Abstract
This work examines the variation of longitudinal relaxation rate R1(= 1/T1) of the 19F-CF3-resonance of semifluorinated alkanes (SFAs) with oxygen tension (pO2), temperature (T) and pH in vitro. Contrary to their related perfluorocarbons (PFCs), SFA are amphiphilic and facilitate stable emulsions, a prerequisite for clinical use. A linear relationship between R1 and pO2 was confirmed for the observed SFAs at different temperatures. Using a standard saturation recovery sequence, T1 has been successfully measured using fluorine 19F-MRI with a self-constructed birdcage resonator at 9.4 T. A calibration curve to calculate pO2 depending on T and R1 was found for each SFA used. In contrast to the commonly used PFC, SFAs are less sensitive to changes in pO2, but more sensitive to changes in temperature. The influence of pH to R1 was found to be negligible.
Introduction
Efficacy of radiotherapy and chemotherapy is significantly reduced in low oxygenated (hypoxic) tumors (Tatum et al. Citation2006). Thus, tumor control and overall survival are reduced. Since inverse treatment planning in radiotherapy can compensate these effects of hypoxia, the precise determination of hypoxic volumes is crucial. There are several approaches to measure hypoxia in tissue (Domsch et al. Citation2014, Graves and Giaccia Citation2007, Padhani et al. Citation2007, Tatum et al. Citation2006). Ideal methods for clinical application should be non-invasive and should be based on routinely available imaging modalities, e.g., positron emission tomography (PET), magnetic resonance imaging (MRI) or computer tomography (CT) (Horsman et al. Citation2012). Approaches that are based on MRI are of significant interest because they provide good spatial resolution and do not use ionizing radiation.
Molecular oxygen is paramagnetic and tends to shorten T1 of fluorine compounds in solution or in vivo (Kodibagkar et al. Citation2008). There is a linear enhancement of longitudinal relaxation rate R1 of fluorine nucleus toward oxygen partial pressure (Parhami and Fung Citation1983), which has been examined for many fluorine compounds in phantoms and in vivo (Mason et al. Citation1996, Sotak et al. Citation1993). Due to their immiscibility in water, these compounds are administered as an emulsion or recently as nanoparticles (Winter Citation2014).
Some inherent properties of 19F make it desirable as an in vivo MR probe. Its sensitivity reaches 83% of that of protons at the same field strength and its natural abundance is 100% since it is the only stable isotope. As a result, the signal magnitude is comparable to that of protons. There is little or no background noise from naturally occurring biological fluorine. As a consequence only dispensed fluorine contributes to the signal. Above all, the biocompatibility of many perfluorocarbons (PFCs) has been proven (Northoff et al. Citation1992). This allows the administration of high concentrations of these compounds to increase sensitivity. Mapping R1 of PFCs with 19F-MRI is a method for directly monitoring the heterogeneous distribution of oxygen partial pressure. The most effective approach suggested so far is a direct intratumoral delivery of reporter molecules (Tatum et al. Citation2006).
Nevertheless, PFCs and 19F-MRI are not in common use due to technical problems. Many PFCs contain several resonances that can cause serious chemical shift artifacts in 19F-imaging. This can be prevented by frequency selective excitation of only one separated single resonance, although this leads to the suppression of some signals from fluorine nuclei.
Semifluorinated alkanes (SFAs) are colorless, non-aqueous liquids consisting of diblock molecules with fluorocarbon (RF) and hydrocarbon (RH) segments with chemical structure F(CF2)m-(CH2)nH. The nomenclature is simplified in FmHn, where m and n describe the number of carbon atoms in the fluorocarbon or the hydrocarbon chain, respectively. At first sight, physicochemical properties of SFAs are similar to those of PFCs, e.g., strong intramolecular bonds, weak intermolecular interactions, high gas-dissolving capacities and low surface tensions. Similar to PFCs, SFAs are considered to be extremely hydrophobic, also are thermally, chemically and biologically inert (Meinert and Knoblich Citation1993, Riess and Krafft Citation1998) In addition to their lower densities, PFCs are extremely hydro- and lipophobic, whereas SFAs are amphiphilic compounds that are characterized by a hydrophobic and lipophobic RF-segment and a hydrophobic and lipophilic RH-segment. The ratio of the RF- and RH-segments defines their physicochemical properties (Le et al. Citation1996, Meinert and Roy Citation2000). Biocompatibility of SFAs appears to be dependent on their lipophilic behavior and on the molecular dimension of the SFA used (Mackiewicz et al. Citation2007, Tsagogiorgas et al. Citation2010).
