Abstract
Post cryopreservation viability of human embryonic kidney (HEK) cells under two-dimensional (2D) and three-dimensional (3D) culture conditions was studied using trehalose as the sole cryoprotective agent. An L9 (34) Taguchi design was used to optimize the cryoprotection cocktail seeding process prior to slow-freezing with the specific aim of maximizing cell viability measured 7 days post thaw, using the combinatorial cell viability and in-vitro cytotoxicity WST assay. At low (200 mM) and medium (800 mM) levels of trehalose concentration, encapsulation in alginate offered a greater protection to cryopreservation. However, at the highest trehalose concentration (1200 mM) and in the absence of the pre-incubation step, there was no statistical difference at the 95% CI (p = 0.0212) between the viability of the HEK cells under 2D and 3D culture conditions estimated to be 17.9 ± 4.6% and 14.0 ± 3.6%, respectively. A parallel comparison between cryoprotective agents conducted at the optimal levels of the L9 study, using trehalose, dimethylsulfoxide and glycerol in alginate microcapsules yielded a viability of 36.0 ± 7.4% for trehalose, in average 75% higher than the results associated with the other two cell membrane-permeating compounds. In summary, the effectiveness of trehalose has been demonstrated by the fact that 3D cell cultures can readily be equilibrated with trehalose before cryopreservation, thus mitigating the cytotoxic effects of glycerol and dimethylsulfoxide.
Introduction
Advantages of three-dimensional (3D) vs. two-dimensional (2D) cultures in terms of promoting mammalian cell survival and proliferation as well as karyotypic stability, homogeneity, scalability and reproducibility have been examined in detail by many researchers (Chang Citation2013, Kinney et al. Citation2014). A sub-component of process reproducibility is maintaining quiescence achieved by lyopreservation and/or cryopreservation. In the quiescent state, cells enter an amorphous/glassy phase characterized by low molecular mobility and activity to arrest biophysical and biochemical cellular activities. Successful preservation is governed by complex interactions between the type and concentration of cryo/lyoprotectant cocktails, kinetics of liquid water removal enhanced by 3D conformation, as well as cell–cell and cell–matrix interactions and specific properties of the individual IC cell lines (He Citation2011, Martín-Ibáñez et al. Citation2012, Serra et al. Citation2011, Wikström et al. Citation2012, Zhang et al. Citation2010). The critical variables leading to cryo-injury, use of cryoprotective agents (CPAs) and biophysics of mammalian cell cryopreservation for optimal recovery rates based on modeling of cell dehydration and intracellular ice formation have been extensively reviewed by He (Citation2011) and Martín-Ibáñez et al. (2012). Recent advances in 3D microencapsulation technology (e.g. using alginate capsules <100 microns) have enhanced the success rates for cryopreservation of mammalian cells across a multitude of freezing protocols, thus enabling the use of lower CPA concentrations (He Citation2011, Huang et al. Citation2015, Zhang et al. Citation2010).
The use of the anhydrobiotic compound trehalose (α,α-trehalose, α-d-glucopyranosyl-α-d-glucopyranoside) has been documented as both a cryo/lyoprotective agent with or without other CPAs for a wide variety of therapeutic applications ranging from blood banks to reproductive technology (Eroglu et al. Citation2000, Lee et al. Citation2013, Limaye and Kale Citation2001). The most re-occurring postulated preservation mechanisms summarized by Martín-Ibáñez et al. (Citation2012) are: (1) trehalose forms hydrogen bonds with biomolecules (similar to water) and preserve their functional conformations during water deficit (Lawson et al. Citation2011), and (2) trehalose acts as a non-permeating molecule to form a stable glassy matrix with low molecular mobility that suspends any intracellular degradative biochemical processes in response to water loss by inhibiting the resultant lipid phase transition (Clegg et al. Citation1982, Crowe Citation1971). There is also evidence in support of trehalose acting similar to a permeating CPA as it has been suggested by studies using various strategies to increase the intracellular concentration of trehalose, including the overexpression of Agt1, a specialized disaccharide transporter in Saccharomyces cerevisae (Tapia et al. Citation2015), introduction of trehalose using osmotic shock as well as heat shock (Puhlev et al. Citation2001), transfection using a genetically engineered mutant of the pore-forming α-hemolysin from Staphylococcus aureus (Acker et al. Citation2002) and nanoparticle-mediated intracellular delivery that higher intracellular concentrations of trehalose is often coupled with enhanced post-dessication viability (Rao et al. Citation2015). Additional clarification for the role of trehalose as an intracellular CPA may be obtained through the use of the acetylated trehalose with the proven ability to permeate through the mammalian cell membrane (Abazari et al. Citation2015).
