Abstract
Herein, Fe3O4 nanoparticles synthesized using aqueous extract of corn ear leaves were investigated for proteasome inhibitory activity, antioxidant activity, synergistic antibacterial, and anticandidal potential. The UV-Vis spectrum displayed an absorption band at 355 nm that indicated the formation of nano-sized Fe3O4 particles. Vibrating sample magnetometer analysis revealed its superparamagnetic nature. Fe3O4 nanoparticles exhibited strong proteasome inhibitory potential and antioxidant activity and exerted strong synergistic antibacterial and anticandidal activity. Its significant proteasome inhibitory potential could be useful in cancer treatment and drug delivery. Furthermore, strong antioxidant, antibacterial, and anticandidal activity make them a promising candidate for biomedical and pharmaceutical applications.
Introduction
The developments in synthesizing different types of biocompatible and functionalized magnetic nanoparticles have attracted a great deal of attention in the modern scientific world. Magnetic nanoparticles are usually composed of magnetic cores and polymeric shells having favorable functional groups and features for various applications including drug delivery, hyperthermia treatment, cell separation, magnetic resonance imaging, contrast agents magneto-motive ultrasound, optical imaging, and biosensors (Chen et al. Citation2012, Oh et al. Citation2007, Xu et al. Citation2011, Zhang et al. Citation2005). Furthermore, various nano-products have made their way into cosmetics and personal care products, including antibacterial agents, anti-wrinkle creams, deodorants, toothpaste, sunscreen lotions, and shampoos (Oberdorster et al. Citation2005).
Generation of Fe3O4 magnetic nanoparticles has been rising significantly as a useful material in drug delivery and biomedical applications because of their non-toxicity, small size, and superparamagnetic properties (Dung et al. Citation2009). When prepared with smaller dimensions or sizes comparable to biological entities, Fe3O4 nanoparticles can be coated with different types of biological molecules and dispersed in a liquid medium to form colloidal solutions known as magnetic fluids or ferro fluids. Because of their liquid properties and sensitivity to applied magnetic fields, these colloidal materials can be used in delivery of different types of targeted drugs such as anticancer drugs or radio nuclide atoms to a targeted region of the body such as tumor, etc. (Arum et al. Citation2015, Jayanthi et al. Citation2015).
Proteasomes are large multi catalytic proteinase complexes located in the cytoplasm and nucleus in all eukaryotic cells (Chen and Ping Dou Citation2010). These complexes are responsible for the degradation of many cytosolic proteins (Tanaka et al. Citation2012). Human cancer cells possess an elevated level of proteasome activity therefore, inhibition of the proteasome activity could be a novel approach to the treatment of cancer (Chen and Ping Dou Citation2010).
There are several methods for the synthesis of Fe3O4 magnetic nanoparticles, and these utilize both chemical and biological processes. However, biological methods are the most widely accepted as they utilize green technology with reduced environmental hazards through the use of non-toxic chemicals and renewable materials (Senthil and Ramesh Citation2012). There are many reports on synthesis of nanoparticles using different parts of the Zea mays plant (Deb Citation2014, Leela and Vivekanandan Citation2008). However, no such report is available on the synthesis of Fe3O4 nanoparticles using the Z. mays plant. Thus, herein, the biosynthesis of Fe3O4 nanoparticles using the aqueous extract of the ear leaves of corn and its biomedical applications such as proteasome inhibitory activity, antioxidant, synergistic antibacterial, and anticandidal potential have been attempted.
Experimental
Preparation of aqueous extract of ear leaves of corn
The corn of Z. mays L. was purchased from a local market located at Gyeonsan, Republic of Korea on 14 August 2014, and the ear leaves were collected (, inset) and cut into small pieces (approximately 1 cm). A total of 20 g of the leaf pieces was placed in a 250 mL conical flask, mixed with 100 mL of double distilled water, and boiled for 15 min with continuous stirring. The prepared ear leaf extract (ELE) was then cooled to room temperature, filtered with Whatman No. 1 filter paper and stored at 4 °C before being used for the synthesis of Fe3O4 magnetic nanoparticles (Patra and Baek Citation2015).
Figure 1. (A) UV-Vis spectrum of the Fe3O4 nanoparticles (inset: The ear leaves covering corn used for the synthesis of Fe3O4 nanoparticles and, FeCl2 and FeCl3 as the precursor compounds in aqueous extract of corn ear leaves (yellow) and synthesized Fe3O4 nanoparticles (black)); (B) Scanning electron microscopy (FE-SEM, inset: Selected Fe3O4 nanoparticle for EDX analysis); and (C) energy-dispersive X-ray spectroscopy (EDX, inset: elemental composition of Fe3O4 nanoparticle) of the Fe3O4 nanoparticles.
