motility (CitationCarrion et al. 2011). On the basis of the percentage of gene similarity of the 16S rRNA, the isolate was named Pseudomonas deceptionensis DC5. The result of screening displayed that the isolate P. deceptionensis DC5 was capable of tolerating silver nitrate at a concentration of 1 mM on the TSA plate ( and ).
Synthesis and characterization of silver nanoparticles
In the current work, silver nanoparticles were successfully synthesized extracellularly in the culture supernatant of P. deceptionensis DC5. The reaction mixture was observed for the change in color after the incubation period. No color change was observed in the control flask containing only TSB medium with 1 mM AgNO3, kept under the same conditions (), whereas the contents of the experimental flask turned completely brown (). As the biosynthesis proceeded, the reduction of silver ions continued, and the color changed gradually from light yellow to dark brown within 48 h. This brown coloration could be due to the surface plasmon vibrations of silver nanoparticles formed in the reaction mixture (CitationSingh et al. 2015a). The synthesis was extracellular, involving the action of extracellular enzymes and proteins released in the culture supernatant. Additionally, the approach evades the downstream processing necessary for intracellular synthesis by microorganisms, and makes the process greener and more suitable for commercial purposes (CitationKumar and Mamidyala 2011).
For the characterization of the silver nanoparticles, the reaction mixture was monitored by UV–Vis spectral analysis. The reaction mixture was continuously scanned in the range of 300–700 nm at different time intervals, to analyze the synthesis of nanoparticles in the reaction mixture. The scanned graph showed the highest peak in the region of 400–500 nm, which corresponds to the presence of silver nanoparticles in the reaction mixture (). After the completion of the incubation period, the reaction mixture showed a strong peak at 428 nm, which is attributed to the surface plasmon resonance band of the silver nanoparticles. Studies propose that the band located in this region resembles that of silver nanoparticle synthesis due to the surface plasmon resonance of particles (CitationWang et al. 2015). The XRD pattern of nanoparticles exhibited intense peaks in the whole spectrum of the 2θ value ranging from 20–80, and this pattern was similar to the Braggs's reflection of silver nanocrystals (). Thus, the reaction mixture indicated the formation of silver nanoparticles.
Figure 2. Time dependent UV-Vis spectra of reaction mixture, which shows the synthesis of silver nanoparticles (a), XRD pattern of silver nanoparticles (b).
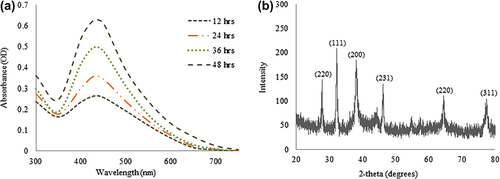
Numerous studies have categorized the shape and size of silver nanoparticles based on TEM structures (CitationSingh et al. 2015b). In the current work, FE-TEM images of silver nanoparticles revealed the spherical shape of nanoparticles (). According to the results of FE-TEM, the particles are relatively uniform in shape, with little variation in size, from 10–30 nm. The purity and elemental composition of the nanoparticles was determined by EDX and elemental mapping. In the EDX spectrum, the nanoparticles displayed a peak at 3 keV (), which is due to the absorption of metallic silver nanocrystallites corresponding to surface plasmon resonance (CitationSingh et al. 2015c). ( and ) shows the elemental mapping results of the biosynthesized silver nanoparticles, which indicates the maximum distribution of the silver element in the obtained product, further suggesting that silver was the major metal element observed. The other elements, such as copper, carbon, and chlorine were also observed (CitationSingh et al. 2015c, CitationSingh et al. 2015b). The distribution of carbon and copper was due to the use of a TEM grid, and slight contamination of chromium and chlorine appeared. The particle size analysis showed the relative distribution of synthesized silver nanoparticles with respect to intensity, number, and volume of nanoparticles. The average particles size was found as 127nm with a PDI value of 0.191 (). Thus, the results indicate that the strain P. deceptionensis DC5 has been found to be capable of synthesizing silver nanoparticles extracellularly in the culture supernatant within 48 h.
Antimicrobial and biofilm inhibition activity
The antimicrobial potential of silver nanoparticles is well known and has been applied in many biological, medical, and clinical areas. The silver nanoparticles have also been found to be quite active against multi-drug-resistant microorganisms (CitationNaqvi et al. 2013). Thus, the studies applied the synthesized nanoparticles against the pathogenic strains S. aureus, S. enterica, V. parahaemolyticus, C. albicans, and B. anthracis. To analyze the results, the zone of inhibition was measured around each well, after the incubation period. The results indicated that the extracellularly synthesized silver nanoparticles follow the following order with regard to their antimicrobial potential against pathogenic microorganisms: maximum for V. parahaemolyticus, followed by C. albicans, S. aureus, S. enterica, and B. anthracis (). The results were comparable and followed the previous studies based on antimicrobial activity of silver nanoparticles (CitationNaqvi et al. 2013, CitationSingh et al. 2015a). Additionally, the results of the biofilm inhibition test were found positive, and the results showed that the synthesized silver nanoparticles were capable of inhibiting the biofilm at a concentration of 5 μg/L against S. aureus and P. aeruginosa (). Thus, the study has demonstrated the extracellular synthesis of silver nanoparticles using P. deceptionensis DC5 in a rapid and facile manner, and further, the synthesized silver nanoparticles have been confirmed to have antimicrobial potential against pathogenic microorganisms.