In this study, the suitability of trehalose for the cryopreservation of human embryonic kidney (HEK) cells has been explored. HEK cells and cell derivatives are routinely used in: (1) various research and bioprocess activities including viral packaging and drug discovery (Dietmair et al. Citation2012), (2) studies where the effects of micro-environmental cues on cell proliferation, transfection and migration are explored (Pebworth et al. Citation2014), (3) in biopharmaceutical production (Bandaranayake and Almo Citation2014) and (4) in desiccation research for genome mapping against anhydrobiotic organisms to elucidate common protection mechanisms against dehydration at the genetic level (Leprince and Buitink Citation2010). HEK cells have been cryopreserved using slow freezing in DMSO-based cocktails recommended for cryopreservation (Chaytor et al. Citation2012, Kleman Citation2008). DMSO is highly toxic under physiological conditions characterized by multi-step solvent removal associated with significant yield losses (Rao et al. Citation2015), as traces of the compound have been shown to lead to cell damage and differentiation (Chetty et al. Citation2013). The specific aim of this research effort is to assess the feasibility of trehalose as a “sole” non-cytotoxic cryoprotectant for the preservation of 3D cultures of HEK cells using conventional slow-freezing. The significance of this capability is twofold: (1) 3D cell cultures can readily be equilibrated with trehalose before cryopreservation, therefore eliminating the need for any transmembrane manipulations (e.g. microinjection, electroporation, genetic engineering, or derivatization, and (2) if trehalose is non-cytotoxic, it eliminates the need for an additional wash step during the thawing procedure.
Materials and methods
Materials
Human embryonic kidney (HEK) cells (ATCC, CRL-1573) were obtained from ATCC (Manassas, VA). Dulbecco's modified Eagle medium (DMEM, Life Technologies 11965) and fetal bovine serum (FBS, Life Technologies 16000) were procured from Life Technologies (Carlsbad, CA). Penicillin-streptomycin (Cellgro, 30–002-CI) and Trypsin-EDTA (Cellgro 25–053-CI) were manufactured by Cellgro (Manassas, VA). All the reagents required for the microencapsulation of HEK cells, including medium viscosity alginate (Sigma A2033 ≥ 2000 cP at 2% w/v) and D-(+)-Trehalose dihydrate (Sigma T9531), as well as reagent grade salts and solvents were purchased from Sigma Aldrich (St-Louis, MO).
Methods
Cell culture and microencapsulation
HEK were maintained in DMEM supplemented with 15% FBS and 1% penicillin-streptomycin at 37 °C in a 5% CO2 humidified environment. The cells were cultured in 100 mm tissue culture polystyrene (TCPS) dishes to 60–80% confluency and sub-cultured at a 1:4 ratio using 0.25% trypsin. For encapsulation in alginate, cell suspensions in DMEM were mixed with a sterilized 3% (w/v) solution of alginate in DMEM to yield a final cell concentration of 106 cells/mL The cell mixture was then placed into a syringe fitted with an 18-gauge needle and slowly dropped into a 1.5% w/v CaCl2 solution on a stir-plate to initiate gelation (Wong and Chang Citation1986). After allowing the alginate gel beads to crosslink for about 30 min, the beads were first washed with 0.9% NaCl followed by DMEM. The alginate-HEK beads were cultured under conditions described above for at least 24 h to acclimate to the 3D hydrogel environment prior to further testing.
