Abstract
Repair of the bone injury remains a challenge in clinical practices. Recent progress in tissue engineering and therapeutic gene delivery systems have led to promising new strategies for successful acceleration of bone repair process. The aim of this study was to create a controlled-release system to slowly release the arginine–chitosan/plasmid DNA nanoparticles encoding BMP-2 gene (Arg-CS/pBMP-2 NPs), efficiently transfect osteoblastic progenitor cells, secrete functional BMP-2 protein, and promote osteogenic differentiation. In this study, chitosan was conjugated with arginine to generate arginine–chitosan polymer (Arg-CS) for gene delivery. Mix the Arg-CS with pBMP-2 to condense pBMP-2 into nano-sized particles. In vitro transfection assays demonstrated that the transfection efficiency of Arg-CS/pBMP-2 nanoparticles and the expression level of BMP-2 was obviously exceed control groups. Further, PELA microspheres as the controlled-release carrier for the nanoparticles were used to encapsulate Arg-CS/pBMP-2 NPs. We demonstrated that the Arg-CS/pBMP-2 NPs could slowly release from the PELA microspheres at least for 42 d. During the co-culture with the PELA microspheres, the content of BMP-2 protein secreted by MC3T3-E1 reached the peak at 7 d. After 21d, the secretion of BMP-2 protein still maintain a higher level. The alkaline phosphatase activity, alizarin red staining, and osteogenesis-related gene expression by real-time quantitative PCR analysis all showed the PELA microspheres entrapping with Arg-CS/pBMP-2 NPs can obviously induce the osteogenic differentiation. The results indicated that the Arg-CS is a suitable gene vector which can promote the gene transfection. And the novel PELA microspheres-nanoparticle controlled-release system has potential clinical application in the future after further research.
Introduction
Bone defects are caused by a variety of routes including comminuted fracture, bone neoplasms excision, or osteomyelitis debridement. If there is no treatment undertaken in these cases, the patient will experience severe lifestyle impairment. As a gold standard for the treatment of bone defects, autogenous bone transplantation has some limitations such as donor site pain and limited resource. Transplantation of allogeneic bones is an alternative, but it has high medical cost and risks of virus transmission and adverse immune reactions which significantly limit its wide application. Osteoinductive biomaterials are promising to solve these problems and provide an alternative to autogenous bone and allografts. Some growth factors, especially BMP-2, have been proven to promote bone healing (Ji et al. Citation2010). Carriers with growth factors have been applied for the fracture healing and spinal fusion in clinical practice. However, recombinant BMP-2 protein therapy has certain demerits, such as large dose requirements, high cost and short half-life (Faensen et al. Citation2011, Jin et al. Citation2014, Osawa et al. Citation2010, Wegman et al. Citation2011). For these reasons, BMP-2 gene therapy is an alternative and promising method for incorporating the exogenous BMP-2 gene into cells for experimental or therapeutic purposes. Bone regeneration by BMP-2 gene transfer into mesenchymal stem cells has been reported (Qiao et al. Citation2013).
Currently, viral and non-viral vectors are the two typical gene delivery systems that deliver the therapeutic gene into target cells. Viral vectors displayed high transfection efficiency. However, the viral vectors have many safety concerns, such as the lethality, immune responses, and oncogenic effects. Thus, the application of a viral vectors is not advisable (Nie et al. Citation2009). In recent years, non-viral vectors have been increasingly researched, due to their high security, ease of synthesis and chemical modification. Among the non-viral vectors, the chitosan (CS) as a cationic gene carrier has been extensively explored duo to the obvious advantages, such as biodegradability, better biocompatibility, and low cost. According to the principle of electrostatic adsorption, the positively charged chitosan (CS) can condense the negatively charged DNA into nano-sized particles, which can ensure smaller diameter and easier entry into cells and nucleus (Malakooty Poor et al. Citation2014, Nahaei et al. Citation2013). Nanoparticles prepared by chitosan and pBMP-2 have been proven to be effective in transfection of osteogenic precursor cell to promote the osteogenic differentiation both in vitro and in vivo (Nie et al. Citation2009, Nie and Wang Citation2007). However, the relatively low transfection efficiency has restricted its further use as an ideal gene vector. Recent researches suggest that the transfection efficiency of CS vectors can be improved by modifying CS with other functional groups, such as TAT peptide, hyaluronic acid, ornithine, polyethylenimine, etc. (Liu et al. Citation2013, Lu et al. Citation2014, Malhotra et al. Citation2013). Arginine, as the crucial functional components of cell-penetrating peptides (CPPs), has aroused general concern (Alex and Sharma Citation2014, Cardarelli et al. Citation2007, Kaplan et al. Citation2005). Recently, a preliminary study conducted by Yu and coworkers demonstrated that arginine-modified cationic polymer might be a promising gene vector that can facilitate the cellular internalization of DNA (Gao et al. Citation2008). In the present work, we are interested in the application of arginine-modified chitosan (Arg-CS) as the gene vector delivering BMP-2 gene into osteogenic precursor cell to promote osteogenic differentiation.
The repair of bone injury is a relatively long process which needs the long-term stimulation by BMP-2 protein. Thus, a long-term sustained release system is needed to release the BMP-2 gene. PELA microsphere as a controlled-release carrier has been studied in our previous study (Li et al. Citation2015a, Citationb, Min et al. 2015). On the basis of response surface methodology (RSM), we investigated the effects of different factors (molecular weight of PEG, amount of PELA, emulsifier concentration, polyvinyl alcohol concentration, and stirring time) on the entrapment efficiency of a drug-loaded PELA microsphere and achieved the optimal preparation parameters. In this research, in order to achieve the long-term expression of BMP-2 protein, we attempt to package the Arg-CS/pBMP-2 nanoparticle complexes (Arg-CS/pBMP-2 NPs) into PELA microspheres to long-term release and transfect the osteogenic precursor cell, and then efficiently promote the osteogenic differentiation.