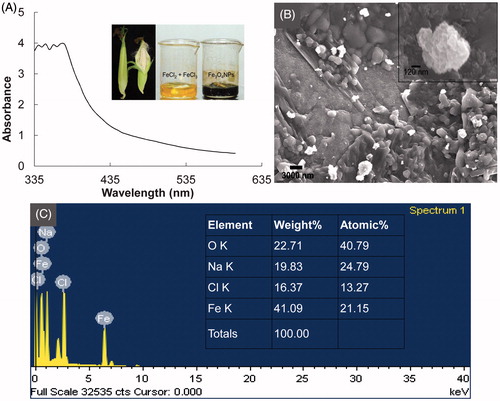
Synthesis and characterization of Fe3O4 magnetic nanoparticles
Fe3O4 magnetic nanoparticles were synthesized according to the procedure described by Awwad and Salem (Citation2012) using FeCl2 and FeCl3 as the precursor compounds and ELE as the reducing agent. The nanoparticles were stored in an airtight bottle at room temperature until use. The synthesized Fe3O4 nanoparticles were characterized by various analytical measurements including UV-Vis spectroscopy, field emission scanning electron microscopy (FE-SEM), energy-dispersive X-ray spectroscopy (EDX), Fourier-transform infrared spectroscopy (FT-IR), differential thermogravimetric (TG/DTG) analysis, vibrating sample magnetometer (VSM), and X-ray diffraction (XRD) analysis using standard procedures (Dizaji et al. Citation2015, Singh et al. Citation2015).
The optical properties of the Fe3O4 nanoparticles were studied by measuring the absorption spectra of the Fe3O4 magnetic nanoparticle solution at a resolution of 1 nm between 350 nm and 600 nm using a micro plate reader (Infinite 200 PRO NanoQuant, Tecan, Mannedorf, Switzerland). The morphology of the Fe3O4 nanoparticles was analyzed using a FE-SEM (S-4200, Hitachi, Japan). Elemental analysis of the Fe3O4 nanoparticles was conducted using an EDX detector (EDS, EDAX Inc., Mahwah, NJ) attached to the FE-SEM machine. FT-IR analysis of the synthesized Fe3O4 nanoparticles and ELE were conducted using an FT-IR spectrophotometer (Jasco 5300, Jasco, Mary’s Court, MD) in the wavelength range of 400–4000 cm−1. The effects of high temperature on the synthesized Fe3O4 nanoparticles were evaluated by a TG machine from 20 °C to 700 °C (SDT Q600, TA Instruments, New Castle, DE). The magnetic properties of Fe3O4 nanoparticles were measured using a VSM machine (Model 7404, Lake Shore Cryotronics, Westerville, OH). All measurements were carried out at room temperature and a magnetic field of −15,000 G to +15,000 G (Basavegowda et al. Citation2014). The Fe3O4 nanoparticles were analyzed using a XRD (X’Pert MRD model, PANalytical, Almelo, The Netherlands). The machine was setup at 30 kV and 40 mA with Cu Kα radians at an angle of 2θ to determine crystallite size and the nature of the magnetic nanoparticles.
Bioactivity potential of Fe3O4 nanoparticles
The proteasome inhibitory potential of Fe3O4 nanoparticles was assayed using a 20 S proteasome assay kit for drug discovery (ENZO Life Sciences, Farmingdale, NY) according to the manufacturer protocols. Fe3O4 nanoparticles at 10 and 100 μg/mL and epoxomicin at 0.56 and 5.56 μg/mL as the standard reference compound were used for the assay. The Chymotrypsin-like proteasomal activity of the Fe3O4 nanoparticles in a 96 well microplate were measured based on the fluorescence intensities at an excitation of 350 nm and emission of 440 nm at 30 °C reaction temperature using a microplate reader.
The antioxidant potential of the Fe3O4 nanoparticles was determined in vitro by ABTS [2,2′-azino-bis(3-ethyl benzo thiazoline-6-sulphonic acid)] free radical scavenging, nitric oxide scavenging, DPPH (1,1-diphenyl-2-picrylhydrazyl) free radical scavenging and reducing power assays. Butylated hydroxyl toluene (BHT) (Supelco, Bellefonte, PA) was used as the standard reference compound. All experiments were conducted using nanoparticles at 20, 40, 60, 80, and 100 μg/mL. The ABTS radical scavenging potential of Fe3O4 nanoparticles was determined as described by Thaipong et al. (Citation2006). The absorbance of the reaction mixtures was recorded at 750 nm using a microplate reader and the results were interpreted according to the following equation:
(1)
where, Acon was the absorbance of the control and Atre was the absorbance of the treatment.