Cell proliferation and toxicity assays for optimizing the concentration of the cryoprotectants
Cell proliferation and toxic responses to the cryoprotectants were determined using the WST-8 cell proliferation assay (ATCC, Manassas, VA) as per the manufacturer's instructions. For proliferation testing, HEK cells were first either seeded into 96-well flat bottom plates, or encapsulated in alginate, and allowed to proliferate for up to a week. WST-8 reagent was added to each well and the cells were incubated in a 37 °C incubator for 4 h before the absorbance was measured at 450 nm using a Tecan Infinite 200 PRO spectrophotometer (Durham, NC). The cell number on day 7 was then determined using the WST absorption calibration curves generated for HEK cells (presented in ), and reported relative to the value at 24 h after encapsulation in alginate. For toxicity testing, HEK cells were seeded into 96-well flat-bottom plates at a cell density of 12,000–13,000 cells/well and allowed to proliferate for 24 h prior to a subsequent 24 h exposure to various concentrations of trehalose dissolved in DMEM, followed by the addition of WST reagent and absorbance measurements. Percent viability was determined by normalizing the WST readings for cells exposed to trehalose to those for control cells maintained DMEM without trehalose. The measurements were performed in triplicate and MS Excel (Microsoft, WA) was used to fit an empirical model to the data for determining the half maximal inhibitory concentration (IC50).
Figure 1. Calibration curve for cell viability as measured using the WST assay for HEK cells cultured in 2D and 3D conditions prior to cryopreservation – representative viability for (i) cells encapsulated in alginate incorporating trehalose: Alginate + Trehalose in DMEM (diamonds); (ii) cells in 2D culture conditions after pretreatment and second incubation in trehalose: Trehalose + Trehalose in DMEM (squares); (iii) cells encapsulated in alginate not incorporating trehalose: Alginate + DMEM (triangles); and (iv) cells in 2D culture conditions after pretreatment trehalose: Trehalose + DMEM (circles).
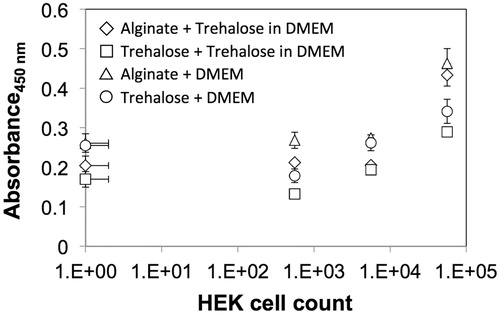
Cryoprotection process
The entire process flow for the cryopreservation experiments, a sub-component of which will be described in the cyroprotection optimization section, is illustrated in . HEK cells were first microencapsulated in 3% (w/v) autoclaved alginate containing 0–200 mM trehalose. The microencapsulated cells were then incubated in 0–150 mM trehalose for 24 h. Following trehalose pre-incubation, cells were placed in cryovials (Nunc A/S, Roskilde, Denmark) with DMEM mixed with 200–1200 mM trehalose as the cryoprotectant. After the cryoprotectant equilibration step, vials were placed in an ice bath for 0–60 min followed by freezing in an isopropanol-based freezing system (“Mr. Frosty”, Nalgene, NY) that provides a 1 °C/min cooling rate in the −80 °C freezer. Non-encapsulated cells were treated the similar way, except that they were pelleted using centrifugation before placing in cryovials prior to the slow-freezing process. After 24 h, cryovials were removed from the freezer and thawed in a 37 °C water bath followed by a single wash with DMEM. Cells were then placed in 96-well flat-bottom plate; either 3 microcapsules per well for encapsulated cells or 12,000–13,000 cells/well for the non-encapsulated cells. All cell preparations were cultured for 7 days before being tested for viability.
Cryoprotectant seeding and formulation optimization
A modified L9 Taguchi design orthogonal array was used for robust screening and optimization of operating parameters (Roy Citation2001). Experiments were conducted in triplicate to maximize the cell viability using the “Larger-the-Better” optimization goal. The formulae needed to determine the optimized level of parameters based on the sensitivity (Sm), signal to noise ratios (S/N), difference (D), and rank (R) using the nominal-the-best-optimization are given in EquationEquations 1–4.
Difference, D is given by:
(1)
where i corresponds to the level, j corresponds to the factor, and L is the total number of levels.
Rank, R is given by:
(2)
Signal-to-noise ratio (dB) for the jth experiment, (S/N)j is given by:
(3)
where n is the total number of replicates per experiment, and Ymj is the measured viability for the mth trial of the jth experiment.