Materials and methods
Materials
Chitosan (molecular weight 50 kDa, deacetylation degree 85%) was purchased from Qingdao Hecreat Bio-tech Company Ltd (Qingdao, China). PLA-PEG-PLA (PELA) triblock copolymer (MW = 20,000 Da) was supplied by Shandong Institute of Medical Devices (Shandong, China). pDoubleEx-EGFP-BMP-2 was purchased from Yingrun Biotechnologies Inc. (Changsha, China). Chitosanase, L-arginine, 1-ethyl-3-(3-dimethyl-aminopropyl) carbodiimide (EDC) and N-hydroxysuccinimide (NHS) were purchased from Sigma-Aldrich (St Louis, MO, USA). Fetal bovine serum (FBS) was purchased from Hyclone (Logan, UT, USA). Alpha-minimum essential medium (α-MEM), and antibiotics were from Gibco (Billings, MT, USA). Antibodies against human BMP-2 monoclonal immunoglobulin G was purchased from Abcam (Cambridge, UK). PrimeScript RT Master Kit, QuantiTect SYBR® Green PCR kit, and RNA-extracting reagent RNAiso plus were purchased from Takara Biotechnology Co (Tokyo, Japan). Plasmid Mini Preparation Kit was supplied by Tiangen (Beijing, China). PVA (mean MW = 22,000 Da), span-20, and other reagents were of analytical grade.
Synthesis of arginine-modified chitosan conjugates
Arg-CS were prepared according to the method described by Liu et al. (Citation2004). In brief, 500 mg of chitosan (CS) was dissolved in N, N, N′,N′-tetramethyl-ethyl-enediamine (TEMED)/HCl buffer (pH 5.0) with magnetic stirring. After CS was completely dissolved, 560 mg of 1-ethyl-3,(3-di-methylaminopropyl carbodiimide) and 340 mg of N-hydroxysuccinimide were added to the solution and mixed vigorously. Then, 250 mg of arginine (Arg) was added and the conjugation reaction was carried out for 6–10 h with magnetic stirring at room temperature (RT). The resulting Arg-CS conjugates were collected and dialyzed (MWCO = 8000) against distilled water for 4 d, then lyophilized. The presence of conjugated groups in the Arg-CS was evaluated by FTIR technique and the 1HNMR spectrophotometer.
Construction of nanoparticles loaded with BMP-2 encoding pDNA
The plasmid DNA contained the sequence of BMP-2 and enhanced green fluorescent protein (pEGFP-BMP-2) was propagated in Escherichia coli cells prior to isolation and purification. The absorption ratio was measured at λ = 260 and 280 nm for the evaluation of the plasmid concentration and purity. The Arg-CS/pBMP-2 nanoparticles were prepared by a complex coacervation technique (Mao et al. Citation2001). In brief, Arg-CS was dissolved in 20 mM sodium acetate/acetic acid buffer (pH 5.5) and pBMP-2 was dissolved in 5 mM sodium sulfate. After preheated at 50 °C, the Arg-CS and pBMP-2 solutions were mixed at an N/P ratio (ratio of primary amine to phosphate) of 4:1 and immediately vortexed for 30s. The resulting complexes were placed at RT for 30 min to obtain the Arg-CS/pBMP-2 nanoparticles (Arg-CS/pBMP-2 NPs). As control, CS/pBMP-2 nanoparticles (CS/pBMP-2 NPs) were also prepared by the same process as described above as the control group.
Morphology and characteristics of the nanoparticles
The morphologies of Arg-CS/pBMP-2 NPs were observed by a transmission electron microscope (2100 HC, Japan) using an acceleration voltage of 120 kV. A drop of Arg-CS/pBMP-2 NPs suspension was placed on a mesh carbon-coated Cu grid. The samples were imaged after the solvent evaporated at RT. The zeta potential of the Arg-CS/pBMP-2 NPs were characterized using a Zetasizer Nano ZS (Malvern Instruments, UK).
Gel retarding analysis
To check the protection of condensed pBMP-2 in the nanoparticles from external DNase I degradation, Arg-CS/pBMP-2 NPs (containing 1 μg of pBMP-2) were incubated with different concentration of DNase I (2, 4 units) for 10 min at 37 °C and then loaded in 1% (W/V) agarose gel. After running the gel for 30 min at 80V, the image was recorded under UV light and processed. As positive and negative controls, 1 μg of free pBMP-2 was loaded alone or after incubation with DNase I.
Cell culture
Mouse MC3T3-E1 osteogenic precursor cells were cultured in α-MEM containing 10% FBS and 1% penicillin/streptomycin at 37 °C in a humidified atmosphere of 5% CO2. The medium was changed every 3 d. To induce osteogenesis, the growth medium was replaced with osteogenic α-MEM supplemented with 10% FBS, 100 mM β-glycerophosphate, 50 μg/ml ascorbic acid, and 100 nmol/L dexamethasone.
Evaluation of transfection efficiency and detection intracellular BMP-2 expression
MC3T3-E1 cells were seeded into 6-well plates at 1.5.× 105 cells per well in 500 μl of culture medium and incubated for 24 h prior to transfection. Arg-CS/pBMP-2 NPs were added to the cells (3eμg pBMP-2/well) and incubated for 6 h. After this process, the nanoparticles solution was discarded, and fresh culture medium was added to the wells. After 72 h incubation, the EGFP-positive transfected cells were detected using fluorescence microscope. Then the cells were carefully washed three times with PBS and detached by trypsinization. The EGFP-positive transfected cells, which are positively correlated with the transfection efficiency was quantified by flow cytometry. Cells, which were exposed only to pBMP-2 or CS/pBMP-2 NPs, were analyzed as controls.
