Abstract
Wound healing is a complex process affected by several factors. In the present work, novel biocompatible PLGA–curcumin microparticle-embedded chitosan scaffold was fabricated for wound-healing application. Process variables involved in the preparation of microparticles were optimized using design of experiment. Scanning electron microscopy (SEM) confirmed the porous nature of scaffold with embedded microparticles. A maximum release of 14% of the encapsulated curcumin was observed at 12th hour. Modified tube dilution method showed that scaffold significantly (p < 0.05) reduced multiplication of Staphylococcus aureus. More than 50% of the excised wound in rats healed in 4 days with an epithilization period of 18 days.
Introduction
Healing from wounds requires participation of various phases of tissue remodeling. A number of factors delays wound healing. Microbial infection at the injured site is one of the primary reasons for the delay in wound healing (Guo and Dipietro Citation2010). Unprotected wounds are prone to microbial infections that affect different phases of healing process and ultimately delays or prevent wound healing (Robson Citation1997). Wound-dressing materials prepared using biodegradable polymer scaffold hastens wound healing. Chitosan is a natural, nontoxic, biodegradable polymer mainly derived from a marine source that has potential antimicrobial property (Kong et al. Citation2010). Chitosan, when used in the form of wound bandages, accelerates wound healing. “HemCon,” a chitosan acetate bandage is effective in treating third degree burns (Dai et al. Citation2009).
Scaffold fabricated from chitosan is biocompatible and safe in animals (VandeVord et al. Citation2002). Chitosan, a positively charged polymer, due to its muco-adhesive, hemostatic, biodegradability, and antimicrobial properties, is extensively studied in tissue engineering application (Hajiabbas et al. Citation2015). Chitosan, in the form of three-dimensional (3D) and two-dimensional (2D) scaffolds, is highly effective as a wound-dressing material (Croisier and Jérôme Citation2013). Physical properties, such as porosity and mechanical strength, of chitosan-based scaffolds can be altered by suitably modifying the preparation of chitosan scaffolds. Besides a suitable variation would make chitosan scaffold appropriate for various biomedical applications (Gao et al. Citation2012). Chitosan-based scaffolds could be used as a drug delivery system if therapeutic agents, such as antimicrobial and anti-inflammatory agents, are adsorbed on the surface of the chitosan scaffolds in such a way that these agents are released for an extended time. Gold nanoparticle-bound chitosan has better biocompatibility than the individual gold nanoparticle (Tan and Zhang Citation2005). Antibiotics, such as doxycycline, vancomycin, tetracycline, and gentamicin have been delivered using scaffolds (Garg et al. Citation2012). However, preparation of these scaffolds that could be used as dual-purpose system, viz.,, tissue engineering and drug delivery system is challenging (Mozafari Citation2014). The affinity between the scaffolds and the therapeutic agents should be strong enough such that they remain attached during storage and when used as dressing materials. At the same time, the bound drug should be released in a sustained manner, so that the efficacy of the drug benefits the patient. In addition, the porous and adsorption properties of the chitosan scaffolds should not be affected (Garg et al. Citation2012).
Curcumin, a natural polyphenol from turmeric, has many pharmacological properties and traditionally used in many ailments (Kasinathan et al. Citation2015b). It has potential antimicrobial and wound-healing activity, including in the treatment of severe burn wounds (El-Refaie et al. Citation2015). It is effective in controlling the growth of bacteria, virus, fungi, and parasites and traditionally used for treating various ailments in humans (Akbik et al. Citation2014). However, curcumin is light sensitive and this limits the use of plain curcumin on the skin exposed to direct sunlight.
The present work focuses on the development of curcumin microparticles incorporated chitosan scaffold as a multipurpose drug delivery system for wound-healing application. The antimicrobial wound-healing drug would be released in a sustained manner from the microparticles, and at the same time, the desired cell culture techniques could be employed in the scaffold system for tissue regeneration in treating severe skin chronic and burn wounds. Due to simultaneous drug release and tissue regeneration from the microparticle-embedded scaffold, the healing of chronic and burn wounds would be faster. Moreover, the microparticle-embedded scaffold protects the light and pH-sensitive curcumin and releases the curcumin in a sustained manner for effective wound healing. Due to the inherent antimicrobial properties of chitosan and curcumin, the wound is protected from the possible microbial infection, thereby accelerating the rate of wound healing by a protective covering of wounds. Suitable modification in the curcumin microparticle-embedded scaffold with desired cell culture technique would make a multipurpose system with enhanced therapeutic potential.