This property allows the production of stable emulsions for simplified administration in clinical settings. The 19F-T1 does not depend on the emulsion constituents (Thomas et al. Citation1994). Some SFAs like perfluorohexyloctane (F6H8) are already approved for ophthalmological purpose in humans. The purpose of this work is to investigate the properties of this clinically approved compound for the determination of hypoxia with 19F-MRI.
Materials and methods
SFA samples were studied both as emulsion and as a neat liquid in vitro. Three different pure SFA samples, perfluorobutylpentane (F4H5), perfluoropentyloctane (F4H8) and F6H8 were examined (). F6H8 was investigated additionally as an emulsion in water (ST084). The liquids were obtained by Novaliq (Novaliq GmbH, Heidelberg, Germany). All experiments were performed on a Bruker BioSpec 94/20USR horizontal bore magnet equipped with a gradient system included and operated by ParaVision 6.0 (Bruker, Ettlingen, Germany). An in-house constructed 19F low-pass birdcage RF transmit/receive coil with four legs with a length of 5 cm and an internal diameter of 2.8 cm was used for fluorine imaging.
Figure 1. Molecules of perflourohexyloctane (F6H8, a) and perfluorobutylpentane (F4H5, b) along with perfluoropentyloctane (F4H8, b). The largest resonance with an arbitrary chemical shift of 0 ppm in both 376.67 MHz–19F-spectra is affiliated to the CF3-group. The remaining resonances for F6H8 are at −32.7 ppm, −39.6 ppm, −40.5 ppm, −41.4 ppm and −44.1 ppm and belong to the CF2-components in the molecule. With less CF2-groups, F4H5 and F4H8 show identical spectra with resonances at 0 ppm, −32.7 ppm,−42.4 ppm and −44.1 ppm.
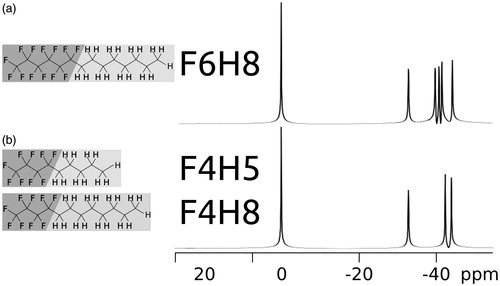
The measurement probes were made of plastic syringes sealed with hot glue. Measurements were made within 6 h after sealing. Each probe was filled with approximately 0.6 ml of liquid. All measurements except temperature curve were performed at room (20 °C) or body temperature (37 °C), with a deviation of less than 0.5 °C. Fixed in a pre-heated water tank, the probes were bubbled with adjustable mixtures of pure oxygen and air at constant temperatures for approximately 15 min after the last detectable change in gas composition. The gas composition during bubbling was continuously monitored with an anesthesiological gas analysis device.
All measurements were performed using a standard slice selective 2D-RARE saturation recovery sequence to determine T1 maps. We used projections perpendicular to the probes with a matrix of 128 × 128, a field of view of 28 × 28 mm2, 2.5 mm slice thickness, 8 averages and a RARE-factor of 8. TEeff was set to 44.8 ms, TR to 4500 ms, bandwidth to 16 kHz to acquire only the signal of the CF3 group (∼25 min). Shimming was performed on the 19F-signal of the CF3-group.
In order to examine the suitability of SFAs for 19F oxygen mapping, the spectrum was determined and the influence of oxygen concentration, temperature and pH on R1 was investigated. A calibration to convert R1 values to pO2 was thereafter derived. Corresponding to the methodology of 19F oximetry published so far, only the resonance of CF3 was observed in detail for each SFA. In the case of physiological conditions, utilization of CF3-resonance should have smallest perturbations from temperature (Shukla et al. Citation1995).
For the purpose of comparison with reference values, we measured two samples of hexafluorobenzene (HFB) with the described method. HFB and two additional samples of F6H8 were bubbled at 20 °C with 21% O2 and 100% O2.