Western blot was performed to further confirm the protein expression level of BMP-2, the transfected cells were lysed and the protein concentrations were estimated using a bicinchoninic acid (BCA) protein assay kit. Thirty micrograms of total proteins was separated on a 10% SDS gel. The separated proteins were subsequently transferred to a polyvinylidene difluoride (PVDF) transfer membrane. The membranes were blocked in blocking buffer for 1 h at RT then incubated with primary antibodies against BMP-2 overnight at 4°C and detected using secondary horseradish peroxidase-conjugated antibodies at RT for 1 h. The PVDF transfer membrane was then washed with Tris-buffered saline and developed using an enhanced chemiluminescence detection system with exposure to FluorChem™ HD2.
Preparation of Arg-CS/pBMP-2 NPs-loaded PELA microspheres
Nanoparticles-loaded PELA microspheres were prepared using the water-in-oil-in-water (W/O/W) double emulsion method. Briefly, 300 μl of Arg-CS/pBMP-2 nanoparticles solution (inner aqueous phase, W1) containing 60nμg of pBMP-2 was emulsified in 4 ml of dichloromethane (oil phase, O) containing 280 mg PELA by sonication for 20 min to form the primary emulsion (W1/O). Then the primary emulsion was added dropwise into 40 ml PVA solution (outer aqueous phase, W2) and sonicated to form the second emulsion, which was stirred at 800 rpm for 30 min, Then, the organic solution was evaporated completely and microspheres were solidified. Microspheres were collected by centrifugation at 10,000 rpm for 10 min, washed three times with distilled water and lyophilized.
Morphology and characteristics of the PELA microspheres
The microspheres were imaged using the Hitachi S-3000N scanning electron microscope (SEM). The sample was mounted on aluminum stubs, coated with gold, and then viewed under a SEM at an accelerating voltage of 20 kV. The release of nanoparticles from PELA microspheres was tested in vitro in PBS (pH 7.4) at 37 °C. The microspheres were incubated with 1 ml of PBS in 1.5 ml EP tube at 37 °C. At each time point (2, 4, 8, 12, 16, 22, 28, 35, 42 d), the tubes were centrifuged at 800 rpm for 30 min. The releasate was carefully collected and stored at −20 °C until it was analyzed for pBMP-2 concentration using the Nanodrop spectrophotometer (Thermo Scientific, Waltham, MA) after the addition of chitosanase to degrade chitosan shell. Then, 1 ml of fresh PBS was added into each EP tube for further testing of the release of nanoparticles. The experiments were carried out in triplicate.
BMP-2 protein secretion
The level of BMP-2 protein secreted into the culture medium was determined after 3, 7, 10, 14, 18, 21 d of culture using a BMP-2 ELISA kit according to the manufacturer’s instructions. MC3T3-E1 cells were seeded at 1 × 105 cells/well in 6-well plates and cultured in osteogenic medium with 30tmg Arg-CS/pBMP-2 nanoparticles-loaded PELA microspheres (containing 5oμg of pBMP-2), with medium change every 3 d. The cell culture medium of each time point was collected for enzyme-linked immunosorbent assay (ELISA) to determine the BMP-2 concentration. Cells cultured with CS/pBMP-2 nanoparticles or naked pBMP-2-loaded PELA microspheres as the controls. When the culture medium was collected, the micro spheres were recovered and resuspended in fresh growth medium.
Real-time polymerase chain reaction (RT-PCR)
MC3T3-E1 cells were plated in 6-well plates at a density of 1 × 105 cells/well and cultured in osteogenic medium with PELA microspheres for 3, 7, 14, and 21 d. Cells were harvested at each time point, and total RNA was extracted from the cells using the RNAiso plus reagent. RNA concentration and purity was determined using NanoDrop spectrophotometer. 1 μg total RNA was transcribed into cDNA using PrimeScript RT Master Kit, according to the manufacturer’s protocol. The osteogenic differentiation marker genes were quantified, such as Runx2 (forward: 5′-CCC AGC CAC CTT TAC CTA CA-3′, reverse: 5′-TAT GGA GTG CTG CTG GTC TG-3′), ALP2 (forward: 5′-GCT GAT ATG AGA TGT CCT T-3′, reverse: 5′-GCA CTG CCA CTG CCT ACT-3′), and osteocalcin (OC) (forward: 5′-AAG CAG GAG GGC AAT AAG G-3′, reverse: 5′-GCG GTC TTC AAG CCA TAC TG-3′). The GAPDH was used as a normalization control (forward: 5′-TGT CGT GGA GTC TAC TGG TG-3′, reverse: 5′-GCA TTG CTG ACA ATC TTG AG-3′) (Lin et al. Citation2014b). All the PCR primers were supplied by Sangon Biotech Co., Ltd. (Shanghai, China). Relative mRNA levels were evaluated by RT-qPCR using a QuantiTect SYBR® Green PCR kit. A total of 2 μl cDNA, 0.4 μl primers, 10 μl 2 X SYBR® Premix ExTaq™ and 7.2 μl ddH2O were mixed and used to conduct the reaction with a Roche Light Cycler 480 (Roche Diagnostics GmbH, Mannheim, Germany). Reactions were performed using the following thermal conditions: initial denaturation for one cycle at 95 °C for 30s; 40 cycles of denaturation at 95 °C for 5s, annealing and extension at 60 °C for 34s. Samples were run in triplicate from three independent experiments and analyzed by the 2−ΔΔCt method.