The nitric oxide radical scavenging potential of Fe3O4 nanoparticles was determined by the standard method (Makhija et al. Citation2011). The absorbance of the reaction mixtures was recorded at 546 nm and the results were interpreted as per EquationEq. (1)(1) . The DPPH free radical scavenging potential of Fe3O4 nanoparticles was determined as described by Patra et al. (Citation2015). The absorbance of the reaction mixtures was recorded at 517 nm and the results were interpreted as per EquationEq. (1)
(1) . The reducing power of the Fe3O4 nanoparticles was determined as described by Sun et al. (Citation2011). Briefly, the absorbance was measured at 700 nm against an appropriate control and the results were expressed as OD values at 700 nm.
The synergistic antibacterial potential of Fe3O4 nanoparticles and the standard antibiotics (kanamycin and rifampicin) was determined by the standard disc diffusion method (Naqvi et al. Citation2013). Five different foodborne bacteria were used in the study, Bacillus cereus ATCC 13061, Escherichia coli ATCC 43890, Listeria monocytogenes ATCC 19115, Staphylococcus aureus ATCC 49444, and Salmonella typhimurium ATCC 43174. All pathogens were obtained from the American Type Culture Collection (ATCC, Manassas, VA) and maintained as glycerol stock at −80 °C. Prior to use, the bacterial pathogens were freshly cultured on nutrient broth media (Difco, Becton, Dickinson and Company, Sparks Glencoe, MD). Fe3O4 nanoparticles (1 mg/mL) and two standard antibiotics (kanamycin and rifampicin at 200 μg/mL) were mixed at a 1:1 ratio and sonicated for 15 min at room temperature. Antibiotic discs were prepared by adding 50 μL of the Fe3O4 nanoparticles/antibiotics mixture solution to a 6 mm filter paper disc containing 25 μg Fe3O4 nanoparticles and 5 μg antibiotics. The diameters of the zones of inhibition were measured after 24 h of incubation at 37 °C.
The synergistic anticandidal potential of Fe3O4 nanoparticles and amphotericin b, a standard antifungal agent, was determined by the disc diffusion method (Murray et al. Citation1995). Five different pathogenic Candida species were used in the study, C. albicans KACC 30003 and KACC 30062, C. glabrata KBNO 6P00368, C. geochares KACC 30061, and C. saitoana KACC 41238. These species were obtained from the Korean Agricultural Culture Collection (KACC, Suwon, Republic of Korea). Fe3O4 nanoparticles (2 mg/mL) and amphotericin b (200 μg/mL) were mixed in a 1:1 ratio and sonicated for 15 min at room temperature. Antifungal discs were prepared by adding 50 μL of the Fe3O4 nanoparticles/amphotericin b mixture solution to a 6 mm filter paper disc containing 50 μg Fe3O4 nanoparticles and 5 μg amphotericin b. The Candida species were grown on potato-dextrose agar (PDA) media at 28 °C, loaded uniformly on PDA plates and covered with antifungal discs. After incubating the plates at 28 °C for 48 h, the data were obtained by measuring the diameters of the zones of inhibition.
Statistical analysis
All results were expressed as the mean value of three independent replicates ± the standard deviation (SD). Significance differences between the mean values were identified by one-way analysis of variance (ANOVA) followed by Duncan’s test at the 5% level of significance (P < 0.05) using the Statistical Analysis Software (SAS) (Version: SAS 9.4, SAS Institute Inc., Cary, NC).
Results and discussion
Synthesis and characterization of Fe3O4 magnetic nanoparticles
Fe3O4 nanoparticles were synthesized by a green technology procedure that utilized the non-toxic environmental benign aqueous extract of the ear leaves of corn (, inset). The synthesis method described herein is a novel approach toward utilization of bio-waste materials because there are large amounts of these leaves generated during the processing of corn kernels in food and feedstuff factories. The synthesis of ferromagnetic Fe3O4 was confirmed by the change in the color of the mixture solution from yellow to black (, inset). The utilization of eco-friendly methods for the synthesis of different types of nanoparticles is of the utmost importance to improve their biological applications (Shankar et al. Citation2004).
The UV-Vis spectrum of Fe3O4 nanoparticles showed an absorption band in the range of 335–375 nm () that was primarily due to the absorption and scattering of radiation by magnetic nanoparticles, in accordance with previously reported literature (Rahman et al. Citation2012). The absorption band at 355 nm indicated the formation of nano-sized Fe3O4 particles.