Materials and methods
Materials
Deactylated chitosan (85%), Dulbecco’s Modified Eagle’s Medium (DMEM) and tetrazolium salt (MTT) were procured from Sigma (Bangalore, India). Fetal bovine serum (FBS) was purchased from Invitrogen (Carlsbad, CA). Curcumin (95% purity) was obtained from Venbiotech Pvt Ltd (Chennai, India). PLGA 50:50 (Resomer, 503H) was purchased from Boehringer Ingelheim Pharma GmbH & Co., Ingelheim, Germany. HPLC-grade methanol and other analytical grade solvents and fine chemicals were purchased from Merck (Mumbai, India). The cell line viz., Vero was procured from National Center for Cell Science, Pune, India. Staphylococcus aureus (NCIM 2079) was procured from the National Collection of Industrial Microorganisms, Pune, India.
Preparation of PLGA–curcumin microparticles and optimization
PLGA–curcumin microparticles were prepared by microprecipitation method described previously (Li et al. Citation2007) with little modification. Briefly, curcumin and PLGA were dissolved in acetone and added slowly into 1% polyvinyl alcohol (PVA) solution with high-speed homogenization (Polytron, PT 1300D, Kinematica, Luzen, Switzerland). After homogenization, the dispersion was processed in a rotary evaporator at 35 °C for 30 min to remove the organic solvent. Once the organic solvent was removed, the whole dispersion was centrifuged at 8500 rcf for 30 min at 4 °C. The yellow-colored precipitate settled at the bottom of the centrifuge tube was collected, washed three times with water and resuspended in purified water. The process parameters involved in the preparation of PLGA–curcumin microparticles were optimized by two-level full factorial design (Minitab-16 trial version, Coventry, UK).
The effect of three independent variable drug–polymer ratio (DPR), homogenization speed (HS) and homogenization time (HT) on mean particle size (MPS), polydispersity index (PDI), zeta potential (ZP), and percentage drug content (% DC) was analyzed using two-level full factorial design. The design matrix consisted of eight trials with single block and zero center points ().
Table 1. Factorial design study showing the effect of process variables on particle size and drug content.
Particle size and zeta potential measurement of PLGA–curcumin microparticles
The particle size and zeta potential of PLGA–curcumin microparticles were measured by dynamic light scattering method (Malvern Zetasizer, Malvern, UK) at 25 °C.
Drug content
The amount of curcumin in PLGA microparticles was determined by digesting required quantity of microparticles in acetonitrile followed by addition of HPLC mobile phase (pH 3.5 phosphate buffer) with 15-min sonication. The digested microparticles suspension was centrifuged at 8500 rcf for 30 min and the yellow colored supernatant was analyzed for drug content by RP-HPLC method (Shimadzu, LC-2010C HT, Kyoto, Japan) at 424 nm. The chromatographic conditions used for drug content measurement were Luna C18 (250 mm × 4.6 mm, 5 μm) column and the mobile phase flow rate was maintained at 0.8 mL/min. The column temperature was kept at 25 °C throughout the measurement period.
Synthesis of chitosan HCl and fabrication of chitosan scaffold
Chitosan HCl was synthesized by the method described previously (Signini and Campana Filho Citation1999). Briefly, crude chitosan (2 g) was dissolved in 100 mL of acetic acid solution (2% v/v) by constantly stirring overnight and filtered to remove any insoluble residues. Then, the filtered polymer solution was exhaustively dialyzed using a dialysis bag (cutoff 12–14 kDa, Himedia, Mumbai, India) against 0.2 mol/L NaCl solution for 48 h and then with ultrapure water for 12 h.
The above purified chitosan HCl polymer solution was freeze-dried using lyophilizer (LabTech freeze drier, Kyeonggi-do, South Korea) for 48 h. After lyophilization, the chitosan scaffold was taken out and stabilized first in 0.1 N NaOH followed by 95% ethanol. The stabilized chitosan scaffold was air-dried at room temperature and stored in a desiccator until further use.
Embedding of PLGA–curcumin microparticles on the scaffold
Accurately weighed dry scaffold was taken in a Petri dish and PLGA–curcumin microparticles dispersion (equivalent to 50 μg of curcumin, N6 of ) in purified water was added onto the scaffold and slowly dried at room temperature.