Alkaline phosphatase activity assay and alizarin red staining
MC3T3-E1 cells were seeded on 6-well plates and cultured with PELA microspheres-loaded NPs. After 7 and 14 d following osteogenic induction, the ALP activity was assessed using an ALP detection kit according to the manufacturer’s instruction. Briefly, cells were lysed by 80 μl of 1% Triton X-100 and three freeze-thaw cycles, and then 30 μl of the lysate was mixed with 50 μl of matrix containing disodium phenyl phosphate and 50 μl of alkaline buffer. After placing for 15 min at 37 °C, 150 μl of reagent containing 4-aminoantipyrine and K3Fe(CN)6 was added to stop the reaction. ALP activity was determined as the absorbance at 520 nm. A standard curve was prepared with p-nitrophenol. For analysis of matrix mineralization, MC3T3-E1 cells were cultured with PELA microspheres-loaded NPs in the osteogenic induction medium. After 21 d, the presence of mineralized nodules was assessed by alizarin red staining. Briefly, the cells were fixed with 4% paraformaldehyde for 30 min, rinsed with distilled water three times, followed by incubation with 1% alizarin red S solution for 30 min at 37 °C. After removing the dye, cells were washed with distilled water three times. The cells of each group were observed under a microscope.
Statistical analysis
Statistical analysis was performed with SPSS 20 (SPSS Inc., Chicago, IL). Data are expressed as a mean ± standard deviation (SD). Comparisons among groups were analyzed using one-way analysis of variance (ANOVA), followed by Student–Newman–Keuls test. A value of P < 0.05 was considered to be significant.
Results
Synthesis and characterization of arginine-modified chitosan cationic polymers
The synthesis of Arg-CS is depicted in . The FTIR spectra displayed the distinct peaks of arginine, chitosan, and Arg-CS (). For arginine, the absorption band at 1558.53 cm−1 is attributed to the guanidine group, and the band at 1474.47 cm−1 is assigned to COO- symmetric bending. The bands at 1136.63 cm−1 and 793.87 cm−1 correspond to the C-C-N asymmetric bending and COO- scissoring modes, respectively (Vasudevan et al. Citation2013). In chitosan, the characteristic peaks of NH2 scissoring vibrations at 1655.66 cm−1, carbonyl asymmetric stretching vibrations at 1593.12 cm−1, and C-O stretching vibrations of the pyranose ring at 1077.49 cm−1 and 1026.23 cm−1 (Banerjee et al. Citation2002). Further, comparing with those of chitosan and arginine, several noticeable changes were also observed in the spectra of Arg-CS samples. The band of guanidine group appears at 1543.65 cm− 1 and the band of C-C-N asymmetric bending at 1149.80 cm−1. The new band around 1462.91 cm−1 is most likely due to an amide bond linking between chitosan and arginine (Liu et al. Citation2004). The successful conjugation of arginine to chitosan was further confirmed by 1H NMR spectra. shows the 1H NMR spectrum of arginine, chitosan, and arginine–chitosan. In the arginine–chitosan spectrum the protons contributed by methyl group of chitosan occurred at 2.76 ppm along with proton chemical shift at 3.49 ppm and 4.76 ppm which corresponds to the protons of glucosamine residue. In comparison to chitosan and arginine, the arginine–chitosan spectrum had proton chemical shift occurred at 1.62 ppm since the proton intensity provided by both β- and γ-carbons of arginine fall in the region between 1 ppm and 2 ppm. And integration of protons of δ-carbon of arginine into the chitosan backbone was also found to resonate at 3.26 ppm. On the basis of the FTIR and 1H NMR spectra of arginine–chitosan, it can be concluded that arginine was successfully grafted on the amino groups of chitosan.
Characterization of Arg-CS/pBMP-2 NPs
Morphological characteristics of Arg-CS/pBMP-2 NPs were analyzed by TEM. As shown in , the chitosan-derivative nanoparticles did not form aggregates and showed a uniform distribution of nano-sized particles, most of which had a regular spherical shape and a diameter of 81.39 ± 22.96 nm. Using a Mastersizer laser diffractometer, the zeta potential of the Arg-CS/pBMP-2 NPs was found to be 8.37 ± 4.05 mV ().
Figure 3. (A) The zeta potential of the Arg-CS/pBMP-2 nanoparticles; (B) Nanoparticles morphological analysis by TEM; (C) Gel electrophoresis of Arg-CS/pBMP-2 nanoparticles, digests, and control samples. Lane 1 shows the DNA marker. Lane 2 shows naked plasmid DNA. Lane 3 shows Arg-CS/pBMP-2 nanoparticles. Lanes 4 and 5 show naked plasmid DNA digested by DNase I (2U, 4U respectively). Lanes 6 and 7 show Arg-CS/pBMP-2 nanoparticles digested by DNase I (2U, 4U respectively).

Electrophoresis assay
The integrity of the nanoparticles and the protective capacity against nuclease degradation by cationic Arg-CS polymer were investigated by running agarose gel electrophoresis. In , lane 3 shows that, the pBMP-2 was totally retained within the gel-loading well, which illustrates the complete combination between the Arg-CS and pBMP-2. However the naked plasmid DNA without polymer loaded in the second well can move toward the positive electrode freely.
For effective gene expression, the pBMP-2 in the gene vehicle should be protected from the nuclease. The resistance of Arg-CS/pBMP-2 NPs against DNase I degradation is shown in . The nanoparticles were found to be resistant even at a high concentration of DNase I (lane 6 2U, lane 7 4U), whereas the naked plasmid DNA was almost completely degraded by the same concentration of DNase I (lane 4 2U, lane 5 4U). This result suggests that the pBMP-2 was exceptionally well protected by Arg-CS polymer against nuclease degradation during the process of cell transfection.
