Abstract
Cryogels are used quite a lot nowadays for adsorption studies as synthetic adsorbents. In this study, lipase enzyme (obtained from Candida cylindracea) adsorption capacity of poly(2-hydroxyethyl methacrylate-N-methacryloyl-L-tryptophan), poly(HEMA-MATrp), and Cu(II) ions immobilized poly(HEMA-MATrp), poly(HEMA-MATrp)-Cu(II), cryogel membranes were synthesized to determine and compare the adsorption behavior of lipase enzyme. In this regard, the effect of pH, interaction time, lipase initial concentration, temperature and ionic strength on the adsorption capacity of these membranes was investigated. Maximum lipase enzyme adsorption capacities of poly(HEMA-MATrp) and poly(HEMA-MATrp)-Cu(II) cryogel membranes were determined as 166.4 mg/g and 196.4 mg/g, respectively.
Keywords:
Introduction
Enzyme industry in the global market has reached the figure of 7 billion by the year 2013 (World Enzymes Market Citation2009). Today, one of the most crucial enzymes in the industry is lipase (EC 3.1.1.3). Lipase enzyme is a chemical-, regio-, and an enantio-selective molecule involved in both hydrolysis and synthesis reactions. This enzyme catalyzes the hydrolysis of water-insoluble triglycerides into free fatty acids and glycerol and has functions for emulsifying lipid substrate interface. Lipase enzyme obtained from higher animals has been well characterized (Bacha et al. Citation2005, Fendri et al. Citation2006, Mejdoubet al. Citation1994). There is much less information about lipase owned by lower animals. Due to the determination of lipase activity in the gastric water, there are many research on lipases of invertebrates such as some crustaceans (Homarus americanus) and molluscs (Aplysia californica) (Brockerhoff et al. Citation1970, Brockerhoff and Jensen Citation1974).
Some of the lipase enzymes have a versatile feature, such as catalyzing reactions of aminolysis, alcoholysis, esterification and transesterification (Dunn Citation2012, Jaeger and Eggert Citation2002, Kapoor and Gupta Citation2012, Solano et al. Citation2012). Therefore, this enzyme is used for biosynthetic industrial applications, oil processes, production of surfactants and the preparation of enantiomerically pure compounds (Brabcová et al. Citation2012, Dunn Citation2012, Solano et al. Citation2012). It is used in pharmaceutics for the production of drugs (Björkling et al. Citation1991). Active functional property of lipases in both aqueous and nonaqueous solvent systems comes into prominence (Jaeger et al. Citation1994).
The activity of lipase enzyme is inhibited or stimulated by cofactors (Patil et al. Citation2011). Metal ions are capable of inhibiting or stimulating the production of microbial enzymes. Ca2+ is an important ion for enzyme activity. While Ca2+ is an activator metal ion; Zn2+, Fe2+, and Fe3+ ions inhibit strongly enzyme activity of lipase (Verma et al. Citation2012). Titrimetric and spectrophotometric methods are used for the determination of hydrolytic activity of the lipase enzyme (Schmidt and Bornscheuer Citation2005). Especially, p-nitrophenyl alkanoates esters are often preferred among colorific substrate for spectrophotometric lipase activity determination (Furutani et al. Citation1995).
In recent years, especially adsorption, precipitation using ammonium sulfate and chromatographic techniques has been used for the purification of proteins (Garner and Smith Citation1970, Genest et al. Citation1998, Rovery et al. Citation1978, Segura et al. Citation2006). These methods are not only time-consuming, but also very expensive in terms of cost.
Cryogels are hydrophilic materials having macropores. The preparation procedure is based on the idea that solvent freezes under cryogenic conditions and dissolves again at room temperature so as to create gaps in the structure due to the frozen solvent (Aslıyüce et al. Citation2010, Berillo et al. Citation2012, Gupta et al. Citation2013, Hajizadeh et al. Citation2013, Kirsebom et al. Citation2010, Yun et al. Citation2011). On account of the large surface area and porous structure, cryogels have been used very extensively for studies investigating cells (Hwang et al. Citation2010), solid-phase extraction (Kueseng et al. Citation2010) and for chromatographic applications (Akduman et al. Citation2013, Asliyuce et al. Citation2012, Asliyuce et al. Citation2013, Uzun et al. Citation2013, Yao et al. Citation2006). Cryogel-based biosensors (Flexer et al. Citation2011, Tsujimura et al. Citation2010), chitosan (Hedström et al. Citation2008) and polyvinyl alcohol cryogels (Tsai et al. Citation2007) were also studied.
In the scope of this study, poly(2-hydroxyethyl methacrylate-N-methacryloyl-L-tryptophan), poly(HEMA-MATrp), and Cu(II) ions immobilized poly(HEMA-MATrp), poly(HEMA-MATrp)-Cu(II), cryogels were synthesized via copolymerization process and the adsorption efficiencies of these polymeric structures toward lipase enzyme were investigated and compared. In addition, optimum pH, interaction time and temperature, initial lipase concentration and ionic strength for lipase adsorption capacities of cryogels were determined. With this method, it is aimed to designate a method for the separation and purification of lipase as an alternative to separation and purification methods in the literature. Simplicity and separation speed of cryogel have been reflected in the separation and purification of lipase from biological samples, and by this way, a very important contribution would be made to literature in respect to huge demand for the separation and purification of this enzyme.