Sensitivity (dB) for the jth experiment (Sm)j is given by:
(4)
The design matrix was conducted according to the layout in with 4 factors and 3 levels: cryoprotectant concentration (Tre, mM), cryoprotectant uptake time (Uptime, min), pre-treatment trehalose concentration (PretreatC, mM), membrane material composition (Material, attribute scale). The experimental range for the pre-incubation time (PretreatC) was based on the results of the WST assay that was used to determine the HEK toxic responses to the cryoprotectant. The levels of the other 3 variables were chosen to minimize interactions between the beneficial effects of microencapsulation during cryopreservation and the trehalose content of the cryo-formulation. Level 1 of the cryoprotectant uptake time, set to zero, corresponds to an experimental duration of 15 s. Following independent calculations and response plot generation for the sensitivity and signal-to-noise ratio, results were validated using the software Minitab® 16 (State College, PA). Three confirmation runs were performed for the optimal results.
Table 1. L9 Taguchi experimental design.
Results
Effect of cell culture condition and trehalose on HEK cell viability
To compare the effects of 2D vs. 3D culture, HEK cells in alginate gel beads were microencapsulated using the modified drop method as described in the “Methods” section and characterized using standard microscopy techniques and cell viability assays. shows alginate-HEK microcapsules with an average diameter of 2.5 ± 0.5 mm. As seen in , HEK cells encapsulated in alginate show a rounded morphology, which is typical of cells encapsulated in unmodified alginate that do not present moieties for cell adhesion. To compare the viability of HEK cells encapsulated in the alginate beads to those cultured on tissue culture polystyrene (TCPS), a mitochondrial colorimetric assay was employed that revealed >75% cell survival for both 2D and 3D cultures after one week (). Next, the effect of trehalose on HEK viability was assessed. shows the fitted dose response curve for the HEK cells exposed to varying concentrations of trehalose for 24 h based on which the IC50 of trehalose was calculated to be 240 mM or 9.1% (w/v).
Figure 3. Visual characterization of alginate microcapsules. (a) Representative pictures showing alginate-HEK microcapsules with an average diameter of 2.5 ± 0.5 mm. (b) Representative microscope images showing HEK cells encapsulated in alginate with a rounded morphology. White arrows show cells in focus.
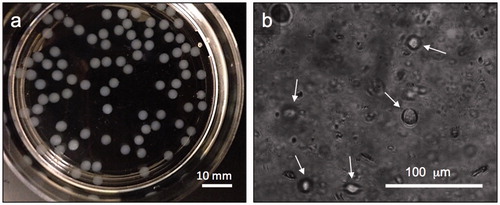
Cryoprotectant seeding and formulation optimization
An L9 Taguchi array was used for the cryoprotection process optimization with the specific aim of maximizing cell viability. The choice of the specific parameters and their respective operating range was based on the results of a 2D cytotoxicity screening presented in and the performance of various membrane formulations for cell microencapsulation and the 2D configuration. The normalized cell viability data and (S/N) plots are presented in and , and the selected levels of operations per response are summarized in . Based on the mathematical optimization results, the following levels for each factor were selected for the confirmation runs and subsequent experimentation: Tre = 1200 mM, Uptime = 60 min, PretreatC = 150 mM, and Material = alginate. At low (200 mM) and medium (800 mM) levels of cryoprotectant concentrations, encapsulation in alginate offered a greater protection to cryopreservation. However, interestingly at the highest trehalose concentration (1200 mM) and in the absence of the pre-incubation step, there was no statistical difference at the 95% CI (P = 0.0212) between the viability of the HEK cells under 2D culture conditions (Run 8) and the cells in the 3D culture conditions (Run 1), estimated to be 17.9 ± 4.6% and 14.0 ± 3.6%, respectively. Thus, experimental conditions described by Run 8 are recommended for HEK cryopreservation under 2D culture conditions.
Figure 5. Viability of cells under the optimization conditions as described by the L9 Taguchi design measured on Day 7 post-thawing, normalized against non-cryopreserved alginate-encapsulated HEK cells incubated in DMEM. Runs 1 and 8 (denoted by asterisks) represent cells in 3D and 2D culture conditions, respectively, at the highest trehalose concentration (1200 mM) and in the absence of the pre-incubation step.