In vitro transfection efficiency
To determine whether arginine conjugated to the chitosan backbone could improve the transfection efficiency of the nanoparticles, we compared the transfection efficiency of Arg-CS/pBMP-2 NPs with CS/pBMP-2 NPs and naked pBMP-2 to MC3T3-E1 cells. For this purpose, we used a enhanced green fluorescent protein-encoding plasmid as a reporter gene. As shown in , the fluorescent microscopy images showed the levels of expressed enhanced green fluorescence protein (EGFP) obtained at 72 h after the transfection in the MC3T3-E1 cells. The transfection efficiency of Arg-CS/pBMP-2 NPs was significantly higher than that of CS/pBMP-2 NPs. This enhancement of the transfection efficiency to MC3T3-E1 cells could be a result of the presence of arginine in the chitosan backbone. For quantitative assessment of the transfection efficiency of the nanoparticles, the flow cytometry analysis was used at 72 h post-transfection (). shows that the fluorescence-positive populations of the cells were larger with delivery of pBMP-2 using Arg-CS than using CS (P < 0.05) and naked plasmid (P < 0.01). Furthermore, western blot analysis was performed to examine the expression of BMP-2 protein at 72 h post-transfection. As shown in , the expression level of BMP-2 protein could be detected in Arg-CS/pBMP-2 NPs, CS/pBMP-2 NPs, and naked plasmid groups. And the Arg-CS/pBMP-2 NPs group showed significantly higher BMP-2 protein than CS/pBMP-2 NPs group (P < 0.05) and naked plasmid group (P < 0.01).
Characteristics of PELA microspheres
The microspheres encapsulating Arg-CS/pBMP-2 NPs were successfully prepared by using the modified double emulsion/solvent evaporation technique. The morphology of these microspheres is shown in (SEM). Most of microspheres were smaller than 100 μm in diameter and the diameter was 43.34 ± 15.24 μm. Further, the in vitro release kinetics of the pBMP-2 from PELA microspheres was researched at days 2, 4, 8, 12, 16, 22, 28, 35, 42. As shown in , the release curve exhibits a classic initial burst release about 16.46 ± 4.73% in the first 2 d, followed by a slow release at a relatively constant rate. After 2 weeks, an accelerated release of pBMP-2 was found and last for about 1 week, which could be due to the degradation of PELA wall and release of residual pBMP-2 inside the microspheres. After 3 weeks, the pBMP-2 release reached the plateau and the cumulative release of pBMP-2 reached 56.87 ± 5.09% at 42 d. These results suggest that the pBMP-2 could be released from the PELA microspheres in a sustained pattern. In this research, the entrapment efficiency of PELA microspheres was 67.49 ± 0.85% which was measured by spectrophotofluorometer. All samples were researched in triplicate and data points are shown as means ± standard deviation.
Figure 5. (A) SEM of PELA microcapsules. a and b: Arg-CS/pBMP-2 nanoparticles-loaded microcapsules (100×, 200×); (B) Arg-CS/pBMP-2 nanoparticles release profile from PELA microcapsules in PBS. (C) BMP-2 expressed in cultures was analyzed by ELISA. Data are presented as the mean ± standard deviation (n = 3). *P < 0.05; **P < 0.01.

BMP-2 enzyme-linked immunosorbent assay
To study the long-term transfection efficiency of the nanoparticles loaded in the PELA microspheres, the MC3T3-E1 cells were cultured with PELA microspheres loaded the nanoparticles and the amount of BMP-2 secreted by transfected cells in the culture medium were quantitated using ELISA (). As shown in , MC3T3-E1 cells cultured with the PELA microspheres loaded the Arg-CS/pBMP-2 NPs produced relatively higher level of BMP-2 protein during the culture period. The BMP-2 in the supernatant could be clearly detected after 3 d of culture, reaching the maximum concentration on the 7 d, and then followed by a moderate decline. The peak concentration of BMP-2 was 48.35 ± 3.38 pg/ml. Twenty one days after culture, BMP-2 was still maintained at a high level (29.09 ± 1.87 pg/ml). In comparison, the BMP-2 concentrations of MC3T3-E1 cells cultured with the PELA microspheres-loaded CS/pBMP-2 NPs or naked pBMP-2 maintained at a relatively low level during the culture of MC3T3-E1 cells.
Real time RT-PCR
In this study, the gene expressions of ALP2, RUNX2, and osteocalcin were measured after MC3T3-E1 cells were cultured with the PELA microspheres-loaded Arg-CS/pBMP-2 NPs, CS/pBMP-2 NPs or naked pBMP-2 in osteogenic induction medium at 3, 7, 14, and 21 d. Data from showed that gradual increasing in the ALP2, RUNX2, and osteocalcin mRNA expressions were observed on all samples over time. In particular, the MC3T3-E1 cells cultured with PELA microspheres-loaded Arg-CS/pBMP-2 NPs showed the highest expressions of ALP2, RUNX2, and osteocalcin genes at all the time points, and the CS/pBMP-2 NPs group showed a significantly lower gene expression compared to Arg-CS/pBMP-2 NPs group (P < 0.05). However, the naked pBMP-2 leads to the lowest gene expression level (P < 0.01).
Figure 6. (A) Calcium deposition analysis using alizarin red staining. After 21 d, the MC3T3-E1 cells cultured with PELA microspheres loaded Arg-CS/pBMP-2 NPs showed a significant increase in calcium nodules formation. (B) In vitro ALP activity was measured at 7 d and 14 d. The group transferred with Arg-CS/pBMP-2 NPs showed the highest ALP activity level than the other transferred groups at days 7 and 14 (P<0.01). (C) Expression levels of ALP2, OC and Runx2 mRNA were quantified by real-time RT-PCR after 3, 7, 14, and 21 days post-transfection. Data are presented as the mean±standard deviation (n=3). *P<0.05; **P<0.01.