Materials
Lipase (from Candida cylindracea); 2-hydroxyethyl methacrylate (HEMA), ethylene glycol dimethacrylate (EGDMA), L-tryptophan, methacryloyl chloride, sodium nitrite (NaNO2), potassium carbonate (K2CO3), ethyl acetate, N,N,N0,N0-tetramethyl ethylene diamine (TEMED), ammonium persulfate (APS), sodium lauryl sulfate (SLS), diethyl ether, copper(II) sulfate pentahydrate (CuSO4.5H2O), ethyl acetate, cyclohexane, sodium chloride, ethylene glycol, and p-nitriphenyl palmitate (p-NPP) were obtained from Sigma-Aldrich (Taufkirchen, Germany). For spectrophotometric measurements, Spektro Double Beam UV-VIS PC 8 Scanning Auto Cell UVD-3200 (Los Angeles, CA) device was used. N-Methacryloyl-L-tryptophan (MATrp) was synthesized according to the literature in the laboratory (Yılmaz et al. Citation2009). Wheat germ has been obtained from local market. All other chemicals are of analytical grade.
Method
MATrp synthesis
To synthesize functional monomer (MATrp), L-tryptophan (5 g) and NaNO2 (0.2 g) were dissolved in aqueous K2CO3 (30 mL, 5% (w/v)) solution and then cooled to 0 °C (Yılmaz et al. Citation2009). Methacryloyl chloride (4 mL) was added slowly under inert atmosphere before stirring magnetically for 2 h at room temperature. After adjusting pH of the solution (pH: 7.0), extraction process was conducted with ethyl acetate. By removing liquid phase using an evaporator, MATrp was crystallized with ether and cyclohexane.
Synthesis of poly(HEMA-MATrp) cryogels
About 50 mg of functional monomer N-methacryloyl-L-tryptophan (MATrp) and 2.5 mL of carrier monomer (HEMA) and 2.5 mL of distilled water was mixed. To this solution, 0.5 g of SLS, 0.6 mL of EGDMA and 9.4 mL of distilled water were added and to obtain a homogeneity mixture was stirred using a magnetic stirrer. By this solution, monomer: volume ratio was adjusted to 21% by w/v. The mixture was kept for 20 min in an ice bath. About 10 mg of APS and 50 μL of TEMED were added and then frozen for 24 h at 12 °C. Cryogels prepared were cut into in the shape of a disk and washed several times with distilled water.
Immobilization of Cu(II) ions onto poly(HEMA-MATrp) cryogels
For this operation, cryogels were placed in 25 mL of aqueous solution of CuSO4.5H2O compound (100 mg/L) and stirred continuously with a magnetic stirrer for 2 h. The color of cryogels was turned from white to light blue and finally followed by washing with distilled water ().
Purification lipase from wheat germ
The wheat germ of 5 g was extracted by pulverizing in 0.1 M (pH 7.0) phosphate buffer of 50 mL (0.0034 moles of NaH2PO4 + 0.0065 moles of Na2HPO4 + 100 mL distilled water) and washed with acetone with the ratio of 10:1 (w/w) to purify from its oil. Suspension mixture was centrifuged at 3000 rpm at 25 °C for 15 min for the removal of unwanted particles. The precipitate was removed and the total enzyme activity and the protein amount of supernatant were quantified (). The Bradford method was used to determine the amount of protein at 595 nm (Bradford Citation1976). Then, the resultant mixture was centrifuged again at 9000 rpm at 25 °C by adding ammonium sulfate solution of 30% (w/v). The precipitate obtained was dissolved in 0.1 M pH 7.0 phosphate buffer of 10 mL and the total enzyme activity and the amount of protein in the solution were determined. The samples of 1 mL were taken and each was mixed with 0.1 M pH 6.0 and pH 7.0 phosphate buffer solutions of 4 mL. The adsorption process was performed at 25 °C for the lipase solution at pH 6.0 and pH 7.0 for 40 and 30 min using poly(HEMA-MATrp) and poly(HEMA-MATrp)-Cu(II) cryogels, respectively. In the last stage, the desorption process was conducted for poly(HEMA-MATrp) and poly(HEMA-MATrp)-Cu(II) cryogels using 0.1 M ethylene glycol and 1.0 M NaCl solutions of 10 mL each, respectively, and similarly, the total enzyme activity and the protein amount of the medium were determined.
Table 1. Purification steps of lipase from wheat germ.
Characterization of poly(HEMA-MATrp) cryogels
Swelling test
In order to determine the water retention capacity of poly(HEMA-MATrp) cryogels, dry cryogels were placed in distilled water within water bath with constant temperature after weighed carefully and waited at 25 °C for 30 min. Then, a water-swelled membrane was taken and re-weighed. According to the difference obtained in terms of weight, water retain capacity was estimated from the following equation.
(1)
In this equation, Wo and Ws refer to weights of dry and swollen cryogels (g).
Determination of surface morphology
Surface morphology of cryogels was investigated using scanning electron microscope (SEM) (Carl Zeiss AG-EVO® 50 Series, Germany). Firstly, samples were dried via lyophilization process and were prepared for SEM analysis. Then, an appropriate amount of sample was placed on SEM holder, and it is coated with a thin gold layer in vacuum for 2 min. The sample prepared was then placed on the SEM and images were taken at desired magnification.
Elemental analyses
To determine the Trp composition of poly(HEMA-MATrp) cryogels, elemental analysis (St. Joseph, MI) was used and nitrogen incorporation was concerned for the estimation of Trp incorporation.