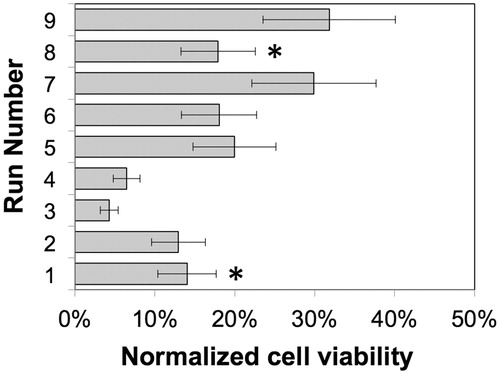
Figure 6. Signal-to-Noise (S/N) plots for the Taguchi L9 optimization by factor: cryoprotectant concentration (Tre), cryoprotectant uptake time (Uptime), pre-treatment trehalose concentration (PretreatC), and membrane material composition (Material).
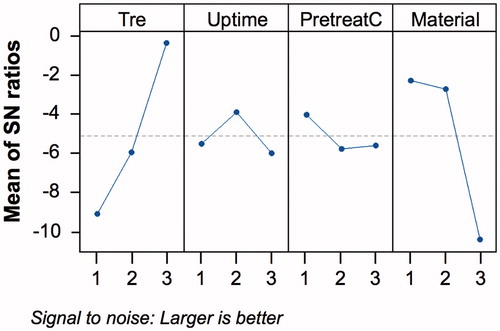
Table 2: Summary of L9 Taguchi analysis of cryoprotectant seeding using the Larger-the-Better optimization type.
Comparison with other cryoprotectants
HEK cells encapsulated in alginate were also frozen using two other cryoprotectants, glycerol and DMSO for comparison. The conditions used for the comparison were similar to the L9 confirmation runs except for the choice of CPA in the second equilibration step (Variable 1, Tre). The first equilibration step (Variable 3, PretreatC) was set at the optimal level of 150 mM trehalose, the equilibration time for CPA permeation was set to 60 min and the cells were cultured in 3D using alginate microencapsulation (variable 4, Material). The freezing and thawing protocols were identical. After the cells were thawed, they were maintained under the optimal HEK culture conditions described in the Methods section (i.e. in DMEM supplemented with 15% FBS and 1% penicillin-streptomycin at 37 °C in a 5% CO2 humidified environment).
The efficiency of the different cryoprotectants on preserving HEK cells during the freezing process are compared in . The data was normalized to alginate encapsulated HEK cells that were not exposed to any cryoprotectants or cryopreserved, but maintained under the optimal HEK culture conditions for 7 days. The cell viability data for alginate-encapsualted HEK cells under conditions described in Run #1 of the Taguchi array (i.e. no pretreatment, 15 s incubation with 200 mM trehalose) is also shown for comparison. As shown in , the viability of cells following treatment with trehalose is significantly higher relative to those treated with DMSO or glycerol. Specifically, treatment of the 3D matrix with trehalose at 4 °C (variable 1/Tre) results in doubling viability as compared to the frozen microcapsules in the absence of any cryoprotectant characterized by a viability of 15.9 ± 6.3%. Moreover, comparison with cells exposed to conditions in Run #1 shows that the cell viability is negativelty impacted by the exposure to glycerol and DMSO, but not trehalose, indicating that glycerol and DMSO exhibit cytotoxic effects to HEK cells.
Figure 7. Comparative effect of cryoprotectants on the 7 day post-thaw cell viability of HEK cells microencapsulated in alginate. Alginate encapsulated cells were frozen with either 45% w/v trehalose, 10% w/v glycerol, or 10% w/v DMSO in DMEM, and compared to the post-thaw viability of microencapsulated cells minimally exposed to any CPA agent (Run #1). Cell viability for all the conditions were normalized against non-cryopreserved alginate-encapsulated HEK cells incubated in DMEM.
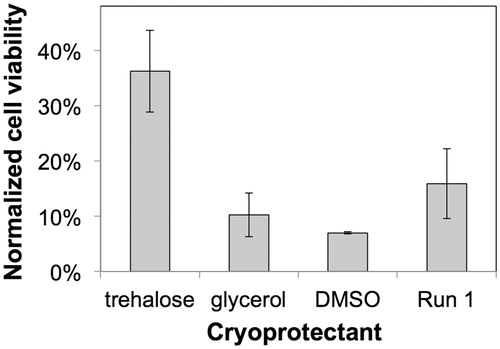
Discussion
There have been numerous publications describing a diverse array of strategies for cryoprotection and cryopreservation that have been elaborated upon and referenced throughout this research effort. However, very few or no publications have addressed the combinatorial effects of the 3D cell culture environment and trehalose on HEK cell viability and proliferation after cryopreservation. Thus, the postulated mechanisms proposed in the next three sections regarding trehalose cytotoxicity, membrane permeability and the protective effect of 3D on cells in the cryopreserved state are based on the state of the art in documented behaviour of mammalian cell lines in general.