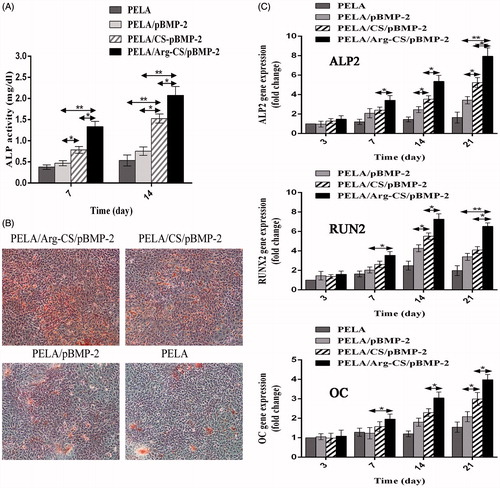
In vitro cell osteogenic differentiation results
The osteogenic differentiation markers of MC3T3-E1 cells including the ALP activity and calcified nodule formation. Alkaline phosphatase (ALP) as a standard early marker of induction of osteogenic differentiation was measured in vitro at the culture period of 7 and 14 d. Quantitative analysis of the ALP activity revealed that the activity in all groups increased time dependently (). All transferred groups showed higher ALP activity than the control group. However, the group transferred with Arg-CS/pBMP-2 NPs showed the highest ALP activity level than the other transferred groups at days 7 and 14 (P < 0.01). In addition, alizarin red stains was performed after 21 d of exposure to the osteogenic induction medium, the MC3T3-E1 cells cultured with PELA microspheres-loaded Arg-CS/pBMP-2 NPs showed a significant increase in calcium nodules formation indicating that the microsphere can be used as a good implant to accelerate the osteogenic differentiation of MC3T3-E1 cells.
Discussion
The growth factors, especially BMP-2, can promote the differentiation of osteogenic precursor cell. However, BMPs protein therapy has certain demerits, such as high cost, large dose requirements and short half-life. Gene therapy may be an alternative technique to deliver this short-lived cytokine, as it offers the advantages of inducing local BMPs expression and maintain the natural conformation of overexpressed endogenous BMPs (Kang et al. Citation2000).
Viral or non-viral vectors are the two typical gene delivery systems, for bone regeneration, non-viral systems are advantageous due to the safety, lower cost, lower immunogenicity, and convenient application. The cationic polymer chitosan is subjected to widespread research for chemical modifications to achieve an ideal gene vector. Arginine moieties have been found in CPPs, which was proved to be the crucial component mediating the process of cell penetration. Recently, arginine or arginine rich peptides have been demonstrated to enhance the expression of transgenes either by themselves alone or in the form of arginine-conjugated polymers (Hussein-Al-Ali et al. Citation2014). Therefore, in this study, we graft arginine to the chitosan backbone forming a kind of Arg-CS polymer to promote cell transfection efficiency. As shown in , the chemical composition of Arg-CS derivative was ascertained by FTIR and 1H NMR. In Arg-CS derivative, arginine on reaction with chitosan resulted in guanidine group peak at 1543.65 cm−1 along with a new band around 1462.91 cm−1 that clearly correlated to substitution of arginine. Subsequent NMR further ascertained the successful grafting of arginine into chitosan.
Nanoparticle formation is the crucial process for generating a polymeric gene carrier in which the average diameter of the particles is important factor to be considered. The Arg-CS/pBMP-2 NPs encoding BMP-2 were fabricated through complex coacervation, with resulting nanoparticles diameters 81.39 ± 22.96 nm. The nanoparticle sizes were suitable for cells transfection via endocytosis of the whole nanoparticle. The zeta potential also indicated a better dispersity. Furthermore, the gel electrophoresis showed the pBMP-2 condensed into the Arg-CS/pBMP-2 NPs can resist even a high concentration of DNase I, which was favorable for the survival and effective expression of BMP-2 gene after endocytosis. Transfection efficiency is considered as one of the main thresholds for non-viral vector for bone tissue engineering. Although chitosan has cationic and biocompatible properties, the gene transfection efficiency of chitosan has been known to be lower as compared to other cationic molecules such as PEI. To enhance the transfection efficiency of chitosan, the additional arginine moieties were linked to chitosan backbone. The previous research by Zhu et al. showed the conjugating arginine can introduce guanidino moieties. Meanwhile, the oligomeric arginine and the introduced guanidino moieties had been found to efficiently translocate the Arg-CS/pBMP-2 NPs through cell membranes. In this study, shows the transfection efficiency in MC3T3-E1 cells. In comparison with CS/pBMP-2 NPs group and naked pBMP-2 group, Arg-CS/pBMP-2 NPs showed much higher transfection efficiency indicating that the introduction of arginine to chitosan backbone is beneficial for gene delivery.
It is well known that the repair of bone injury needs a relatively long process. Therefore, a carrier is necessary to maintain a long-term sustained release of Arg-CS/pBMP-2 NPs. PELA microsphere as a sustained release carrier has been researched in our previous study. In the present study, in order to achieve the long-term expression of BMP-2 protein, we attempt to encapsulate the Arg-CS/pBMP-2 NPs into PELA microspheres to long-term release and transfect the osteogenic precursor cell. As shown in , most of the PELA microspheres were smaller than 100 μm in diameter and the diameter was 43.34 ± 15.24 μm which were similar to our previous study in the aspect of morphology. On the basis of optimized process parameters, we measured the entrapment efficiency of the PELA microspheres-loaded Arg-CS/pBMP-2 NPs reached 67.49 ± 0.85%. Further, the in vitro release kinetics of Arg-CS/pBMP-2 NPs from PELA microspheres was measured. The release characteristics of Arg-CS/pBMP-2 NPs suggested that the PELA microsphere was a well-controlled system capable of releasing Arg-CS/pBMP-2 NPs for a longer period of more than 42 d. Though a small initial burst release was found in the first 48 h, the majority of pBMP-2 was cumulatively released from PELA microspheres under a more sustained period. Even after 42 d, the release of Arg-CS/pBMP-2 NPs still can maintain at an adequate level for long-term osteogenic induction.