FTIR analyses
Fourier transform infrared spectroscopy (FTIR) (Thermo Scientific, Nicholet IS10, Paisley, PA) was used for the characterization of cryogels synthesized. For this operation, dry cryogel sample of 2 mg was obtained in the form of the pellet after mixing with 98 mg of dry KBr powder (IR-Grade) and then FTIR spectra were obtained.
Adsorption experiment
Adsorption experiments were conducted via the batch system. Samples were prepared by mixing 1 mL of lipase and 4 mL buffer of the solution. This solution was equilibrated via stirring with a magnetic stirrer for 15 min. and adsorption process was conducted by the addition of a cryogel disc.
Adsorption capacity was calculated by the following equation.
(2)
Wherein Q is adsorption capacity (mg/g), Ci is the concentration of lipase before adsorption (mg/L), Cf is the concentration of lipase after adsorption (mg/L), V is the volume of adsorption medium (L), and m is the mass of cryogel (g).
Desorption and repeated use
Desorption of lipase enzyme adsorbed by poly(HEMA-MATrp) cryogels studied via the batch system. Membranes adsorbed enzyme were mixed continuously with 0.1 M ethylene glycol solution (for poly(HEMA-MATrp) cryogels) (Altıntaş and Denizli Citation2009) and 1 M NaCI solution (for poly(HEMA-MATrp)-Cu(II) cryogels) (Baydemir et al. Citation2013) in 10 mL desorption media for 1 h. To determine the reusability of membranes, adsorption–desorption cycle was repeated four times using same membranes. Between two successive adsorption–desorption cycle, cryogels were washed with 0.1 M NaOH (10 mL, 30 min.) for regeneration and with buffer solution (pH: 6.0, 10 mL, 30 min.) for stabilization.
Desorption ratio is calculated as follows:
(3)
Determination of lipase activity
The hydrolysis of p-nitrophenyl palmitate (p-NPP) is the indicator of the lipase activity by means of the spectrophotometric methods. For the preparation of the solution of p-NPP, the solution of 10 mL isopropanol with p-NPP of 30 mg was mixed with 0.05 M phosphate buffer (pH: 8.0) of 90 mL consisting the Na-deoxycholate of 200 mg and gum arabic (the final concentration of p-NPP is 0.8 mM) of 100 mg. It is important to determine the liberation of 1 μmol of p-nitrophenol per minute (ɛ = 18.5 mM−1 cm−1) at the reaction time of three minutes, the absorbance of which can be measured at the wavelength of 410 nm at laboratory conditions where the experiments were conducted. The quantity of enzyme estimated after this measurement can be expressed as the one activity unit (1 U) of the enzyme. There is a p-NPP solution of 900 μL and lipase solution (the concentration of lipase is 0.05 mg/mL) of 100 μL in the reaction mixture (Karadzic et al. Citation2006). The activity of lipase desorbed from the polymeric structure was estimated through the p-NPP solution (the concentration of p-NPP was 0.8 mM in pH: 8.0 phosphate buffer) of 2 mL. The reaction was ended by the addition of sodium carbonate solution (0.5 M). The supernatant was obtained after centrifugation at 10.000 rpm for 10 min and the 10-fold dilution was applied to measure activity.
Determination of the lipase kinetic parameters
The relation between the enzyme activity and the substrate concentration was estimated via using Michaelis–Menten kinetics. Here, the enzymes are free and immobilized lipase. For the determination of the kinetic parameters given by the Lineweaver–Burk equation below, vmax which is the units of activity per milligram of protein (Umg−1) and Km, which is the substrate concentration, the method described previously was applied.
(4)
SDS-PAGE analysis of lipase desorbed from cryogels
For this process, 12% running gel (0.375 M Tris-HCl, pH: 8.8, 12% acrylamide) was poured between two glass using a syringe. To stop air contact of the gel completely, it is covered with distilled water. After 30 min, time for total polymerization of running gel, all apparatus was evacuated and all water covering gel was poured off. After completion of welling process to install samples, 5% loading gel (0.125 M Tris-HCl, pH 6.8, 5% acrylamide) gel was poured onto running gel. Electrophorese buffer (25 mM Tris, 192 mM glycine, 0.1% SDS, pH 8.3) was used to wash wells. The inside of the tank was filled with buffer containing SDS and 8 μL of the sample (desorbed lipase solution) and 8 μL of the sample buffer (prepared with SDS) were mixed and built into the gel by means of electrophoresing needles. The marker was installed in the first well. Installed samples were subjected to electrophorese process at 150 A through the power supply, for 3.5–4 h. Image of bromide phenol blue was followed on the gel. After running process was completed, gel was carefully removed completely from the glass and stained for 4 h in a solution of 50% (v/v) methanol, 10% (v/v) acetic acid and 0.25% (w/v) Coomassie Brilliant Blue R-250 and destained using a 5% (v/v) methanol, 7.5% (v/v) acetic acid solution for 2 h, while the remainder was destained by soaking in distilled water. After they were photographed, the resulting bands were compared with marker band.