The longitudinal relaxation rate of PFCs is reported to be oxygen tension and temperature-dependent (Mason et al. Citation1993). Samples of F6H8 were equilibrated at five different oxygen concentrations, prepared from pure bottled oxygen and bottled air. The investigated oxygen concentrations were 21/40/60/80 and 100% O2 under atmospheric pressure at temperatures of 23.2/26.0/28.8/32.2/35.0/36.6/39.0 and 42.4 °C. The pO2 was calculated using standard partial pressure equations taking into account the barometric pressure and oxygen percentage by using the approximate conversion 100%atm O2 = 760 mmHg (1013 hPa). The temperature was maintained during imaging using a temperature-adjustable water circulation and a fiber optical temperature sensor located between the probes. The data were then fitted to EquationEq. (1)(1) (Liu et al. Citation2011):
(1)
where R1 is the longitudinal relaxation rate and pO2 is the oxygen pressure in the sample. For given magnetic field and temperature, A and B are constant. Both A and B exhibit a linear dependence with temperature T (Barker et al. Citation1994, Liu et al. Citation2011), then A = C + D ċ T and B = E + F ċ T, with constant C, D, E and F. EquationEquation (2)(2) as a combination of these values and EquationEq. (1)
(1) can be interpreted as a temperature-dependent model:
(2)
The possible influence of pH on R1 was also investigated. Keeping the temperature and oxygen partial pressure constant at 20 °C and 21% O2, respectively, the effect of a change in pH on R1 was studied by measurement at 7 pH values (1.7/2.7/3.8/6.1/11.6/11.9/12.6). The adjustment of the pH value was achieved by the addition of HCl and NaOH to the standard ST084 sample. Changing the pH value did not lead to a decomposition of the emulsion.
Results
The spectrum of F6H8 has six 19F resonances, which are well resolved at 376.67 MHz as shown in . The largest resonance with an arbitrary chemical shift of 0 parts per million (ppm) in the 19F-spectrum is the CF3-group. Other resonances occur at −32.7 ppm, −39.6 ppm, −40.6 ppm, −41.4 ppm and −44.1 ppm and belong to the CF2-components in the molecule. The indistinguishable 19F-spectra of F4H5 and F4H8 in shows resonances at 0 ppm, −32.7 ppm, −42.4 ppm and −44.1 ppm.
shows the relationship between R1 and pO2 for F6H8, F4H8 and F4H5 at physiological temperature. Each data point represents the mean R1 and standard deviation obtained with ParaVision 6 (Bruker) from the pixels in the R1 map corresponding to the vial with the appropriate pO2. R1 versus pO2 relation of HFB and F6H8 at 20 °C are also displayed in that figure to permit comparability with references. Note that due to the change in the structure of the molecule, the observed shift of R1 at 36.5 °C from F6H8 to F4H8 is almost identical to the shift from F4H8 to F4H5. shows the linear relationship of R1 and temperature at five different oxygen levels for F6H8. The parameters of the calibration curves of F6H8 pure and emulsified as ST084 did not show any differences within the error margin, while slope and intercept differ for various substances.
Figure 2. Linear regression of longitudinal relaxation rate R1 of F6H8, F4H8 and F4H5 versus oxygen partial pressure pO2 at physiological temperature (37 °C). Additionally, HFB and F6H8 at 20 °C.
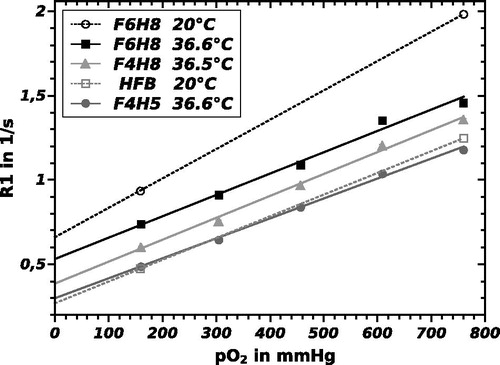
The calculated sensitivity index (representing the sensitivity of each probe toward changes in pO2) in units of 10−3 mmHg−1, defined as B/A (= slope/intercept) in an R1 = A + B ċ pO2 calibration curve, is 2.33 for F6H8, 3.42 for F4H8 and 3.98 for F4H5 at 9.4 T and 37 °C. As to be seen in , slope and intercept of the calibration curve decline for increasing temperature. The calibration curve obeys the EquationEq. (2)(2) with parameters from .