In this study, for cells cultured with PELA microspheres, ELISA was used to determine how much BMP-2 was secreted by MC3T3-E1. The secretion level of BMP-2 in cells cultured with PELA microspheres-loaded Arg-CS/pBMP-2 NPs was significantly higher than that of the two control groups during 21 d of culture. According to the ELISA results, The BMP-2 secretion could be detected after 3 d of culture, reaching its highest level on the 7 d and still maintaining a high level after 21 d of culture. This may be due to the fact that MC3T3-E1 have a relatively static growth period at the first few days after inoculation, in this period it is hard to endocytosed the nanoparticles for the relatively static cells. Moreover, upon entry into the cell the Arg-CS sheel must be degraded by a polysaccharide enzyme before the pBMP-2 can be gradually released and enter into the nucleus. Until the exponential phase, the cells achieve integration with the architecture of their microenvironments and increase the expression of BMP-2 protein. These possible reasons may explain why the expression of BMP-2 protein remained at a low level during the first few days of culture. However, in comparison with the PELA microspheres-loaded CS/pBMP-2 NPs group and blank control group, the BMP-2 protein expression remained significantly higher in PELA microspheres-loaded Arg-CS/pBMP-2 NPs group.
The osteogenic differentiation markers of MC3T3-E1 cells including the osteogenesis-related gene expression, ALP activity and calcified nodule formation (Lin et al. Citation2014a). In this research, we examined the mRNA expressions of Runx-2, ALP, and OC. Runx-2 as a transcription factor is essential for osteoblastic differentiation. The ALP and OC are closely related to osteoblasts maturation and matrix mineralization (Hakki et al. Citation2010, Liu et al. Citation2014). As shown in , an obviously upregulation in the Runx-2, ALP and OC mRNA expression were observed on PELA microspheres-loaded Arg-CS/pBMP-2 NPs group, as compared to other groups. The highest expression of ALP and OC mRNA gene were on days 21, mainly attributed to the effect of over-expressed BMP-2. However, among all samples, a gradual decrease in the Runx2 mRNA expression was found after 14 d of culture. Interestingly, in the previous research, Eun-Cheol Kim considered that the decrease in the Runx2 mRNA expression after 14 d is a mark of accelerating osteogenic differentiation (Kim et al. Citation2014). Further, ALP activity and calcium deposition were detected. ALP activity and calcium deposition are early and late markers of osteoblastic cells, respectively. Enhanced ALP activity level on days 7, 14, and calcium deposition amount on days 21 were observed on the PELA microspheres-loaded Arg-CS/pBMP-2 NPs group, as compared to other groups. Consequently, the PELA microspheres-loaded Arg-CS/pBMP-2 NPs may induce the enhanced osteogenic differentiation of MC3T3-E1 cells.
Although we proved that Arg-CS/pBMP-2 NPs can effectively transfect MC3T3-E1 cells and promote osteogenic differentiation by PELA microspheres-loaded Arg-CS/pBMP-2 NPs during the culture period, the main limitation of this study is that we did not verify its effectiveness in vivo. Further studies are needed to fully confirm its application in vivo.
Conclusions
In this study, we designed and evaluated a novel gene sustained release system composed of PELA microspheres with encapsulated Arg-CS/pBMP-2 NPs in it for bone tissue engineering applications. The data revealed that the Arg-CS/pBMP-2 NPs have a better performance in cell transfection, and the release of nanoparticles from PELA microspheres was steady and last for more than 42 d. MC3T3-E1 cells cultured with the PELA microspheres-loaded Arg-CS/pBMP-2 NPs nanoparticles showed increased BMP-2 protein expression resulting in enhanced differentiation of osteoblastic progenitor cells. In conclusion, the novel gene controlled-release system consisting of PELA microspheres with encapsulated Arg-CS/pBMP-2 NPs in it may be a promising system for bone tissue engineering applications.
Funding information
This work was supported by the Natural Science Foundation of Guangdong Province (No. 2014A030313348), Medical Research Foundation of Guangdong Province (A2014424), Scientific Research Foundation of Southern Medical University (No. PY2014NO64), People’s Republic of China.
Acknowledgements
We would like to thank Chen Jianting (orthopedics department professor in Nan Fang hospital, People’s Republic of China) for providing the MC3t3-E1 cells used in this study.
Disclosure statement
The authors report no declarations of interest. The authors alone are responsible for the content and writing of the paper.
References
- Alex SM, Sharma CP. 2014. Enhanced intracellular uptake and endocytic pathway selection mediated by hemocompatible ornithine grafted chitosan polycation for gene delivery. Colloids Surf B Biointerfaces. 122:792–800.
- Banerjee T, Mitra S, Kumar Singh A, Kumar Sharma R, Maitra A. 2002. Preparation, characterization and biodistribution of ultrafine chitosan nanoparticles. Int J Pharm. 243:93–105.
- Cardarelli F, Serresi M, Bizzarri R, Giacca M, Beltram F. 2007. In vivo study of HIV-1 Tat arginine-rich motif unveils its transport properties. Mol Ther. 15:1313–1322.
- Faensen B, Wildemann B, Hain C, Höhne J, Funke Y. 2011. Local application of BMP-2 specific plasmids in fibrin glue does not promote implant fixation. BMC Musculoskelet Disord. 12:163.
- Gao Y, Xu Z, Chen S, Gu W, Chen L, Li Y. 2008. Arginine-chitosan/DNA self-assemble nanoparticles for gene delivery: In vitro characteristics and transfection efficiency. Int J Pharm. 359:241–246.
- Hakki SS, Bozkurt BS, Hakki EE. 2010. Boron regulates mineralized tissue-associated proteins in osteoblasts (MC3T3-E1). J Trace Elem Med Biol. 24:243–250.