Results and discussion
Characterization of cryogels
Swelling test
Swelling test of poly(HEMA-MATrp) membranes was performed as mentioned previously. In this respect, the weight of dry and swelled cryogels was measured as 29.4 and 162.5 mg/disk, respectively. According to these values, water-swelling capacity of membranes was calculated as 453%, in another word, each gram of poly(HEMA-MATrp) cryogel can absorb 4.53 g of water. Additionally, weight of dry and water-swelled poly(HEMA-MATrp)-Cu(II) cryogel membrane were measured as 29.8 and 167.2 mg/disc, respectively. From these results, water retention capacity of the membranes was calculated as 461% and so each gram of poly(HEMA-MATrp)-Cu(II) cryogel can absorb 4.61 g of water. As can be seen from the results, the number of water retaining group within polymeric structure was increased due to the incorporation of Cu(II) ions into the poly(HEMA-MATrp) structure.
Surface morphology
SEM images of poly(HEMA-MATrp) and poly(HEMA-MATrp)-Cu(II) cryogels showing the surface morphology of membranes are given below (). As illustrated in the figure, desired macroporous structures were obtained. Porous cell surface and interconnected flow channels can be seen clearly. The adsorption process was conducted very easily and in a very short time interval through these macropores of cryogel structure.
Elemental analyses
As mentioned earlier, elemental analysis was performed for the determination of MATrp incorporation amount onto poly(HEMA-MATrp) cryogel structure. The amount of MATrp within the polymeric structure was determined via nitrogen stoichiometry since the fact that only nitrogen source within the polymeric structure is MATrp. The value obtained from the elemental analysis is 0.4–0.8% N incorporation. In the case of considering the amount of MATrp used during the synthesis, this value is quite higher than needed. We have thought that there may be some impurity. Actually, the MATrp incorporation is quite satisfactory. Moreover, Cu(II)-chelating capacity of poly(HEMA-MATrp) cryogel structure was determined as approximately 0.2–0.4 μmol/g. It can be concluded that the interaction between functional monomer (MATrp) and ions (Cu(II)) is quite effective.
FTIR analysis
FTIR spectrums of poly(HEMA), poly(HEMA-MATrp), and poly(HEMA-MATrp)-Cu(II) cryogels are shown in . From the figure for three of the spectrum, it is remarkable that there are O-H stretching (alcohol, 3308–3392 cm−1), C-H stretching (alkane, 2947–2949 cm−1) and C = O (carbonyl, 1715–1716 cm−1). Thus, the band obtained from the spectrum of poly (HEMA-MATrp) cryogel is quite sharp and notable as compared to that of poly(HEMA) cryogels. This result may be the explanation of additional carbonyl groups within the structure of poly(HEMA-MATrp) cryogels (Baydemir et al. Citation2013). Additionally, C = C stretching (alkene, 1635 cm−1), N-H bending (amine; 1521 cm−1), C-C stretching (aromatic ring; 1558, 1506), C-N stretching (aromatic amine; 1472 cm−1) and O-H bending (carboxylic acid; 946 cm−1) are quite remarkable for the spectrum of poly (HEMA-MATrp) cryogels. From these results, it can be concluded that functional monomer, that is, MATrp was incorporated into poly (HEMA) structure successfully. In addition to the spectrum of poly(HEMA-MATrp), for poly(HEMA-MATrp)-Cu(II) cryogels, there is an additional band at 473 cm−1 due to Cu-N bond.
Adsorption experiments
Effect of pH
To examine the influence of pH on a number of adsorption, experiments were carried out in the range of pH: 4.0–9.0. The highest adsorption amount was determined at pH: 6.0 for poly(HEMA-MATrp) cryogels (). According to this result, it may conclude that lipase molecule as a target and the functional monomer (MATrp), charge distribution, and the relevance of hydrophobic interactions are appropriate to have acceptable results. At pH 6.0, indole ring within the structure of both lipase and MATrp have almost no net charge. Hydrophobic interactions are more successful under conditions with no net charge, that is, neutral. Therefore, the highest adsorption capacity was observed at this pH. At increasing and decreasing the pH value, charge distribution has been changed and thus adsorption capacity was decreased.
Figure 4. Effect of pH on adsorption of lipase onto poly(HEMA-MATrp) (Clipase: 1.5 mg/mL, interaction time: 40 min., temperature: 25 °C) and poly(HEMA-MATrp)-Cu(II) (Clipase: 2.0 mg/mL, interaction time: 30 min., temperature: 25 °C) cryogels.
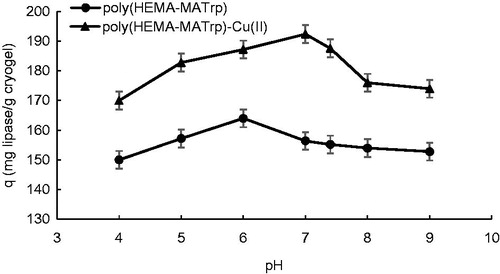
At the same time, as can be seen from , adsorption capacity of poly(HEMA-MATrp)-Cu(II) cryogels was reached the highest point at pH 7.0. At below and above of this value, Cu(II) ions may be dissolved or interacted with OH− ions, and thus, the possibility of Cu(II) ions to interact with lipase molecules will decrease. Therefore, because of these reasons for pH values other than 7.0, Cu(II) ions cannot make coordinated covalent bonds with lipase enzyme molecules. As a result of this study, pH 7.0 was determined as the optimum pH for the adsorption of lipase onto cryogels and this pH were studied for all remaining studies.