Table I. Comparison of temperature and pO2 coefficients for various fluorine compounds for the relation [mmHg].
Table II. Coefficients at physiological temperature (37 °C) for the relation R1[1/s] = A + B·pO2 [mmHg].
A plot of R1 versus pH is shown in . The fit curve of the pH-measurement can be described as:
Discussion
The aim of this study was to explore the suitability of SFAs as reporter probes for 19F oximetry. Therefore, we measured longitudinal relaxation rate (R1) at given oxygen partial pressure (pO2) and temperature (T) for three SFAs in vitro using a magnetic field of 9.4 T. In vitro studies are reported to be comparable to measurements in vivo (Mason et al. Citation1993). From our measurements, it is possible to determine the constants A–F from EquationEqs. (1)(1) and Equation(2)
(2) . If any two of the three parameters (pO2, T and R1) are known, the third is easily determined from EquationEq. (2)
(2) and without additional calibration.
The SFAs used in this study are highly sensitive to pO2. In contrast to HFB and Perfluoro-15-crown-5-ether (PFCE), both with only one single resonance (Mignion et al. Citation2013), the spectra of SFAs present one single separated CF3-resonance and several CF2-resonances, depending on the amount of CF2-groups contained in the molecule. The spectrum of F6H8 is highly comparable with the spectrum of perfluorooctylbromid (PFOB), which shows only one additional resonance (Mason et al. Citation1992).
Nevertheless, 19F-oximetry needs a well-separated single signal like CF3-resonance (Bellemann et al. Citation2002, Giraudeau et al. Citation2010, Wolters et al. Citation2013) to avoid chemical shift artifacts that result in ghosting.
Calibration results showed that the longitudinal relaxation rate R1 of F4H5, F4H8 and F6H8 are linearly dependent on pO2 at the physiological temperature of 37 °C. We also proved the feasibility of the temperature-dependent model.
Consistent with the behavior of other PFCs, the longitudinal relaxation rate of the three SFA examined is a linear function of dissolved oxygen concentration (). As with other PFCs, R1 was also found to be dependent on temperature. As a consequence, EquationEq. (2)(2) is a function of both temperature and dissolved oxygen. The change in R1 is related to paramagnetic molecular oxygen, adjacent to the fluorine nuclei in the PFCs (Parhami and Fung Citation1983). At any temperature, the longitudinal relaxation rate R1 of the CF3-group of each SFA was linear with pO2. Since the vapor pressure of the SFA used accounts for only a very small percentage of atmospheric pressure (e.g., <1 mmHg for F6H8 at 25 °C and 25.1 mmHg for F4H5 at 37 °C (Tsagogiorgas et al. Citation2010)), it was not considered in the pO2 calibration. The vapor pressure of water is ∼40 mmHg at 37 °C and was also neglected.
The parameters slope and intercept () are comparable to literature values of HFB and PFOB, although these were measured at different field strength. Sensitivity index of F6H8 (2.64) is ca. 55% of the corresponding value of HFB (4.79) at 20 °C. With its negligible dependence on temperature and a generally low dependence on the magnetic field (Mason et al. Citation1996), sensitivity index of HFB measured at 37 °C and 11.7 T is equivalent (4.76) (Mignion et al. Citation2013). Nevertheless, our measured sensitivity for HFB is smaller or equal magnitude to values given in references (). As a result, in terms of sensitivity F6H8 is less suitable for oximetry than HFB by a factor of at least 2.
Compared to other PFC used in oximetry, the SFA examined show higher sensitivity to changes in temperature. The error introduced into a pO2 determination by a 1 °C error in temperature estimate ranges from 8 mmHg/ °C for PFTB to 0.1 mmHg/ °C for HFB (Tressaund, Citation2008) while F6H8 shows 13.6, F4H8 5.8 and F4H5 6.6 mmHg/ °C, respectively. The stated values were calculated at a temperature of 37 °C. Temperature sensitivity declines with decreasing temperature. Compared to HFB (Mason et al. Citation1996), the difference is two orders of magnitude () and could hamper clinical use as a hypoxia marker without thermal surveillance. On the other hand, this increased sensitivity predestines F6H8 for temperature monitoring.