Effect of cell culture condition and trehalose on HEK cell viability
The upper limit for trehalose concentration in this study was set to 150 mM (or 5.7% w/v), ca. 50% of the IC50 value obtained using cytotoxcity assays performed using standard 2D culture methods. Previous research has suggested that the mammalian cell membrane is impermeable to trehalose (He Citation2011, Rao et al. Citation2015); therefore, cytoxicity at higher doses of trehalose could be atributed to the hypertonicity of the media and water efflux resulting in cell shrinkage, distortion and preferential hydration (Huang and Tunnacliffe Citation2007). A wide variety of pre-incubation times have been documented for trehalose; for instance, Wikström et al. (Citation2012) reported an upper pre-incubation period of 12 days for the cryopreservation of microencapsulated human retinal pigment epithelial cells, while on the other extreme, the pre-incubation period was limited to 15 min for human corneal epithelium cells in 2D (Hovakimyan et al. Citation2012).
Cryoprotectant seeding and formulation optimization
The choice of alginate for 3D culture of HEK cells was motivated by strong evidence that alginate membranes are permeable to hydrophilic molecules characterized by Stokes radii of less than 3 nm (Mobed-Miremadi et al. Citation2014). Relevant to this study, alginate permeability to trehalose and small saccharides has been demonstrated by: (1) the enzymatic production of trehalose in a packed bed reactor containing recombinant E. coli encapsulated in alginate beads (Di Lernia et al. Citation2002) and (2) glucose equilibration studies for glucose diffusion modeling (Estapé et al. Citation1992) and optimization of parameters for encapsulation of pancreatic islet cells (Wong and Chang Citation1986). Assuming a conservative equilibration time of 300 s at 30 °C and that change in the viscosity of trehalose-DMEM medium is negligible as a function of temperature, an equilibration time of 274 s can be extrapolated at 4 °C using the Stokes–Einstein equation (Fournier Citation2011) for a membrane thickness of 5–10 μm (Mobed-Miremadi et al. Citation2014). Thereby, it is safe to assume that HEK cells would have been exposed up to 6 times the IC50 concentration (trehalose IC50 = 240 mM, ) at 1200 mM (45% w/v) under the optimized cryoprotection seeding conditions of 60 min at 4 °C. It could be hypothesized that the dehydration-driven apoptosis is reduced due to a decrease in Q10 temperature coefficient (Zotin Citation1990) and shorter exposure times as compared to the cytotoxicity study conducted at 37 °C for 24 h. This hypothesis can be extended to 2D (Run 8) and 3D (Runs 7 and 9) experimental runs.
At higher CPA concentrations, as indicated by the analyses of , alginate matrix–trehalose combination offers a higher protection to cells during cryopreservation whether incorporated in the 3D matrix or equilibrated across the alginate membrane (via preincubation). At neutral pH, liquid water penetrates into the chains of alginate to form hydrogen bridges through the –OH and –COO− groups, and fills up the space along the chains and/or the center of wide pores or voids (Chen et al. Citation2009). It could therefore be hypothesized that the hydrogen bonding of trehalose with alginate is the root cause of the additional stabilization during freezing in 3D.