Effect of interaction time
Under optimum conditions achieved, adsorption process was performed at the time intervals of 5–90 min. Adsorption capacity values calculated in this period is given in . As seen from the figure, lipase adsorption on cryogels was taken place in a relatively short time. While the lipase adsorption capacity of poly(HEMA-MATrp) cryogels was achieved to at the end of the 40 min, that of poly(HEMA-MATrp)-Cu(II) cryogels was 193.2 mg/g at the end of the 30 min. After those points, there was no remarkable change observed. The reason for the achievement of maximum adsorption capacity within that much quite short time interval, large macropores and thus interconnected flow channels within the sponge-like structure of polymeric cryogels.
Effect of temperature
To examine the effect of temperature on the adsorption, adsorption process was conducted at the temperature of 4, 13, 25, and 35 °C. It was noticed from the result of the experiments that the amount of lipase adsorption onto poly(HEMA-MATrp) cryogels was increased with increasing temperature as expected for hydrophobic interaction (from 155.2 mg/g to 170.8 mg/g) (). As it is well known, there is a direct proportion between hydrophobic interactions and temperature. Additionally, to determine the effect of temperature on the lipase adsorption capacity of poly(HEMA-MATrp)-Cu(II) cryogels, adsorption process was conducted at same four different temperatures. As a result of experiments, as expected, the adsorption capacity of the cryogels was decreased with increasing temperature (from 197.2 mg/g to 187.2 mg/g), that is, adsorption capacity of the cryogels is inversely proportional to temperature (). The strength of coordinated covalent bond or similar interactions decreases with increasing temperature because of the fact that increasing temperature effects the bond energies so thus molecular stability and also the number of this kind of bonds decrease with increasing temperature due to broken bonds with temperature.
Effect of ionic strength
To investigate the effect of ionic strength, NaCI was used for experiments performed in this study. The salt concentration of 100–500 mM was chosen to estimate the amount of lipase adsorption. It was observed from the experiments that adsorption capacity was increased with increasing ionic strength (from 164.4 mg/g to 182.4 mg/g) (). This result was also proved that interaction between lipase and poly(HEMA-MATrp) is based on hydrophobic interaction. An explanation for this may be that the presence of salt makes the water distribution around polar (hydrophilic) group impaired, and provides the higher accessibility to apolar (hydrophobic) groups (Chen et al. Citation2007). Thus, because of the salt occurrence probability of apolar–polar interaction increases and due to these interactions, much higher adsorption capacity can be attained. As seen from , the adsorption capacity of poly(HEMA-MATrp)-Cu(II) was decreased with increasing salt concentration (from 193.6 mg/g to 170 mg/g). This result confirms the presence of electrostatic interactions in the adsorption process. Because charge distribution of polar groups (aspartate, lysine, arginine, etc.) on lipase enzyme was affected by the presence of ions (Na+ and CI−) coming from salt molecules in the adsorption medium, Na+, and Cl− ions may mask the possible interaction of lipase with Cu(II) ions by interaction with these groups. In addition, Cl− ions interact electrostatically with Cu(II) ions incorporated into the polymeric structure and because of this amount of lipase molecules to be bound to the structure will decrease. As a conclusion, increasing salt concentration (ionic strength) effect the adsorption capacity inversely.
Figure 7. Effect of ionic strength on adsorption of lipase onto poly(HEMA-MATrp) (pH: 6.0, interaction time: 40 min., CLipase: 1.5 mg/mL, temperature: 25 °C) and poly(HEMA-MATrp)-Cu(II) (pH: 7.0, interaction time: 30 min., CLipase: 2.0 mg/mL, temperature: 25 °C) cryogels.
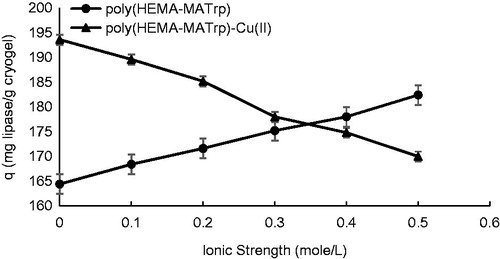
Figure 8. SDS-PAGE of desorbed lipase obtained from Candida cylindracea. Lane 1. Lipase, lysozyme, and hemoglobin marker, Lane 2: Lipase marker, Lane 3: Initial [(Before adsorption for poly(HEMA-MATrp)], Lane 4: Final [(After adsorption for poly(HEMA-MATrp)], Lane 5: Desorbed sample [(After dialysis for poly(HEMA-MATrp)], Lane 6: Initial [(Before adsorption for poly(HEMA-MATrp)-Cu(II)], Lane 7: Final [(After adsorption for poly(HEMA-MATrp)-Cu(II)], Lane 8: Desorbed sample [(After dialysis for poly(HEMA-MATrp)-Cu(II)].