Conclusion
As part of our investigation, we observed that when culture supernatant of P. deceptionensis DC5 was exposed to the aqueous silver ions, the ions were reduced in solution, thereby leading to the synthesis of silver nanoparticles. The methodology that was applied for the extracellular synthesis of silver nanoparticles was quite facile and economical. The synthesized nanoparticles were spherical, with dimensions in the range of 10–30 nm. The nanoparticles were characterized UV–Vis, XRD, EDX, DLS, and FE-TEM, which confirm the reduction of silver ions to silver nanoparticles. Moreover, the antimicrobial activity against S. aureus, S. enterica, V. parahaemolyticus, C. albicans, and B. anthracis, and biofilm inhibition activity against S. aureus and P. aeruginosa suggested the immense use of synthesized silver nanoparticles in the medical, clinical, and biological fields for their effective antimicrobial function.
Acknowledgments
This research was supported by the Korea Institute of Planning & Evaluation for Technology in Food, Agriculture, Forestry & Fisheries (KIPET NO: 313038-03-2-SB010), Republic of Korea.
Declaration of interest
The authors report no declarations of interest. The authors alone are responsible for the content and writing of the paper.
References
- Carrion O, Minana-Galbis D, Montes MJ, Mercade E. 2011. Pseudomonas deceptionensis sp. nov., a psychrotolerant bacterium from the Antarctic. Int J Syst Evol Microbiol. 61:2401–2405.
- Gajbhiye M, Kesharwani J, Ingle A, Gade A, Rai M. 2009. Fungus-mediated synthesis of silver nanoparticles and their activity against pathogenic fungi in combination with fluconazole. Nanomedicine. 5:382–386.
- Gurunathan S, Han JW, Kwon DN, Kim JH. 2014. Enhanced antibacterial and anti-biofilm activities of silver nanoparticles against Gram-negative and Gram-positive bacteria. Nanoscale Res Lett. 9:373.
- Gutierrez-Wing C, Velazquez-salazar JJ, Jose-yacaman M. 2012. Procedures for the synthesis and capping of metal nanoparticles. Methods Mol Biol. 906:3–19.
- Kim OS, Cho YJ, Lee K, Yoon SH, Kim M, Na H, et al. 2012. Introducing EzTaxon-e: a prokaryotic 16S rRNA gene sequence database with phylotypes that represent uncultured species. Int J Syst Evol Microbiol. 62:716–721.
- Kogan MJ, Olmedo I, Hosta L, Guerrero AR, Cruz LJ, Albericio F. 2007. Peptides and metallic nanoparticles for biomedical applications. Nanomedicine (Lond). 2:287–306.
- Kumar CG, Mamidyala SK. 2011. Extracellular synthesis of silver nanoparticles using culture supernatant of Pseudomonas aeruginosa. Colloids Surf B Biointerfaces. 84:462–466.
- Liao H, Nehl CL, Hafner JH. 2006. Biomedical applications of plasmon resonant metal nanoparticles. Nanomedicine (Lond). 1:201–208.
- Makarov VV, Love AJ, Sinitsyna OV, Makarova SS, Yaminsky IV, Taliansky ME, Kalinina NO. 2014. “Green” nanotechnologies: synthesis of metal nanoparticles using plants. Acta Naturae. 6:35–44.
- Mishra A, Tripathy SK, Yun SI. 2011. Bio-synthesis of gold and silver nanoparticles from Candida guilliermondii and their antimicrobial effect against pathogenic bacteria. J Nanosci Nanotechnol. 11:243–248.
- Mody VV, Siwale R, Singh A, Mody HR. 2010. Introduction to metallic nanoparticles. J Pharm Bioallied Sci. 2:282–289.
- Mourato A, Gadanho M, Lino AR, Tenreiro R. 2011. Biosynthesis of crystalline silver and gold nanoparticles by extremophilic yeasts. Bioinorg Chem Appl. 2011:546074.
- Naqvi SZ, Kiran U, Ali MI, Jamal A, Hameed A, Ahmed S, Ali N. 2013. Combined efficacy of biologically synthesized silver nanoparticles and different antibiotics against multidrug-resistant bacteria. Int J Nanomedicine. 8:3187–3195.
- Samadi N, Golkaran D, Eslamifar A, Jamalifar H, Fazeli MR, Mohseni FA. 2009. Intra/extracellular biosynthesis of silver nanoparticles by an autochthonous strain of Proteus mirabilis isolated from photographic waste. J Biomed Nanotechnol. 5: 247–253.
- Singh P, Kim YJ, Singh H, Wang C, Hwang KH, Farh Mel A, Yang DC. 2015a. Biosynthesis, characterization, and antimicrobial applications of silver nanoparticles. Int J Nanomedicine. 10:2567–2577.
- Singh P, Kim YJ, Wang C, Mathiyalagan R, El-agamy farh M, Yang DC. 2015b. Biogenic silver and gold nanoparticles synthesized using red ginseng root extract, and their applications. Artif Cells Nanomed Biotechnol. 1–6. [Epub ahead of print]
- Singh P, Kim YJ, Wang C, Mathiyalagan R, Yang DC. 2015c. The development of a green approach for the biosynthesis of silver and gold nanoparticles by using Panax ginseng root extract, and their biological applications. Artif Cells Nanomed Biotechnol. 1–8. [Epub ahead of print]
- Sunkar S, Nachiyar CV. 2012. Biogenesis of antibacterial silver nanoparticles using the endophytic bacterium Bacillus cereus isolated from Garcinia xanthochymus. Asian Pac J Trop Biomed. 2:953–959.
- Wang C, Kim YJ, Singh P, Mathiyalagan R, Jin Y, Yang DC. 2015. Green synthesis of silver nanoparticles by Bacillus methylotrophicus, and their antimicrobial activity. Artif Cells Nanomed Biotechnol. 1–6. [Epub ahead of print] Wei GX, Campagna AN, Bobek LA. 2006. Effect of MUC7 peptides on the growth of bacteria and on Streptococcus mutans biofilm. J Antimicrob Chemother. 57 1100–1109.