Scanning electron microscopy (SEM) evaluation
The surface and cross-section morphology of the PLGA–curcumin microparticle-embedded chitosan scaffold was examined by using ZEISS scanning electron microscope (SEM) (ZEISS, EVO 18, Oberkochen, Germany).
In vitro drug release from the PLGA microparticle-embedded chitosan scaffold
Accurately weighed PLGA–curcumin microparticle-embedded scaffold was immersed in 100 mL of pH 7.4 phosphate-buffered saline (PBS) containing polysorbate 60 (1% v/v) (Amirthalingam et al. Citation2015). The PBS medium was stirred at 50 rpm in a water bath shaker at 37 °C. Dissolution samples (0.5 mL) were withdrawn at specified time intervals and the same amount of fresh dissolution medium was replaced to maintain the volume. The dissolution sample was mixed with equal amount of acetonitrile and centrifuged at 3000 rcf for 30 min. The yellow-colored supernatant was taken and mixed with 0.5 mL of mobile phase and the cumulative amount of curcumin released from the scaffold was analyzed by HPLC at 424 nm.
Cell culture with PLGA microparticle-embedded chitosan scaffold
Biocompatibility of the scaffold was studied by seeding Vero cells on presterilized PLGA–curcumin microparticle-embedded scaffold. The scaffold was sterilized by washing once with ethanol, three times with sterile PBS (pH 7.4) and UV treatment for 45 min. The sterilized scaffold was placed in a sterile tissue culture Petri plate and Vero cells (≤1000 cells) were seeded. The seeded scaffold was flooded with DMEM medium enriched with 10% FBS and incubated at 37 °C for 72 h in a CO2 incubator (5% CO2 and 95% humidity). At the end of 72 h incubation, viability, and proliferation of the cells were analyzed qualitatively by transferring the scaffold into a fresh Petri plate. MTT solution (0.5 mL of 2 mg/mL concentration) was added to the scaffold and incubated for 5 h in a CO2 incubator. The change in color was observed.
Antibacterial activity of PLGA–curcumin microparticle-embedded scaffold
Modified tube dilution method
The effectiveness of PLGA–curcumin microparticle-embedded scaffold in inhibiting microbial growth was assessed using the modified tube dilution method (Amirthalingam et al. Citation2015). PLGA–curcumin microparticle-embedded scaffold and plain scaffold (weight equivalent to 100 mg sterilized under UV light) was aseptically transferred into the sterile Mueller Hinton broth (MHB) (2 mL) distributed in a series of test tubes. Staphylococcus aureus culture (50 μL from the stock with transmittance 25%) was added to each of the MHB with and without a scaffold. Antimicrobial activity of PLGA–curcumin microparticle-embedded scaffold was analyzed by measuring the transmittance at 630 nm using colorimeter (Systronics, Ahmedabad, India) at 4, 8, 16, and 28 h against control. PLGA–curcumin microparticle-embedded scaffold was considered to impede the microbial growth if the transmittance of MHB containing PLGA–curcumin microparticle-embedded scaffold was higher than the control.
In vivo wound healing activity of PLGA–curcumin microparticles in rats
Healthy Sprague Dawley rats (180–220 g), bred in the animal house of Kasturba Medical College, Manipal University, India were used for excision wound study. The rats were kept in the controlled conditions (23 ± 2 °C and 50 ± 5% RH) and 10–12 h light and dark cycles, respectively. They were fed with standard rat feed and water ad libitum throughout the study. The study was conducted after obtaining the institutional animal ethical permission (No: IAEC/KMC/57/2014). Excision wound was induced in an overnight fasted animal under ketamine (50 mg/kg, i.m.) anesthesia, by surgically cutting circular wound of 300 mm2 on the dorsal thoracic region of rats. This was considered as wound day (initial day or day 0). Two groups of eight animals (control – no treatment and test sample – 250 μL of PLGA-curcumin microparticles in chitosan HCl solution, N6 of ) in each were treated topically once a day. The wound size was measured by the planimetric measurement in each animal on 0, 4th, 8th, 12th, 16th, 20th, and 22nd postwounding day. This was done by tracing the wound on a transparent paper and then transferring to 1-mm2 graph sheet (Shanbhag et al. Citation2010). The wound contraction rate in percentage was calculated from the initial wound size using the formula;
(WSI: wound size on initial day or day 0; WSS: wound size on specific measurement day)
The time taken for healing the wound about 50% of the initial wound size was considered as WC50 (wound contraction rate, 50%). Skin biopsies for Hematoxylin and Eosin (H&E) staining from two rats in each group were collected on day 12th of the postwounding day. The blinded slides were examined under a light microscope and reported by an experienced pathologist from our university. The skin epithelialization period (EP) was noted when wound completely healed without leaving eschar. All values were expressed as mean ± SEM. The data were analyzed using analysis of variance (ANOVA) (P < 0.05 considered statistically significant) followed by Dunnett’s test.