![Figure 8. SDS-PAGE of desorbed lipase obtained from Candida cylindracea. Lane 1. Lipase, lysozyme, and hemoglobin marker, Lane 2: Lipase marker, Lane 3: Initial [(Before adsorption for poly(HEMA-MATrp)], Lane 4: Final [(After adsorption for poly(HEMA-MATrp)], Lane 5: Desorbed sample [(After dialysis for poly(HEMA-MATrp)], Lane 6: Initial [(Before adsorption for poly(HEMA-MATrp)-Cu(II)], Lane 7: Final [(After adsorption for poly(HEMA-MATrp)-Cu(II)], Lane 8: Desorbed sample [(After dialysis for poly(HEMA-MATrp)-Cu(II)].](/cms/asset/5cdcf2fa-fe0b-4192-b43e-54075ad71219/ianb_a_1161642_f0008_c.jpg)
Desorption and repeated use
As a result of four-cycle repeated use of same poly(HEMA-MATrp) cryogel, lipase enzyme was obtained at the recovery of 84% and adsorption capacity was decreased only 5.6% (from 163.6 mg/g to 156.4 mg/g). According to this result, it can be concluded that poly(HEMA-MATrp) cryogels have quite a high reusability ratio. Moreover, same procedure (four adsorption–desorption cycle) was repeated for poly(HEMA-MATrp)-Cu(II) cryogel. Lipase desorption ratio of cryogel was estimated as 79%. The lipase adsorption capacity of cryogels was decreased from 192.0 to 183.6 mg/g cryogel, it may be concluded from this result that there is no significant decrease observed for repeated use of the same cryogel. Considering all data with together, it may be concluded that there is a specific and reversible interaction between lipase enzyme and poly(HEMA-MATrp)- Cu(II) cryogels and also these cryogels have high reusability.
SDS-PAGE analysis of lipase desorbed from cryogels
As can be seen from , purification of lipase desorbed from polymeric structures was achieved successfully due to the same distance was covered for both of lipase desorbed from cryogel species (poly(HEMA-MATrp) and poly(HEMA-MATrp)-Cu(II)) and the standard lipase marker (58 kDa). These results can be evident for that adsorption–desorption cycle for lipase using poly(HEMA-MATrp) and poly(HEMA-MATrp)-Cu(II) cryogels were performed successfully.
Purification lipase from wheat germ
The total protein content of the crude extract (9.8 mg/mL) was decreased to 5.9 mg/mL by the process of ammonium sulfate precipitation and to the values 2.22 and 2.30 mg/mL after purification process achieved by poly(HEMA-MATrp) and poly(HEMA-MATrp)-Cu(II), respectively. UV-Vis spectra of solutions were taken for each purification step. It was observed that the peak intensity obtained at 280 nm was decreased depending on the decrease in the total protein amount (). Similarly, there was a slight decrease (28.3 U/mL, from 345.7 to 317.4 U/mL) observed in the enzymatic activity of crude extract (345.7 U/mL) after the process of ammonium sulfate precipitation; however, this value was decreased to 214.5 and 240.8 U/mL for the purification process achieved by the poly(HEMA-MATrp) and poly(HEMA-MATrp)-Cu(II) cryogels, respectively. Nevertheless, the specific activity value (35.3 U/mg) indicating the purification efficiency was estimated as 53.8, 96.6, and 104.7, and thus, purification factors based on these values as 1.52-, 2.74-, and 2.97-fold for the process of ammonium sulfate precipitation and purification processes via using poly(HEMA-MATrp) and poly(HEMA-MATrp)-Cu(II) cryogels, receptively. These results suggesting that the cryogels synthesized in this study adsorb the lipase successfully and selectively and thus can be used for the selective enzyme purification processes under mild conditions. Additionally, the chelated structure provided with Cu(II) ion appears to be advantageous (at least 8.4%) for the purification process.
Kinetic parameters
Nontreated and the desorbed lipases are in accordance with the Michaelis–Menten kinetics. The kinetic parameters of Lineweaver–Burk for both are given as the Km (0.392 for nontreated and 0.400 mM for desorbed lipase) and the Vmax (196.08 for nontreated and 166.67 Umg−1 for desorbed lipase) values that are almost the same. It is obvious in the light of these results that the affinity of the free and desorbed lipase is considerably similar. In this regard, there is no remarkable change observed in the enzyme activity of the lipase obtained at the end of the adsorption process conducted through poly(HEMA-MATrp) cryogels.
Adsorption isotherms
Adsorption isotherms were examined to characterize the interaction between lipase enzyme and cryogels. For Langmuir adsorption isotherm, adsorption phenomena are regarded to occur on the surface homogeneously and in a single layer, whereas for Freundlich isotherms, it occurs in multilayer and heterogeneous. Equations given below are used for Langmuir and Freundlich isotherms:
Langmuir equation:
If it is linearized;
the equation is obtained. The y-intercept of the graph of 1/Ceq versus 1/Qeq gives 1/Qmax and the slope of this graphs equals to 1/Qmax.b value. Here, Qeq is the amount of lipase adsorbed (mg/g), Ceq is the lipase concentration at equilibrium (mg/L), b is the Langmuir adsorption constant (L/mg), Qmax is the maximum adsorption capacity (mg/g).
Freundlich equation:
Here, Kf and n are Freundlich adsorption isotherm constants. n is the slope of the graph lnQeq versus lnCeq and lnKf is the intercepting point.
Adsorption and correlation constants needed for both adsorption isotherms were calculated and given in .
Table 2. Parameters for adsorption isotherms of poly(HEMA-MATrp) and poly(HEMA-MATrp)-Cu(II).
From the results obtained, correlation coefficient values for Langmuir isotherms (0.9565 and 0.9897) was found relatively higher than those of Freundlich adsorption isotherms (0.8798 and 0.9267) for both of poly(HEMA-MATrp) and poly(HEMA-MATrp)-Cu(II) cryogels, respectively. In addition, Qmax values obtained from calculations were determined as consistent with values obtained from experiments. According to these results, it may be concluded that the adsorption of lipase enzyme onto poly(HEMA-MATrp) and poly(HEMA-MATrp)-Cu(II) cryogels were achieved homogeneously and thus every single point on the surface of polymers have equal energies.