Further analysis of the existence of the nanoparticles and the morphology of the synthesized Fe3O4 nanoparticles was revealed by FE-SEM analysis (). The SEM image confirmed that the nanoparticles were spherical in shape, and strongly aggregated and clustered. They also contained a large number of sub-particles of smaller size. The elemental composition of the synthesized nanoparticles was determined by an EDX machine attached to the SEM. The elemental composition confirmed that Fe3O4 nanoparticles were composed of 63.8% Fe and O, 19.8% Na, and 16.4% Cl (). Two typical absorption peaks at 0.6 and 6.5 keV were observed owing to surface plasmon resonance, which confirmed that the synthesized nanoparticles were composed of Fe and O (). The presence of Na might have been due to the addition of NaOH to the reaction mixture. Similar results were reported previously (Basavegowda et al. Citation2014, Shankar et al. Citation2004).
FT-IR spectroscopy analysis of both the ELE and synthesized Fe3O4 nanoparticles was conducted (). The spectra of the ELE showed strong bands at 3413, 2363, 2181, 1645, 1078, and 664 cm−1 (), whereas those of the Fe3O4 nanoparticles showed strong bands at 3464, 2366, 1626, 1109, 1018, and 602 cm−1 (). The band at 3413 cm−1 in the ELE was assigned to O–H stretching vibration associated with alcohol or phenol groups, which was shifted to 3464 cm−1 in the Fe3O4 nanoparticles owing to the biomaterial binding. The absorption peaks at 1645 and 1626 cm−1 were assigned to C = C stretching vibrations of the biomolecules (Awwad and Salem Citation2012). The absorption peaks at 1078 cm−1 were assigned to C–N stretching vibrations belonging to the alipathic amine group, which were shifted to 1018 cm−1 in the Fe3O4 nanoparticles (). The band at 602 cm−1 in the synthesized Fe3O4 nanoparticles was due to the metal-oxygen stretching vibration modes and corresponded to Fe3O4 (Koutzarova et al. Citation2006).
Figure 2. (A) Fourier-transform infrared spectroscopy [FT-IR; Fe3O4 nanoparticles (FeNPs) and aqueous extract of corn ear leaves (ELE)]; (B) Differential thermogravimetric (TG/DTG) spectra of Fe3O4 nanoparticles; (C) Vibrating sample magnetometer (VSM) graph of Fe3O4 nanoparticles (inset: indicates the magnetic properties of the synthesized Fe3O4 nanoparticles); and (D) X-ray diffraction (XRD) analysis of Fe3O4 nanoparticles.
![Figure 2. (A) Fourier-transform infrared spectroscopy [FT-IR; Fe3O4 nanoparticles (FeNPs) and aqueous extract of corn ear leaves (ELE)]; (B) Differential thermogravimetric (TG/DTG) spectra of Fe3O4 nanoparticles; (C) Vibrating sample magnetometer (VSM) graph of Fe3O4 nanoparticles (inset: indicates the magnetic properties of the synthesized Fe3O4 nanoparticles); and (D) X-ray diffraction (XRD) analysis of Fe3O4 nanoparticles.](/cms/asset/f13c7765-1b07-422e-9a8e-39184941b130/ianb_a_1153484_f0002_c.jpg)
The TG/DTG analysis of the synthesized Fe3O4 nanoparticles presented in revealed the weight loss steps from room temperature to 700 °C. The maximum weight loss occurred at 400 °C (), indicating that bioactive compounds from ELE attached to the surface of Fe3O4 nanoparticles were degraded at temperatures higher than 400 °C as previously reported (Basavegowda et al. Citation2014, Thomasa et al. Citation2015). The magnetic properties of the synthesized Fe3O4 nanoparticles were examined by VSM analysis (). The hysteresis loops indicated that Fe3O4 nanoparticles were superparamagnetic in nature (Rahman et al. Citation2012). The coercivity (Hc), saturation magnetization (Ms), and remanent magnetization (Mr) value were 0 G, 1.411 and 0.129 emu g−1, respectively. The VSM results obtained in the present study were similar to those previously reported for magnetic nanoparticles (Aftabtalab and Sadabadi Citation2015, Atta et al. Citation2015, Basavegowda et al. Citation2014, Rahman et al. Citation2012, Shankar et al. Citation2004). The XRD pattern of the synthesized Fe3O4 nanoparticles is shown in . The diffraction peaks obtained at 31.76°, 35.54°, 43.32°, 45.50°, 56.53°, 62.80°, and 75.33° 2θ correspond to (220), (311), (400), (422), (511), (440), and (533) planes of Fe3O4, respectively, and are comparable to the standard XRD pattern of Fe3O4 nanoparticles in the database of the Joint Committee on Powder Diffraction Standards (JCPDS card No.: 19-0629) (Basavegowda et al. Citation2014). The estimated absolute crystallite sizes of the Fe3O4 nanoparticles was calculated to be 37.86 nm based on the Scherer equation (Aftabtalab and Sadabadi Citation2015). All peaks of the Fe3O4 nanoparticles were identified; however, some unassigned peaks were observed, which might have been due to the crystallization of some bioactive compounds from the ELE on the surface of the Fe3O4 nanoparticles (Philip et al. Citation2011).