Comparison with other cryoprotectants
The maximal yield of ca. 36% using the optimal conditions is not within the acceptable range for reproducibility and scale-up from laboratory R&D to commercial scale processes. Moreover, the reported yields in this study are significantly lower than those reported across various mammalian cell lines in the literature (Eroglu et al. Citation2000, He Citation2011, Lee et al. Citation2013, Limaye and Kale Citation2001, Martín-Ibáñez et al. Citation2012, Serra et al. Citation2011, Wikström et al. Citation2012, Zhang et al. Citation2010) specifically, 60% post-thawing efficiency has been reported for HEK cells using 5% DMSO dissolved in histidine–tryptophan–ketoglutarate medium (Chaytor et al. Citation2012), which is at least 70% higher than the values obtained for the optimal conditions in 2D using DMSO (Run 8). The latter can be explained by higher viability efficiencies immediately after thawing relative to those observed after 1–7 days culture of the thawed cells (Heng et al. Citation2006). As outlined in the “Methods” section, it is noteworthy to mention that the data presented in this study is representative of cell viability after 7 day of culture post-thaw. Another possible source of discrepancy is that the DMEM-based freezing and thawing media used in our protocol did not include FBS or other serum substitutes that have been shown to prevent ice recrystallization and thereby cell death during thawing (Varma et al. Citation2015, Verdanova et al. Citation2014). Finally, the losses due to washing steps post rehydration for the removal of cytotoxic non-permeating CPAs such as glycerol and DMSO are not taken into account in the final reported value as outlined by Rao et al. (Citation2015).
Meanwhile, 3D encapsulation of cells to preserve their viability during the freeze-thaw cycle opens up the possibility to further increase freezing efficiencies by varying the biophysical and biochemical properties of the scaffold. For instance, recent studies have demonstrated the individual as well as synergistic effects of matrix stiffness and cell–matrix interactions on cell responses to small molecule toxins (Pebworth et al. Citation2014). While it is not clear if the intracellular responses to freeze-thaw and other toxic insults are similar, it could be postulated that biophysical and biochemical properties of the 3D cell culture matrix may also have a significant effect on cell viability during the freeze-thaw process.
Conclusions
HEK cell viability optimization in traditional 2D and alginate-based 3D culture conditions was successfully conducted using an L9 Taguchi design. Extracellular trehalose whether incorporated directly into the 3D matrix or equilibrated across the alginate membrane, offers HEK cells higher protection against cryopreservation relative to 3D encapsulation alone during the slow-freezing process. Under optimal conditions consisting of microencapsulation in alginate, pre-incubation in 150 mM trehalose for 24 hr followed by a second equilibration step of 60 min in 1200 mM in the cryopreservation cocktail a viability of 36.0 ± 7.4% was obtained for trehalose. This post-cryopreservation yield is up to 75% higher than the value obtained for the optimized protocol in 3D conducted using non-permeating CPAs, DMSO and glycerol (designated as standard cryoprotective agents used for HEK cryopreservation in 2D) instead of trehalose. The list of parameters in the Taguchi design to optimize the combination of 3D cell culture matrix properties and CPAs will be expanded in future studies. Specifically, the inclusion of serum or serum substitutes to the freezing trehalose-DMEM cocktail and the optimization of post-thawing steps to assess the effect of ice recrystallization on cell viability will be explored. Moreover, the ability to store desiccated HEK cells at ambient temperature offers a tremendous economic and pragmatic advantage over cryopreservation in 2D and 3D. Therefore, future efforts will also include the evaluation of combining 3D culture and trehalose supplementation through direct incorporation and/or preincubation for lyoprotection.
Acknowledgements
The authors would like to acknowledge the Bannan School of Engineering for funding this research effort.
Disclosure statement
The authors report no declarations of interest. The authors alone are responsible for the content and writing of the paper.
References
- Abazari A, Meimetis LG, Budin G, Bale SS, Weissleder R, Toner M. 2015. Engineered trehalose permeable to mammalian cells. PLoS One. 10:e0130323.
- Acker JP, Fowler A, Lauman B, Cheley S, Toner M. 2002. Survival of desiccated mammalian cells: Beneficial effects of isotonic media. Cell Preserv Technol. 1:129–140.
- Bandaranayake AD, Almo SC. 2014. Recent advances in mammalian protein production. FEBS Lett. 588:253–260.
- Chang TMS. 2013. Selected Topics in Nanomedicine. Singapore: World Scientific Publishing.
- Chen H, Ouyang W, Martoni C, Prakash S. 2009. Genipin cross-linked polymeric alginate-chitosan microcapsules for oral delivery: in-vitro analysis. Int J Polym Sci. 2009: 617184.
- Clegg JS, Seitz P, Seitz W, Hazlewood CF. 1982. Cellular responses to extreme water loss: the water-replacement hypothesis. Cryobiology. 19:306–316.
- Crowe JH. 1971. Anhydrobiosis: an unsolved problem. Am Naturalist. 105:563–573.