Conclusion
As a result of this study, it can be concluded that poly(HEMA-MATrp) and poly(HEMA-MATrp)-Cu(II) cryogels are quite effective for lipase enzyme separation and purification. It is estimated that the interaction between poly(HEMA-MATrp) cryogels and lipase occurs via hydrophobic indole ring of tryptophan on the cryogel membranes and hydrophobic groups in the structure of the lipase enzyme (amino acids and alkyl groups having hydrophobic side chains). At the same time, poly(HEMA-MATrp)-Cu(II) cryogels enable the electrostatic interaction between lipase enzyme and the cryogels. This behavior is confirmed by a decrease in the adsorption capacity with increasing temperature and ionic strength. These electrostatic interactions have positive effects on the adsorption capacity of poly(HEMA-MATrp) cryogels. Adsorption characteristics of cryogels for lipase enzyme are well matched with Langmuir adsorption model. According to this model, the lipase molecules were adsorbed onto poly(HEMA-MATrp) and poly(HEMA-MATrp)-Cu(II) cryogels via monolayer interaction pattern and homogeneously.
References
- Akduman B, Uygun M, Uygun DA, Akgöl S, Denizli A. 2013. Purification of yeast alcohol dehydrogenase by using immobilized metal affinity cryogels. Mater Sci Eng C Mater Biol Appl. 33:4842–4848.
- Altıntaş EB, Denizli A. 2009. Monosize magnetic hydrophobic beads for lysozyme purification under magnetic field. Mat Sci Eng C. 29:1627–1634.
- Asliyuce S, Uzun L, Rad AY, Unal S, Say R, Denizli A. 2012. Molecular imprinting based composite cryogel membranes for purification of anti-hepatitis B surface antibody by fast protein liquid chromatography. J Chromatogr B. 889:95–102.
- Asliyuce S, Uzun L, Say R, Denizli A. 2013. Immunoglobulin G recognition with Fab fragments imprinted monolithic cryogels: evaluation of the effects of metal-ion assisted-coordination of template molecule. React Funct Polym. 73:813–820.
- Aslıyüce S,Bereli N, Uzun L, Onur MA, Say R, Denizli A. 2010. Ion-imprinted supermacroporous cryogel, for in vitro removal of iron out of human plasma with beta thalassemia. Sep Purif Technol. 73:243–249.
- Bacha AB, Gargouri Y, Ali YB, Miled N, Reinbolt J, Mejdoub H. 2005. Purification and biochemical characterization of ostrich pancreatic lipase. Enzyme Microb Technol. 37:309–317.
- Baydemir G, Andaç M, Derazshamshir A, Uygun DA, Özçalışkan E, Akgöl S, Denizli A. 2013. Synthesis and characterization of amino acid containing Cu(II) chelated nanoparticles for lysozyme adsorption. Mater Sci Eng C Mater Biol Appl. 33:532–536.
- Berillo D, Mattiasson B, Galaev IY, Kirsebom H. 2012. Formation of macroporous self-assembled hydrogels through cryogelation of Fmoc-Phe-Phe. J Colloid Interface Sci. 368:226–230.
- Björkling F, Godtfredsen SE, Kirk O. 1991. The future impact of industrial lipases. Trends Biotechnol. 9:360–363.
- Brabcová J,Blažek J, Janská L, Krečmerová M, Zarevúcka M. 2012. Lipases as tools in the synthesis of prodrugs from racemic 9-(2,3-dihydroxypropyl)adenine. Molecules. 17:13813–13824.
- Bradford MM. 1976. A rapid and sensitive method for the quantitation of microgram quantities of protein utilizing the principle of protein-dye binding. Anal Biochem. 72:248–254.
- Brockerhoff H, Hoyle RJ, Hwang PC. 1970. Digestive enzymes of the American lobster (Homarus americanus). J Fish Res Board Can. 27:1357–1370.
- Chen WY, Liu ZC, Lin PH, Fang CI, Yamamoto S. 2007. The hydrophobic interactions of the ion-exchanger resin ligands with proteins at high salt concentrations by adsorption isotherms and isothermal titration calorimetry. Sep Purif Technol. 54:212–219.
- Dunn PJ. 2012. The importance of green chemistry in process research and development. Chem Soc Rev. 41:1452–1461.
- Fendri A, Frikha F, Mosbah H, Miled N, Zouari N, Ben Bacha A, et al. 2006. Biochemical characterization, cloning, and molecular modelling of chicken pancreatic lipase. Arch Biochem Biophys. 451:149–159.
- Flexer V, Durand F, Tsujimura S, Mano N. 2011. Efficient direct electron transfer of PQQ-glucose dehydrogenase on carbon cryogel electrodes at neutral pH. Anal Chem. 83:5721–5727.
- Furutani T, Su R, Ooshima H, Kato J. 1995. Simple screening method for lipase for transesterification in organic solvent. Enzyme Microb Technol. 17:1067–1072.
- Garner CW, Smith LC. 1970. A convenient purification of porcine pancreatic lipase free of proteolytic activity. Arch Biochem Biophys. 140:503–507.
- Genest PW, Field TG, Vasudevan PT, Palekar AA. 1998. Continuous purification of porcine lipase by rotating annular size-exclusion chromatography. J Appl Biochem Biotechnol. 73:215–230.