Bioactivity of the of Fe3O4 magnetic nanoparticles
The proteasome inhibitory potential of Fe3O4 magnetic nanoparticles is presented in . The results showed that Fe3O4 magnetic nanoparticles at a concentration of 100 and 10 μg/mL exhibited strong proteasome inhibitory potential of 78.11% and 51.73%, respectively, whereas the reference standard epoxomycin at a concentration of 5.56 and 0.56 μg/mL exhibited moderate proteasome inhibitory activity of 47.72% and 33.04%, respectively. The results displayed a dose dependent anti-proteasome potential of Fe3O4 magnetic nanoparticles. The Fe3O4 magnetic nanoparticles could also serve as a strong candidate for cancer treatment due to its strong proteasome inhibitory potential. Proteasomes are responsible for the degradation of many cytoplasmic proteins and for processing of foreign proteins prior to the deployment of cellular immune responses (Tanaka et al. Citation2012). Human cancer cells possess elevated levels of proteasome activity; therefore, the inhibition of proteasome could be a novel approach for treatment of cancer (Zhao et al. Citation2015). Proteasome inhibitors have been reported to overcome various types of drug resistance in cancer cells (Zhao et al. Citation2015); thus, various types of anticancer drugs could be conjugated with synthesized Fe3O4 magnetic nanoparticles and utilized in the treatment of cancer.
Figure 3. Proteasome inhibitory (A) and antioxidant potential (B–E) of Fe3O4 nanoparticles. FeNP-10 and −100: Fe3O4 nanoparticle at a concentration of 10 and 100 μg/mL, respectively; Epox-1 and −10: epoxomicin at a concentration of 0.56 and 5.56 μg/mL, respectively; (B) ABTS radical scavenging assay; (C) Nitric oxide scavenging assay; (D) DPPH free radical scavenging assay; and (E) Reducing power assay. Different superscript letters indicate significant differences at P < 0.05.
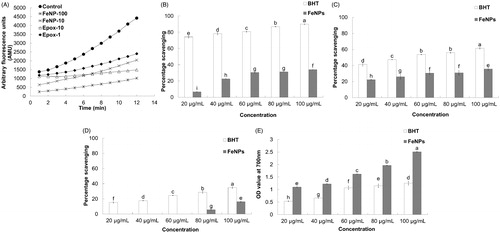
The Fe3O4 magnetic nanoparticles exhibited moderate ABTS radical scavenging potential of 34.03% at 100 μg/mL, whereas the BHT taken as the reference standard showed high ABTS radical scavenging of 89.08% at the same concentration (). The lower ABTS scavenging potential of the Fe3O4 magnetic nanoparticles compared to BHT might have been due to the presence of different functional groups attached to the Fe3O4 magnetic nanoparticles, which affects its ability to react with ABTS radicals, or due to the stereo selectivity of the radicals (Adedapo et al. Citation2008). The Fe3O4 magnetic nanoparticles and BHT exhibited nitric oxide scavenging potential, with 35.67% and 61.47% scavenging being observed at 100 μg/mL, respectively (). This reactive nitric oxide shows increased reactivity when it comes in contact with oxygen and produces more reactive molecules, resulting in cellular damage, DNA fragmentation, and lipid peroxidation (Santiso et al. Citation2012). Thus, the nitric oxide scavenging potential of Fe3O4 magnetic nanoparticles could make it a potential candidate for use as an antioxidant component that can be utilized in drug delivery. The Fe3O4 magnetic nanoparticles exhibited a very low DPPH radical scavenging potential of only 16.13% at 100 μg/mL (). The low DPPH radical scavenging potential of Fe3O4 magnetic nanoparticles may be attributed to the stereo selectivity of the bioactive compounds, which are present in the surface of Fe3O4 magnetic nanoparticles as capping agents’ of the DPPH free radicals (Adedapo et al. Citation2008). The Fe3O4 magnetic nanoparticles exhibited a strong reducing power relative to the reference compound BHT at all tested concentrations (). This strong reducing power of the Fe3O4 magnetic nanoparticles may be correlated with their high antioxidant capacity (Srivastava et al. Citation2006).