Results and discussion
PLGA–curcumin microparticles preparation and optimization
The PLGA–curcumin microparticles were prepared and optimized in 1% PVA dispersion medium using high-speed homogenizer. The PLGA–curcumin microparticles were formed immediately upon addition into the dispersion medium and simultaneously homogenized to form microparticles. The microparticles preparation method was optimized using full factorial design experiments and eight microparticles formulations were prepared by studying the effect of the drug to polymer ratio, homogenization speed, and homogenization time on drug content and particle size.
Effect on mean particle size
Evaluation of main effects () showed that the independent variables had a significant effect on the mean particle size. The mean particle size reduced with an increase in the level of the independent variables. Two-way ANOVA showed that the interaction between the two variables increased the mean particle size. However, interaction between all the three variables reduced the mean particle size.
Figure 1. (a) Main effect plot showing the effect of individual process variables on the mean particle size. (b) An interaction plot showing the effect of process variables on the mean particle size.
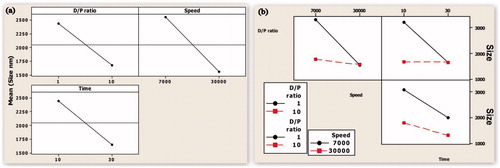
Study on interaction () showed that when the DPR was maintained at its lowest level and time 20 min, the mean particle size is significantly affected, that is, the MPS reduced when the speed is increased from 7000 to 30 000 ().
Figure 2. (a) Contour plot showing the effect of lower drug–polymer ratio on mean particle size. (b) Contour plot showing the effect of higher drug–polymer ratio on mean particle size.
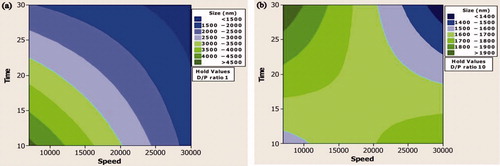
However, when the level of DPR was 10, the change in speed does not play a significant role and there was not a significant change in the MPS. Similarly, when the speed is maintained close to 18 000 RPM, the homogenization time had a significant effect only when DPR was maintained at 1. The role of time on MPS became insignificant when the level of DPR was 10 (). Interaction between speed and time showed that when the level of DPR was 5.5 and speed 7000, an increase in time from 10 to 30 min reduced the MPS from > 3000 to approximately 2000 nm. When DPR was 5.5 and speed 30 000, the MPS reduced from close to 2000 nm to 1000 nm.
Effect on PDI
A study on the main effect showed that DPR had less influence on the PDI and HT had maximum effect on the PDI (). Two-way interaction showed that interaction between DPR and HT affected more than 50% of the outcome (). At a constant HT, the interaction between DPR and HS did not affect the outcome much, although a slight increase in PDI could be observed. When the HS was maintained at 20 min and DPR at 1, PDI decreased from greater than 0.5 to less than 0.3 when the HT increased from 10 to 30 min. However, when the HS was maintained at 20 min and DPR at 10 PDI slightly increased from a value of less than 0.4 to value close to 0.5. At a constant DPR of 5.5, the interaction between HS and HT did not affect the outcome much although a slight fall in PDI was observed.
Table 2. The effect of process variables on size, zeta potential and drug content.
Effect on zeta potential
Among the independent variables, 30% of the outcome (zeta potential) was affected by the HS (). Zeta potential decreased as the HS increased. HT had the least influence on the zeta potential. At both low and high levels of DPR, a decrease in ZP was observed when speed was increased from 7000 to 30 000 rpm when HT was 20 min. However, the decrease was more prominent when the DPR was 10. When HS was maintained at 18 500 rpm and DPR 1, a slight increase in ZP was observed when the HT increased from 10 to 30 min and very small reduction in ZP is observed when HS is 18 500 rpm and DPR 10. However, the interaction between HS and HT had a significant effect on the ZP when the level of DPR was maintained constant, that is, 5.5. At 7000 rpm, ZP increase with increasing HT and at 30 000 rpm, ZP decreased with increasing HT.