The slope B of a linear fit of R1 over pH is constant and approximately 0 within the error margin. In that case, R1 and pH do not correlate or the correlation is negligible. R1 is thus independent of the pH value, SFAs are therefore not suitable as pH indicators.
Although PFCs are described as chemically inert and stable, long-term tissue toxicity effects have been reported for HFB and PFCE (Mignion et al. Citation2013). Some SFAs are already approved for human use in ophthalmology or have been successfully examined in pulmonology (Tsagogiorgas et al. Citation2010). F6H8 as emulsion used for drug delivery was well tolerated after multiple intravenous injections in rats (Tsagogiorgas et al. Citation2015).
The ST084 emulsion did not decompose for more than 6 months during the realization of this study. The distribution in vivo may differ from ideal distribution in phantom. Nevertheless, in vitro experiments are a prerequisite for in vivo measurements.
Conclusion
The linear relationship between R1 and pO2 for the 19F resonances of fluorinated molecules is well established and is now also verified for SFAs. In this study, we have demonstrated the feasibility of 19F-oximetry with SFAs, primarily F6H8 as a neat liquid and emulsified as ST084. Our determined calibration factors are the prerequisite for non-invasive oxygen tension measurements in vivo using 19F MRI.
Acknowledgements
We gratefully thank Novaliq GmbH, Heidelberg, Germany for supplies of all SFA samples examined.
Declaration of interest
This work was supported by the Federal Ministry of Education of Research (02NUK008F). The funders had no role in study design, data collection and analysis, decision to publish or preparation of the manuscript. The authors report no declarations of interest. The authors alone are responsible for the content and writing of the paper.
References
- Barker BR, Mason RP, Bansal N, Peshock RM. 1994. Oxygen tension mapping with F-19 echo-planar MR imaging of sequestered perfluorocarbon. J Magn Reson Imaging. 4:595–602.
- Bellemann ME, Brückner J, Peschke P, Brix G, Mason RP. 2002. Quantification and visualization of oxygen partial pressure in vivo by 19F NMR imaging of perfluorocarbons. Biomed Tech (Berl). 47:451–454.
- Dardzinski BJ, Sotak CH. 1994. Rapid tissue oxygen tension mapping using 19F inversion-recovery echo-planar imaging of perfluoro-15-crown-5-ether. Magn Reson Med. 32:88–97.
- Domsch S, Mie MB, Wenz F, Schad LR. 2014. Non-invasive multiparametric qBOLD approach for robust mapping of the oxygen extraction fraction. Z Med Phys. 24:231–242.
- Giraudeau C, Flament J, Marty B, Boumezbeur F, Meriaux S, Robic C, et al. 2010. A new paradigm for high-sensitivity 19F magnetic resonance imaging of perfluorooctylbromide. Magn Reson Med. 63:1119–1124.
- Graves EE, Giaccia AJ. 2007. Imaging tumoral hypoxia: oxygen concentrations and beyond. Oncology (Williston Park). 21:368–376 (discussion 377–378, 384).
- Horsman MR, Mortensen LS, Petersen JB, Busk M, Overgaard J. 2012. Imaging hypoxia to improve radiotherapy outcome. Nat Rev Clin Oncol. 9:674–687.
- Kodibagkar VD, Wang X, Mason RP. 2008. Physical principles of quantitative nuclear magnetic resonance oximetry. Front Biosci. 13:1371–1384.
- Le TD, Arlauskas RA, Weers JG. 1996. Characterization of the lipophilicity of fluorocarbon derivatives containing halogens or hydrocarbon blocks. J Fluor Chem. 78:155–163.
- Liu S, Shah SJ, Wilmes LJ, Feiner J, Kodibagkar VD, Wendland MF, et al. 2011. Quantitative tissue oxygen measurement in multiple organs using 19F MRI in a rat model. Magn Reson Med. 66:1722–1730.
- Mackiewicz J, Mühling B, Hiebl W, Meinert H, Maaijwee K, Kociok N, et al. 2007. In vivo retinal tolerance of various heavy silicone oils. Invest Ophthalmol Vis Sci. 48:1873.