- Gupta A, Sarkar J, Kumar A. 2013. High throughput analysis and capture of benzo[a]pyrene using supermacroporous poly(4-vinyl pyridine-co-divinyl benzene) cryogel matrix. J Chromatogr A. 1278:16–21.
- Brockerhoff H, Jensen RG. 1974. Lipolytic Enzymes. New York: Academic Press.
- Hajizadeh S, Xu C, Kirsebom H, Ye L, Mattiasson B. 2013. Cryogelation of molecularly imprinted nanoparticles: a macroporous structure as affinity chromatography column for removal of β-blockers from complex samples. J Chromatogr A. 1274:6–12.
- Hedström M, Plieva F, Galaev IY, Mattiasson B. 2008. Monolithic macroporous albumin/chitosan cryogel structure: a new matrix for enzyme immobilization. Anal Bioanal Chem. 390:907–912.
- Hwang Y, Zhang C, Varghese S. 2010. Poly (ethylene glycol) cryogels as potential cell scaffolds: effect of polymerization conditions on cryogel microstructure and properties. J Mater Chem. 20:345–351.
- Jaeger KE, Ransac S, Dijkstra BW, Colson C, van Heuvel M, Misset O. 1994. Bacterial lipases. FEMS Microbiol Rev. 15:29–63.
- Jaeger K-E, Eggert T. 2002. Lipases for biotechnology. Curr Opin Biotechnol. 13:390–397.
- Kapoor M, Gupta MN. 2012. Lipase promiscuity and its biochemical applications. Process Biochem. 47:555–569.
- Karadzic I, Masui A, Zivkovic LI, Fujiwara N. 2006. Purification and characterization of an alkaline lipase from Pseudomonas aeruginosa isolated from putrid mineral cutting oil as component of metalworking fluid. J Biosci Bioeng. 102:82–89.
- Kirsebom H, Topgaard D, Galaev IY, Mattiasson B. 2010. Modulating the porosity of cryogels by influencing the nonfrozen liquid phase through the addition of inert solutes. Langmuir. 26:16129–16133.
- Kueseng P, Thammakhet C, Thavarungkul P, Kanatharana P. 2010. Multiwalled carbon nanotubes/cryogel composite, a new sorbent for determination of trace polycyclic aromatic hydrocarbons. Microchem J. 96:317–323.
- Mejdoub H, Reinbolt J, Gargouri Y. 1994. Dromedary pancreatic lipase: purification and structural properties. Biochimica et Biophysica Acta. 1213:119–126.
- Patil KJ, Chopda MZ, Mahajan RT. 2011. Lipase biodiversity. Indian J Sci Technol. 4:971–982.
- Rovery M, Boudouard M, Bianchetta J. 1978. An improved large scale procedure for the purification of porcine pancreatic lipase. Biochimica et Biophysica Acta. 525:373–379.
- Schmidt M, Bornscheuer UT. 2005. High-throughput assays for lipases and esterases. Biomol Eng. 22:51–56.
- Segura RL, Betancor L, Palomo JM, Hidalgo A, Fernández-Lorente G, Terreni M, et al. 2006. Purification and identification of different lipases contained in PPL commercial extracts: a minor contaminant is the main responsible of most esterasic activity. Enzyme Microb Technol. 39:817–823.
- Solano DM, Hoyos P, Hernáiz MJ, Alcántara AR, Sánchez-Montero JM. 2012. Industrial biotransformations in the synthesis of building blocks leading to enantiopure drugs. Bioresour Technol. 115:196–207.
- Tsai YC, Huang JD, Chiu CC. 2007. Amperometric ethanol biosensor based on poly(vinyl alcohol)-multiwalled carbon nanotube-alcohol dehydrogenase biocomposite. Biosens Bioelectron. 22:3051–3056.
- Tsujimura S, Nishina A, Hamano Y, Kano K, Shiraishi S. 2010. Electrochemical reaction of fructose dehydrogenase on carbon cryogel electrodes with controlled pore sizes. Electrochem Commun. 12:446–449.
- Uzun L, Armutcu C, Biçen Ö, Ersöz A, Say R, Denizli A. 2013. Simultaneous depletion of immunoglobulin G and albumin from human plasma using novel monolithic cryogel columns. Colloids Surf B Biointerfaces. 112:1–8.
- Verma N, Thakur S, Bhatt AK. 2012. Microbial lipases: industrial applications and properties (a review). Int Res J Biol Sci. 1:88–92.
- World Enzymes Market. 2009. “World Enzymes.” Available from: http://www.reportlinker.com/p0148002/World-Enzymes-Market.html.
- Yao K, Yun J, Shen S, Wang L, He X, Yu X. 2006. Characterization of a novel continuous supermacroporous monolithic cryogel embedded with nanoparticles for protein chromatography. J Chromatogr A. 1109:103–110.
- Yılmaz F, Bereli N, Yavuz H, Denizli A. 2009. Supermacroporous hydrophobic affinity cryogels for protein chromatography. Biochem Eng J. 43:272–279.
- Yun J, Jespersen GR, Kirsebom H, Gustavsson PE, Mattiasson B, Galaev IY. 2011. An improved capillary model for describing the microstructure characteristics, fluid hydrodynamics and breakthrough performance of proteins in cryogel beds. J Chromatogr A. 1218:5487–5497.