Effect on % DC
One-way ANOVA showed that among the three independent variables DPR had maximum effect on drug encapsulation with % DC increases with increase in DPR (). The 70% of the outcome, that is, % DC was dependent on DPR (). Although the speed as a single variable did not have a significant effect on % DC, the increase in speed reduced % DC. However, HT did not have any significant effect on % DC. Due to quick microprecipitation of polymer–drug solution in the stabilizer, the curcumin-encapsulated PLGA microparticles were formed immediately upon addition. Therefore, exposure to higher duration would not affect the encapsulation. However, long HT is required to reduce the MPS.
Figure 3. (a) Main effect plot showing the effect of individual process variables on drug content. (b) An interaction plot showing the effect of process variables on drug content.
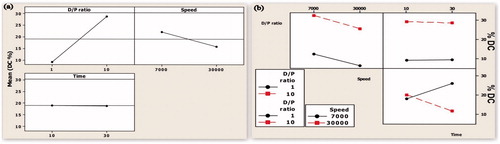
Study on interaction () showed that when the effect of DPR on % DC was dependent on its interaction with HS when HT was maintained at 20 min. At both low and high DPR levels, the % DC reduced with increasing speed. However, when the speed was maintained at a constant level, the interaction between DPR and time did not have any effect on % DC. When the level of DPR was maintained at 5.5 and speed at 7000 rpm, % DC increased when HT was increased from 10 to 30 min, However, % DC decreased with increasing HT when the speed was maintained at 30 000 rpm.
With the implementation of ICHHT-2009 guideline, it has become mandatory for the researchers to analyze and identify the importance and level of process variables involved in the formulation of a drug (Guideline Citation2009, Narayanan et al. Citation2014). In the present study, the effect of variables involved in the preparation of curcumin-loaded PLGA microparticles was studied using factorial design. The study showed that maximum drug encapsulation with optimum particle size could be achieved when the level of DP ratio, HS and HT was 10%, 7000 rpm and 30 min, respectively (). Therefore, further studies were carried out using the microparticles prepared under the conditions indicated earlier. Based on this optimization study, the formulation N6 was taken for in vivo evaluation, scaffold fabrication and antimicrobial activity.
Fabrication and SEM evaluation of PLGA–curcumin microparticle-embedded chitosan scaffold
The chitosan HCl was synthesized and dialyzed against NaCl. The lyophilized powder was white in color and soluble in water. The chitosan scaffold was fabricated by freeze-drying method followed by stabilization with NaOH and ethanol. The SEM image showed that the lyophilized scaffold was highly porous in nature. The cross-sectional SEM image showed clear interconnected pores in the chitosan scaffolds. The physical incorporation of the PLGA–curcumin microparticles in the scaffold was evident from the SEM images and the microparticles were able to attach in the scaffold layers firmly ().
Figure 5. SEM images of PLGA–curcumin microparticle-embedded chitosan scaffold. (a) Cross-section view of PLGA–curcumin microparticle-embedded chitosan scaffold showing clear interconnected pores. (b) Cross-section view of the scaffold (at 1.00 K × magnification) showing the layers. (c) Cross-section view of the scaffold (at 2.00 K × magnification) showing the microparticles in the scaffold layer. d) Cross-section view of the scaffold in another location (at 1.00 K × magnification) showing the layers. (e) Cross-section view of the scaffold (at 10.0 K × magnification) showing the microparticles in the scaffold layer. (f) Individual PLGA–curcumin microparticle-embedded in the chitosan scaffold layer (at 10.0 K × magnification).