- Hussein-Al-Ali SH, Arulselvan P, Fakurazi S, Hussein MZ, Dorniani D. 2014. Arginine-chitosan- and arginine-polyethylene glycol-conjugated superparamagnetic nanoparticles: preparation, cytotoxicity and controlled-release. J Biomater Appl. 29:186–198.
- Ji Y, Xu GP, Zhang ZP, Xia JJ. 2010. BMP-2/PLGA delayed-release microspheres composite graft, selection of bone particulate diameters, and prevention of aseptic inflammation for bone tissue engineering. Ann Biomed Eng. 38:632–639.
- Jin H, Zhang K, Qiao C, Yuan A, Li D. 2014. Efficiently engineered cell sheet using a complex of polyethylenimine-alginate nanocomposites plus bone morphogenetic protein 2 gene to promote new bone formation. Int J Nanomedicine. 9:2179–2190.
- Kang R, Ghivizzani SC, Muzzonigro TS, Herndon JH. 2000. The Marshall R. Urist Young Investigator Award. Orthopaedic applications of gene therapy. From concept to clinic. Clin Orthop Relat Res. 375:324–337.
- Kaplan IM, Wadia JS, Dowdy SF. 2005. Cationic TAT peptide transduction domain enters cells by macropinocytosis. J Control Release. 102:247–253.
- Kim EC, Kim TH, Jung JH, Hong SO, Lee DW. 2014. Enhanced osteogenic differentiation of MC3T3-E1 on rhBMP-2-immobilized titanium via click reaction. Carbohydr Polym. 103:170–178.
- Li X, Jin A, Duan Y, Min S. 2015a. Optimization of entrapping conditions to improve the release of BMP-2 from PELA carriers by response surface methodology. Biomed Mater. 10: 015002.
- Li X, Yi W, Jin A, Duan Y, Min S. 2015b. Effects of sequentially released BMP-2 and BMP-7 from PELA microcapsule-based scaffolds on the bone regeneration. Am J Transl Res. 7:1417–1428.
- Lin Z, Feng R, Li J, Meng Y, Yuan L. 2014a. Nuclear translocation of CBP/p300-interacting protein CITED1 induced by parathyroid hormone requires serine phosphorylation at position 79 in its 63-84 domain. Cell Signal. 26:2436–2445.
- Lin Z, Wang JS, Lin L, Zhang J. 2014b. Effects of BMP2 and VEGF165 on the osteogenic differentiation of rat bone marrow-derived mesenchymal stem cells. Exp Ther Med. 7:625–629.
- Liu Q, Wang W, Zhang L, Zhao L. 2014. Involvement of N-cadherin/β-catenin interaction in the micro/nanotopography induced indirect mechanotransduction. Biomaterials. 35:6206–6218.
- Liu WG, Zhang JR, Cao ZQ, Xu FY, Yao KD. 2004. A chitosan-arginine conjugate as a novel anticoagulation biomaterial. J Mater Sci Mater Med. 15:1199–1203.
- Liu Y, Kong M, Cheng XJ, Wang QQ. 2013. Self-assembled nanoparticles based on amphiphilic chitosan derivative and hyaluronic acid for gene delivery. Carbohydr Polym. 94:309–316.
- Lu H, Dai Y, Lv L, Zhao H. 2014. Chitosan-graft-polyethylenimine/DNA nanoparticles as novel non-viral gene delivery vectors targeting osteoarthritis. PLoS One. 9:e84703.
- Malakooty Poor E, Baghaban Eslaminejad M, Gheibi N, Bagheri F. 2014. Chitosan-pDNA nanoparticle characteristics determine the transfection efficacy of gene delivery to human mesenchymal stem cells. Artif Cells Nanomed Biotechnol. 42:376–384.
- Malhotra M, Tomaro-Duchesneau C, Saha S, Kahouli I, Prakash S. 2013. Development and characterization of chitosan-PEG-TAT nanoparticles for the intracellular delivery of siRNA. Int J Nanomedicine. 8:2041–2052.
- Mao HQ, Roy K, Troung-Le VL, Janes KA, Lin KY. 2001. Chitosan-DNA nanoparticles as gene carriers: synthesis, characterization and transfection efficiency. J Control Release. 70:399–421.
- Nahaei M, Valizadeh H, Baradaran B, Nahaei MR. 2013. Preparation and characterization of chitosan/β-cyclodextrin nanoparticles containing plasmid DNA encoding interleukin-12. Drug Res. 63:7–12.
- Nie H, Ho ML, Wang CK, Wang CH, Fu YC. 2009. BMP-2 plasmid loaded PLGA/HAp composite scaffolds for treatment of bone defects in nude mice. Biomaterials. 30:892–901.
- Nie H, Wang CH. 2007. Fabrication and characterization of PLGA/HAp composite scaffolds for delivery of BMP-2 plasmid DNA. J Control Release. 120:111–121.
- Osawa K, Okubo Y, Nakao K, Koyama N, Bessho K. 2010. Osteoinduction by repeat plasmid injection of human bone morphogenetic protein-2. J Gene Med. 12:937–944.
- Qiao C, Zhang K, Jin H, Miao L, Shi C, Liu X. 2013. Using poly(lactic-co-glycolic acid) microspheres to encapsulate plasmid of bone morphogenetic protein 2/polyethylenimine nanoparticles to promote bone formation in vitro and in vivo. Int J Nanomedicine. 8:2985–2995.
- Vasudevan P, Gokul RS, Sankar S. 2013. Synthesis and characterization of nonlinear optical L-arginine semi-oxalate single crystal. Spectrochim Acta A Mol Biomol Spectrosc. 106:210–215.
- Wegman F, Bijenhof A, Schuijff L, Oner FC, Dhert WJ. 2011. Osteogenic differentiation as a result of BMP-2 plasmid DNA based gene therapy in vitro and in vivo. Eur Cell Mater. 21:230–242.