The Fe3O4 nanoparticles at a concentration of 25 μg and the standard antibiotics (kanamycin and rifampicin) at a concentration of 5 μg/disc did not exert any antibacterial activity when tested separately against the five foodborne pathogenic bacteria (). The synergistic antibacterial potentials of Fe3O4 nanoparticles mixed with the standard antibiotics kanamycin or rifampicin against the five foodborne pathogenic bacteria are presented in and . Fe3O4 nanoparticles (25 μg) combined with kanamycin (5 μg) exerted strong antibacterial activity against all tested foodborne pathogenic bacteria, with inhibition zones between 9.87 and 18.86 mm in diameter ( and ). When Fe3O4 nanoparticles (25 μg) were mixed with rifampicin (5 μg), the mixture only exerted antibacterial activity against S. aureus ATCC 49444, resulting in a 20.90 mm inhibition zone ( and ). Pathogenic bacteria are known to be responsible for different types of foodborne illness and food poisoning (Kim et al. Citation1995), and the use of different standard antibiotics to control the adverse effect of bacteria in food has led to the advent of resistant strains that can be able to survive and even multiply in the presence of an antibiotic (Hwang et al. Citation2012). The applications of Fe3O4 nanoparticles together with conventional antibiotics can exert synergistic effect, enabling a reduction in doses of antibiotics. This, in turn, can lead to decreased occurrence of resistant bacteria and mammalian cell toxicity.
Figure 4. Synergistic antibacterial and anticandidal potential of Fe3O4 nanoparticles. (A) Fe3O4 nanoparticles (25 μg) + kanamycin (5 μg); (B) Fe3O4 nanoparticles (25 μg) + rifampicin (5 μg); and (C) Fe3O4 nanoparticles (50 μg) + amphotericin b (5 μg).
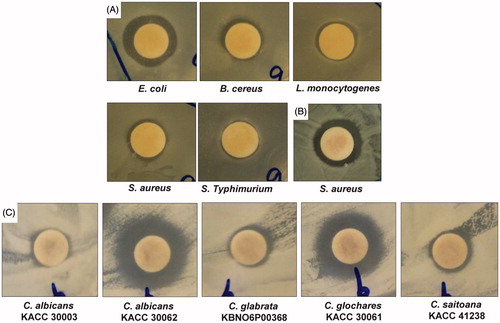
Table 1. Synergistic antibacterial and anticandidal activity of Fe3O4 nanoparticles against foodborne pathogenic bacteria.
Neither Fe3O4 magnetic nanoparticles at 50 μg/disc nor the standard antifungal agent amphotericin b at 5 μg/disc exerted anticandidal effects against the tested Candida species ( and ). However, when Fe3O4 magnetic nanoparticles (50 μg) and amphotericin b (5 μg) were mixed together, the mixture displayed high synergistic positive inhibition activity against all fungal pathogens, generating zones of inhibition between 9.37 and 16.69 mm in diameter ( and ). Similar study on the use of metal nanoparticles combined with antifungal agents has been reported previously (Vazquez-Munoz et al. Citation2014). The use of Fe3O4 magnetic nanoparticles in combination with antifungal drugs may be beneficial in clinical applications, since the amount of both the agents can be substantially reduced and thus potentially avoid the adverse effects caused by the use of high doses of drugs (Gajbhiye et al. Citation2009). The Candida species is the most representative model of pathogenic yeast in humans, and the management of Candida infections faces a number of problems, including resistance of Candida to commonly used antifungal drugs, availability of a limited number of effective antifungal drugs and their potential toxicity, and high cost of antifungal drugs (Khan et al. Citation2003). Thus, the potential anticandidal activity of Fe3O4 magnetic nanoparticles along with the lower concentration of amphotericin b could be beneficial for drugs toxicity toward the Candida species.
Conclusions
In the present investigation, synthesis of Fe3O4 nanoparticles was conducted by a green technology procedure utilizing the aqueous extract of the ear leaves of corn. The developed method is a novel approach for utilization of biological wastes in the synthesis of nanoparticles. The bioactive compounds from the ear leaves of the Z. mays plant played a major role in the reduction and stabilization of the nanoparticles. The synthesized Fe3O4 nanoparticles were characterized by UV-Vis spectroscopy, FE-SEM, EDX, FT-IR, TG/DTG, VSM, and XRD analysis. The Fe3O4 nanoparticles displayed strong proteasome inhibitory potential and can therefore be used as a potential candidate in drug delivery for cancer treatments. Its significant antioxidant activity in terms of its strong DPPH radical, nitric oxide, ABTS radical scavenging and reducing power could make it a promising candidate for biomedical and pharmaceutical applications. Furthermore, the strong synergistic antibacterial and anticandidal potential of Fe3O4 nanoparticles could be beneficial for its application in formulation of drugs utilizing low concentration of antibiotics and anticandidal drugs in order to minimize drug resistant and toxicity.
Funding information
This work was supported by a grant from the Systems and Synthetic Agro-biotech Center through the Next-Generation BioGreen 21 Program (PJ011117012015), Rural Development Administration, Republic of Korea.
Disclosure statement
The authors declare that they have no competing interests.
References
- Adedapo AA, Jimoh FO, Afolayan AJ, Masika PJ. 2008. Antioxidant activities and phenolic contents of the methanol extracts of the stems of Acokanthera oppositifolia and Adenia gummifera. BMC Complement Altern Med. 8:54.