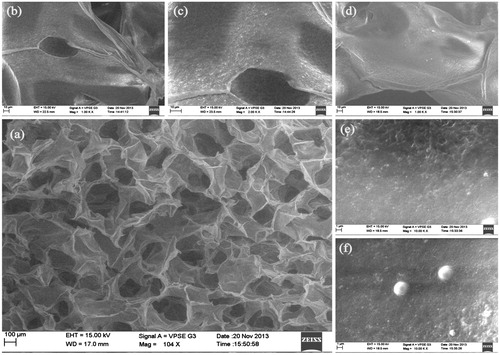
Controlled freeze- and lyophilization-based method is useful in preparing reproducible porous scaffolds that could be used for a variety of applications (Nwe et al. Citation2009). Porous scaffolds are particularly required in tissue engineering to achieve high cell density during culturing of mammalian cells. In the present study, porous chitosan scaffolds were prepared using freeze-drying method with ethanol as stabilizing solvent. Choice of solvent is a particular concern for the drugs with human applications as traces of any toxic solvent can create health hazards (Ho et al. Citation2004). Ethanol is a class 3 solvent that is considered relatively safe.
In vitro drug release from PLGA–curcumin microparticle-embedded scaffold
The in vitro drug release study of PLGA–curcumin microparticle-embedded scaffold showed a slow release profile in the surfactant containing PBS (pH 7.4) dissolution medium. The release of curcumin from the scaffold was sustained with highest release of 14% in 12 h (). These results suggest that the scaffolds could be used as drug delivery systems to release the pharmacological agents effectively.
Cell culture technique
Any formulation intended for human should be safe. In case of scaffolds, the ability of scaffold to support cell viability and proliferation is mandatory as they are mostly used in tissue regeneration. In the present study, the cytotoxicity evaluation of the chitosan scaffold embedded with PLGA–curcumin microparticles were studied using Vero cells as a model cell. MTT assay showed that the cells were viable after 72 h of incubation. The study showed that the PLGA–curcumin microparticle-embedded chitosan scaffold was nontoxic and supports cell growth. This shows that the incorporation of curcumin microparticles on the scaffold did not affect the viability of the Vero cells seeded on the scaffold. The SEM image confirmed that microparticle-embedded scaffold has sufficient pores to support cell growth and proliferation.
Antibacterial property
Presence of microbes at the injured site delays wound healing. The curcumin microparticle-embedded chitosan scaffold showed antimicrobial property against the tested microorganism S.aureus. Among various microbes, S.aureus is an important microorganism causing infection at the injured site (Krausz et al. Citation2015). Therefore, the antimicrobial activity of the drug-loaded scaffold was studied using S.aureus as a representative microorganism. A study on the antimicrobial activity of a scaffold by modified broth dilution method showed that curcumin microparticle-embedded scaffold was able to inhibit the growth S.aureus (). Tukey’s multiple comparisons showed that both plain scaffold and PLGA–curcumin microparticle-embedded chitosan scaffold significantly (P < 0.05) reduced microbial populations. Comparison between the ability of plain chitosan scaffold and PLGA–curcumin microparticle-embedded chitosan scaffold in controlling microbial division showed that PLGA–curcumin microparticle-embedded chitosan scaffold was more potent. The in vivo wound-healing studies in rats showed that the curcumin microparticles were able to heal the wounds significantly in the initial days of wound, where the chances of microbial infections are high. Curcumin is a pleotrophic molecule having good wound healing property (Kasinathan et al. Citation2015a). So, when it is combined with natural polymer like chitosan, the wound-healing action could be significantly increased with maximum protection from the microbial infections.
In vivo wound-healing activity of PLGA–curcumin microparticles in rats
The excision wound-healing study in rats showed significant (P < 0.001 on the 8th day) wound contraction in the PLGA–curcumin microparticles treated groups during the initial days of wound healing compared with the control group. The rate of 50% of the wound to heal from the initial wound size WC50 of the PLGA–curcumin microparticles was about 4 days, whereas in the control group, the WC50 was about 8 days (). The skin epithelialization period (EP) of the PLGA–curcumin microparticles was comparatively efficient than the control animal group (PLGA–curcumin microparticles group 18.16 ± 0.945 and control group 20.66 ± 0.988 days).
Figure 8. Wound contraction rate of PLGA–curcumin microparticles at different time intervals and skin epithelialization period (EP) in SD rats. Values are expressed as mean ± SEM.