- Mason RP, Rodbumrung W, Antich PP. 1996. Hexafluorobenzene: a sensitive 19F NMR indicator of tumor oxygenation. NMR Biomed. 9:125–134.
- Mason RP, Shukla H, Antich PP. 1993. In vivo oxygen tension and temperature: simultaneous determination using 19F NMR spectroscopy of perfluorocarbon. Magn Reson Med. 29:296–302.
- Mason RP, Shukla H, Antich PP. 1992. Oxygent: a novel probe of tissue oxygen tension. Biomater Artif Cells Immobilization Biotechnol. 20:929–932.
- Meinert H, Knoblich A. 1993. The use of semifluorinated alkanes in blood-substitutes. Biomater Artif Cells Immobilization Biotechnol. 21:583–595.
- Meinert H, Roy T. 2000. Semifluorinated alkanes – a new class of compounds with outstanding properties for use in ophthalmology. Eur J Ophthalmol. 10:189–197.
- Mignion L, Magat J, Schakman O, Marbaix E, Gallez B, Jordan BF. 2013. Hexafluorobenzene in comparison with perfluoro-15-crown-5-ether for repeated monitoring of oxygenation using 19F MRI in a mouse model. Magn Reson Med. 69:248–254.
- Northoff H, Haidmann L, Hänsch G, Reuter P, Mader J, Meinert H, 1992. Biocompatibility of synthetic oxygen carriers and fluorosurfactants. Infusionstherapie. 19:115–120.
- Padhani AR, Krohn KA, Lewis JS, Alber M. 2007. Imaging oxygenation of human tumours. Eur Radiol. 17:861–872.
- Parhami P, Fung BM. 1983. Fluorine-19 relaxation study of perfluoro chemicals as oxygen carriers. J Phys Chem. 87:1928–1931.
- Riess JG, Krafft MP. 1998. Fluorinated materials for in vivo oxygen transport (blood substitutes), diagnosis and drug delivery. Biomaterials. 19:1529–1539.
- Shukla HP, Mason RP, Woessner DE, Antich PP. 1995. A comparison of three commercial perfluorocarbon emulsions as high-field 19F NMR probes of oxygen tension and temperature. J Magn Reson B. 106:131–141.
- Sotak CH, Hees PS, Huang HN, Hung MH, Krespan CG, Raynolds S. 1993. A new perfluorocarbon for use in fluorine-19 magnetic resonance imaging and spectroscopy. Magn Reson Med. 29:188–195.
- Tatum JL, Kelloff GJ, Gillies RJ, Arbeit JM, Brown JM, Chao KSC, et al. 2006. Hypoxia: importance in tumor biology, noninvasive measurement by imaging, and value of its measurement in the management of cancer therapy. Int J Radiat Biol. 82:699–757.
- Thomas SR, Pratt RG, Millard RW, Samaratunga RC, Shiferaw Y, Clark LC, Hoffmann RE. 1994. Evaluation of the influence of the aqueous phase bioconstituent environment on the F-19 T1 of perfluorocarbon blood substitute emulsions. J Magn Reson Imaging. 4:631–635.
- Tressaud A, Günter H. 2008. Fluorine and health molecular imaging, biomedical materials and pharmaceuticals. Amsterdam Boston: Elsevier Science, 2008, ISBN 9780080558110.
- Tsagogiorgas C, Krebs J, Pukelsheim M, Beck G, Yard B, Theisinger B, Quintel M, Luecke T. 2010. Semifluorinated alkanes – a new class of excipients suitable for pulmonary drug delivery. Eur J Pharm Biopharm. 76:75–82.
- Tsagogiorgas C, Theisinger S, Heesch E, Krebs J, Holm R, Beck G, Yard B. 2015. Evaluation of pharmacokinetic properties and anaesthetic effects of propofol in a new perfluorohexyloctane (F6H8) emulsion in rats – a comparative study. Int J Pharm. 486:69–76.
- Winter PM. 2014. Perfluorocarbon nanoparticles: evolution of a multimodality and multifunctional imaging agent. Scientifica (Cairo). 2014:746574.
- Wolters M, Mohades SG, Hackeng TM, Post MJ, Kooi ME, Backes WH. 2013. Clinical perspectives of hybrid proton-fluorine magnetic resonance imaging and spectroscopy. Invest Radiol. 48:341–350.