- Aftabtalab A, Sadabadi H. 2015. Application of magnetite (Fe3O4) nanoparticles in hexavalent chromium adsorption from aquatic solutions. J Pet Environ Biotechnol. 6:200.
- Arum Y, Oh YO, Kang HW, Ahn SH, Oh J. 2015. Chitosan-coated Fe3O4 magnetic nanoparticles as carrier of cisplatin for drug delivery. Fish Aquat Sci. 18:89–98.
- Atta AM, Al-Lohedan HA, Al-Hussain SA. 2015. Functionalization of magnetite nanoparticles as oil spill collector. Int J Mol Sci. 16:6911–6931.
- Awwad AM, Salem NM. 2012. A green and facile approach for synthesis of magnetite nanoparticles. Nanosci Nanotechnol. 2:208–213.
- Basavegowda N, Magar KBS, Mishra K, Lee YR. 2014. Green fabrication of ferromagnetic Fe3O4 nanoparticles and their novel catalytic applications for the synthesis of biologically interesting benzoxazinone and benzthioxazinone derivatives. New J Chem. 38:5415–5420.
- Chen D, Ping Dou Q. 2010. The ubiquitin-proteasome system as a prospective molecular target for cancer treatment and prevention. Curr Protein Pept Sci. 11:459–470.
- Chen X, Klingeler R, Kath M, El Gendy AA, Cendrowski K, Kalenczuk RJ, Borowiak-Palen E. 2012. Magnetic silica nanotubes: synthesis, drug release, and feasibility for magnetic hyperthermia. ACS Appl Mater Interfaces. 4:2303–2309.
- Deb S. 2014. Synthesis of silver nano particles using Murraya koenigii (green curry leaves), Zea mays (baby corn) and its antimicrobial activity against pathogens. Int J PharmaTech Res. 6:91–96.
- Dizaji AN, Yilmaz M, Piskin E. 2015. Silver or gold deposition onto magnetite nanoparticles by using plant extracts as reducing and stabilizing agents. Artif Cells Nanomed Biotechnol. 24:1–7.
- Dung DTK, Hai TH, Phuc LH, Long BD, Vinh LK, Truc PN. 2009. Preparation and characterization of magnetic nanoparticles with chitosan coating. J Phys Conf Ser. 187:012036.
- Gajbhiye M, Kesharwani J, Ingle A, Gade A, Rai M. 2009. Fungus-mediated synthesis of silver nanoparticles and their activity against pathogenic fungi in combination with fluconazole. Nanomed Nanotechnol Biol Med. 5:382–386.
- Hwang I, Hwang JH, Choi H, Kim KJ, Lee DG. 2012. Synergistic effects between silver nanoparticles and antibiotics and the mechanisms involved. J Med Microbiol. 61:1719–1726.
- Jayanthi SA, Nathan DMGT, Jayashainy J, Sagayaraj P. 2015. A novel hydrothermal approach for synthesizing a-Fe2O3, g-Fe2O3 and Fe3O4 mesoporous magnetic nanoparticles. Mater Chem Phys. 162:316–325.
- Khan ZU, Chandy R, Metwali KE. 2003. Candida albicans strain carriage in patients and nursing staff of an intensive care unit: a study of morphotypes and resistotypes. Mycoses. 46:476–486.
- Kim J, Marshall MR, Wei C. 1995. Antibacterial activity of some essential oil components against five foodborne pathogens. J Agri Food Chem. 43:2839–2845.
- Koutzarova T, Kolev S, Ghelev C, Paneva D, Nedkov I. 2006. Microstructural study and size control of iron oxide nanoparticles produced by microemulsion technique. Phys Status Solidi C. 3:1302–1307.
- Leela A, Vivekanandan M. 2008. Tapping the unexploited plant resources for the synthesis of silver nanoparticles. Afr J Biotechnol. 7:3162–3165.
- Makhija IK, Aswatha-Ram HN, Shreedhara CS, Vijay Kumar S, Devkar R. 2011. In vitro antioxidant studies of sitopaladi churna, a polyherbal ayurvedic formulation. Free Rad Antioxid. 1:37–41.
- Murray PR, Baron EJ, Pfaller MA, Tenover FC, Yolke RH. 1995. Manual of Clinical Microbiology. 6th ed. Washington (DC): ASM Press.
- Naqvi SZH, Kiran U, Ali MI, Jamal A, Hameed A, Ahmed S, Ali N. 2013. Combined efficacy of biologically synthesized silver nanoparticles and different antibiotics against multidrug-resistant bacteria. Int J Nanomed. 8:3187–3195.