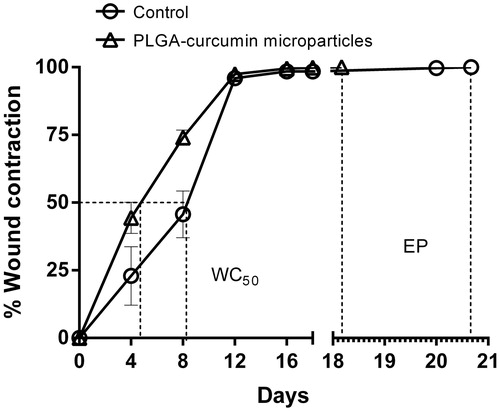
The 12th day histological examination of the wound of control animals showed mild proliferative vessels with chronic inflammatory cells. The PLGA–curcumin microparticles treated group showed good granulation tissue response like proliferation of new vessels than the control group ().
Figure 9. Wound healing and histological evaluation of PLGA–curcumin microparticles in SD rats. (C-0) Control – initial day wound (C-8) Control – 8th day wound (C-12) Control – 12th day wound (at 10× magnification) (C-12x) Control – 12th day wound (at 40× magnification). (T-0) PLGA–curcumin microparticles treated – initial day wound (T-8) PLGA–curcumin microparticles treated – 8th day wound (T-12) PLGA–curcumin microparticles treated - 12th day wound (at 10× magnification) (T-12×) PLGA–curcumin microparticles treated - 12th day wound (at 40× magnification).
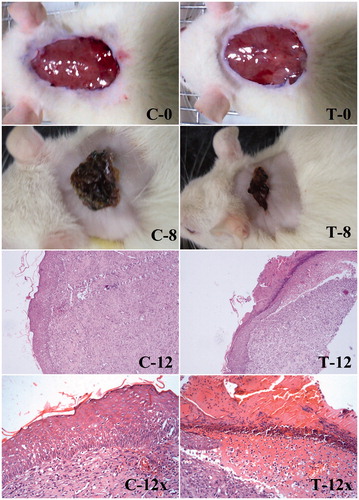
Major skin morphological changes occur in the initial days of wound healing (Vidinský et al. Citation2006). The initial days of wound healing are important, where the chances of infection by pathogenic microorganisms are higher. The open slow healing wound provides a gateway for the microbes to invade the host without much difficulty. Considering this, the PLGA–curcumin microparticles provides faster action by contracting the wound in the initial stage of wound healing compared with the control group. Therefore, the chances of microbial infection at the wound site would be minimal.
Conclusion
In the present study, PLGA–curcumin microparticle-embedded chitosan scaffold was fabricated as a drug delivery system to release the drug curcumin. The in vivo studies on rats showed the PLGA–curcumin microparticles were able to heal the wounds significantly in the initial days of wounds. The PLGA–curcumin microparticle-embedded scaffold was able to impede the growth of microorganisms in the test condition, and it was nontoxic and supported the growth of normal Vero cells. The sustained drug release profile was observed from the PLGA–curcumin microparticle-embedded scaffold. This indicates that this curcumin microparticle-embedded chitosan scaffold could be used as a drug delivery system to treat the severe and chronic wounds. In a further development, an efficient wound-healing system could be developed by growing mesenchymal stem cells on the microparticle-embedded scaffolds for treating severe and chronic wounds. These mesenchymal stem cells grown drug releasing scaffolds could be used as a multipurpose simultaneous system that can be used in drug delivery and in tissue engineering.
Acknowledgements
The authors would like to acknowledge the authorities of Manipal University for providing the necessary facilities required for carrying out this experiment. Our thanks to Dr. Shaila Bhat, Professor and Head, Department of Pathology, Melaka Manipal Medical College, Manipal for histological interpretation.
Disclosure statement
The authors confirm that there are no known conflicts of interest associated with this publication.
References
- Akbik D, Ghadiri M, Chrzanowski W, Rohanizadeh R. 2014. Curcumin as a wound healing agent. Life Sci. 116:1–7.
- Amirthalingam M, Kasinathan N, Mutalik S, Udupa N. 2015. In vitro biocompatibility and release of curcumin from curcumin microcomplex-loaded chitosan scaffold. J Microencapsul. 32:364–371.
- Croisier F, Jérôme C. 2013. Chitosan-based biomaterials for tissue engineering. European Polym J. 49:780–792.
- Dai T, Tegos GP, Burkatovskaya M, Castano AP, Hamblin MR. 2009. Chitosan acetate bandage as a topical antimicrobial dressing for infected burns. Antimicrob Agents Chemother. 53:393–400.