- Oberdorster G, Oberdorster E, Oberdorster J. 2005. Nanotoxicology: an emerging discipline evolving from studies of ultrafine particles. Environ Health Perspect. 113:823–839.
- Oh J, Feldman MD, Kim J, Kang HW, Sanghi P, Milner TE. 2007. Magneto-motive detection of tissue-based macrophages by differential phase optical coherence tomography. Lasers Surg Med Surg. 39:266–272.
- Patra JK, Baek KH. 2015. Novel green synthesis of gold nanoparticles using Citrullus lanatus rind and investigation of proteasome inhibitory activity, antibacterial, and antioxidant potential. Int J Nanomed. 10:7253–7264.
- Patra JK, Kim SH, Baek KH. 2015. Antioxidant and free radical-scavenging potential of essential oil from Enteromorpha linza L. prepared by microwave-assisted hydrodistillation. J Food Biochem. 39:80–90.
- Philip D, Unni C, Aromal SA, Vidhu VK. 2011. Murraya Koenigii leaf-assisted rapid green synthesis of silver and gold nanoparticles. Spectrochim Acta A. 78:899–904.
- Rahman O, Mohapatra SC, Ahmad S. 2012. Fe3O4 inverse spinal super paramagnetic nanoparticles. Mater Chem Phys. 132:196–202.
- Santiso R, Tamayo M, Gosalvez J, Johnston S, Marino A, Fernandez C, Losada C, Fernandeza JL. 2012. DNA fragmentation dynamics allows the assessment of cryptic sperm damage in human: evaluation of exposure to ionizing radiation, hyperthermia, acidic pH and nitric oxide. Mutat Res. 734:41–49.
- Senthil M, Ramesh C. 2012. Biogenic synthesis of Fe3O4 nanoparticles using Tridax procumbens leaf extract and its antibacterial activity on Pseudomonas aeruginosa. Digest J Nanomater Biostruct. 7:1655–1660.
- Shankar SS, Rai A, Ahmad A, Sastry M. 2004. Rapid synthesis of Au, Ag and bimetallic Au core-Ag shell nanoparticles using Neem (Azadirachta indica) leaf broth. J Colloid Interface Sci. 275:496–502.
- Singh P, Kim YJ, Wang C, Mathiyalagan R, El-Agamy Farh M, Yang DC. 2015. Biogenic silver and gold nanoparticles synthesized using red ginseng root extract, and their applications. Artif Cells Nanomed Biotechnol. [Epub ahead of print]. doi:10.3109/21691401.2015.1008514.
- Srivastava A, Harish SR, Shivanandappa T. 2006. Antioxidant activity of the roots of Decalepis hamiltonii (Wight & Arn). LWT-Food Sci Technol. 39:1059–1065.
- Sun L, Zhang J, Lu X, Zhang L, Zhang Y. 2011. Evaluation to the antioxidant activity of total flavonoids extract from persimmon (Diospyros kaki L.) leaves. Food Chem Toxicol. 49:2689–2696.
- Tanaka T, Nakatani T, Kamitani T. 2012. Inhibition of NEDD8-conjugation pathway by novel molecules: potential approaches to anticancer therapy. Mol Oncol. 6:267–275.
- Thaipong K, Boonprakob U, Crosby K, Cisneros-Zevallos L, Byrne DH. 2006. Comparison of ABTS, DPPH, FRAP, and ORAC assays for estimating antioxidant activity from guava fruit extracts. J Food Comp Anal. 19:669–675.
- Thomasa T, Kanotha BP, Nijasb CM, Joyd PA, Josephc JM, Kuthirummale N, Thachil ET. 2015. Preparation and characterization of flexible ferromagnetic nanocomposites for microwave applications. Mater Sci Eng B. 200:40–49.
- Vazquez-Munoz R, Avalos-Borja M, Castro-Longoria E. 2014. Ultrastructural analysis of Candida albicans when exposed to silver nanoparticles. PLoS One. 9:e108876.
- Xu B, Dou HJ, Tao K, Sun K, Ding J, Shi WB, et al. 2011. Two-in-one, fabrication of Fe3O4/MePEG-PLA composite nanocapsules as a potential ultrasonic/MRI dual contrast agent. Langmuir. 27:12134–12142.
- Zhang JQ, Zhang ZR, Yang H, Tan QY, Qin SR, Qiu XL. 2005. Lyophilized paclitaxel magnetoliposomes as a potential drug delivery system for breast carcinoma via parenteral administration: in vitro and in vivo studies. Pharm Res. 22:573–583.
- Zhao J, Luque R, Qi W, Lai J, Gao W, Gilani MRHS, Xu G. 2015. Facile surfactant-free synthesis and characterization of Fe3O4@3-aminophenol–formaldehyde core–shell magnetic microspheres. J Mater Chem A. 3:519–524.