- El-Refaie WM, Elnaggar YS, El-Massik MA, Abdallah OY. 2015. Novel curcumin-loaded gel-core hyaluosomes with promising burn-wound healing potential: development, in-vitro appraisal and in-vivo studies. Int J Pharm. 486:88–98.
- Gao W, Lai JCK, Leung SW. 2012. Functional enhancement of chitosan and nanoparticles in cell culture, tissue engineering, and pharmaceutical applications. Front Physiol. 3:1–13.
- Garg T, Singh O, Arora S, Murthy RSR. 2012. Scaffold: a novel carrier for cell and drug delivery. Crit Rev Ther Drug Carrier Syst. 29:1–63.
- Guideline ICHHT. 2009. Pharmaceutical development. Q8 (R2). As revised in August. 1-24.
- Guo S, Dipietro LA. 2010. Factors affecting wound healing. J Dent Res. 89:219–229.
- Hajiabbas M, Mashayekhan S, Nazaripouya A, Naji M, Hunkeler D, Rajabi Zeleti S, Sharifiaghdas F. 2015. Chitosan-gelatin sheets as scaffolds for muscle tissue engineering. Artif Cells Nanomed Biotechnol. 43:124–132.
- Ho MH, Kuo PY, Hsieh HJ, Hsien TY, Hou LT, Lai JY, Wang DM. 2004. Preparation of porous scaffolds by using freeze-extraction and freeze-gelation methods. Biomaterials. 25:129–138.
- Kasinathan N, Amirthalingam M, Reddy ND, Jagani HV, Volety SM, Rao JV. 2015a. In-situ implant containing PCL-curcumin nanoparticles developed using design of experiments. Drug Deliv. 23:1017–25.
- Kasinathan N, Amirthalingam M, Reddy ND, Vanthi MB, Volety SM, Rao JV. 2015b. Polycaprolactone-based in situ implant containing curcumin-PLGA nanoparticles prepared using the multivariate technique. Artif Cells Nanomed Biotechnol. [Epub ahead of print]. DOI: 10.3109/21691401.2015.1058810.
- Kong M, Chen XG, Xing K, Park HJ. 2010. Antimicrobial properties of chitosan and mode of action: a state of the art review. Int J Food Microbiol Food. 144:51–63.
- Krausz AE, Adler BL, Cabral V, Navati M, Doerner J, Charafeddine RA, et al. 2015. Curcumin-encapsulated nanoparticles as innovative antimicrobial and wound healing agent. Nanomedicine. 11:195–206.
- Li XS, Wang JX, Shen ZG, Zhang PY, Chen JF, Yun J. 2007. Preparation of uniform prednisolone microcrystals by a controlled microprecipitation method. Int J Pharm. 342:26–32.
- Mozafari M. 2014. The critical impact of controlled drug delivery in the future of tissue engineering. Trends Biomater Artif Organs. 28:124–126.
- Narayanan K, Subrahmanyam VM, Rao JV. 2014. Application of experimental design in preparation of nanoliposomes containing hyaluronidase. J Drug Deliv. 2014:1–7.
- Nwe N, Furuike T, Tamura H. 2009. The mechanical and biological properties of chitosan scaffolds for tissue regeneration templates are significantly enhanced by chitosan from Gongronella butleri. Materials. 2:374–398.
- Robson MC. 1997. Wound infection. A failure of wound healing caused by an imbalance of bacteria. Surg Clin North Am. 77:637–650.
- Shanbhag T, Amuthan A, Shenoy S, Sudhakar. 2010. Effect of Phyllanthus niruri. Linn on burn wound in rats. Asian Pac J Trop Med. 3:105–108.
- Signini R, Campana Filho SP. 1999. On the preparation and characterization of chitosan hydrochloride. Polym Bull. 42:159–166.
- Tan WB, Zhang Y. 2005. Surface modification of gold and quantum dot nanoparticles with chitosan for bioapplications.. J Biomed Mater Res A. 75:56–62.
- VandeVord PJ, Matthew HWT, Desilva SP, Mayton L, Wu B, Wooley PH. 2002. Evaluation of the biocompatibility of a chitosan scaffold in mice. J Biomed Mater Res. 59:585–590.
- Vidinský B, Gál P, Toporcer T, Longauer F, Lenhardt Ľ, Bobrov N, Sabo J. 2006. Histological study of the first seven days of skin wound healing in rats. Acta Veterinaria Brno. 75:197–202.