- Chaytor JL, Tokarew JM, Wu LK, Leclère M, Tam RY, Capicciotti CJ, et al. 2012. Inhibiting ice recrystallization and optimization of cell viability after cryopreservation. Glycobiology. 22:123–133.
- Chetty S, Pagliuca FW, Honore C, Kweudjeu A, Rezania A, Melton DA. 2013. A simple tool to improve pluripotent stem cell differentiation. Nat Methods. 10:553–556.
- Di Lernia I, Schiraldi C, Generoso M, De Rosa M. 2002. Trehalose production at high temperature exploiting an immobilized cell bioreactor. Extremophiles. 6:341–347.
- Dietmair S, Hodson MP, Quek LE, Timmins NE, Gray P, Nielsen LK. 2012. A multi-omics analysis of recombinant protein production in Hek293 cells. PLoS One. 7:e43394.
- Eroglu A, Russo MJ, Bieganski R, Fowler A, Cheley S, Bayley H, Toner M. 2000. Intracellular trehalose improves the survival of cryopreserved mammalian cells. Nature Biotechnol. 18:163–167.
- Estapé D, Gòdia F, Solà C. 1992. Determination of glucose and ethanol effective diffusion coefficients in Ca-alginate gel. Enzyme Microb Technol. 14:396–401.
- Fournier RL. 2011. Basic Transport Phenomena in Biomedical Engineering, 3rd ed. Philadelphia: Taylor & Francis.
- He X. 2011. Preservation of embryonic stem cells. In: Atwood C, Ed. Methodological Advances in the Culture, Manipulation and Utilization of Embryonic Stem Cells for Basic and Practical Applications. Rijeka, Croatia: Intech Europe. [cited 2016 Mar 7]. Available from: http://www.intechopen.com/books/methodologicaladvances-in-the-culture-manipulation-and-utilization-of-embryonic-stem-cells-for-basic-and-practicalapplications/preservation-of-embryonic-stem-cells for free access.
- Heng BC, Ye CP, Liu H, Toh WS, Rufaihah AJ, Yang Z, et al. 2006. Loss of viability during freeze-thaw of intact and adherent human embryonic stem cells with conventional slow-cooling protocols is predominantly due to apoptosis rather than cellular necrosis. J Biomed Sci. 13:433–445.
- Hovakimyan M, Ramoth T, Löbler M, Schmitz KP, Witt M, Guthoff R, Stachs O. 2012. Evaluation of protective effects of trehalose on desiccation of epithelial cells in three dimensional reconstructed human corneal epithelium. Curr Eye Res. 37:982–989.
- Huang H, Choi JK, Rao W, Zhao S, Agarwal P, Zhao G, He X. 2015. Alginate hydrogel microencapsulation inhibits devitrification and enables large-volume low-CPA cell vitrification. Advanced Funct Mater. 25:6839–6850.
- Huang Z, Tunnacliffe A. 2007. Desiccation response of mammalian cells: anhydrosignaling. Meth Enzymol. 428:269–277.
- Karlsson JO. 2002. Cryopreservation: freezing and vitrification. Science. 296:655–656.
- Kinney MA, Hookway TA, Wang Y, McDevitt TC. 2014. Engineering three-dimensional stem cell morphogenesis for the development of tissue models and scalable regenerative therapeutics. Ann Biomed Eng. 42:352–367.
- Kleman MI, Oellers K, Lullau E. 2008. Optimal conditions for freezing CHO-S and HEK293-EBNA cell lines: influence of Me2SO, freeze density, and PEI-mediated transfection on revitalization and growth of cells, and expression of recombinant protein. Biotechnol Bioeng. 100:911–922.
- Leprince O, Buitink J. 2010. Desiccation tolerance: from genomics to the field. Plant Sci. 179:554–564.
- Lawson A, Ahmad H, Sambanis A. 2011. Cytotoxicity effects of cryoprotectants as single-component and cocktail vitrification solutions. Cryobiology. 62:115–122.
- Lee YA, Kim YH, Kim BJ, Kim BG, Kim KJ, Auh JH, Schmidt JA, Ryu BY. 2013. Cryopreservation in trehalose preserves functional capacity of murine spermatogonial stem cells. PLoS One. 8:e54889.
- Limaye LS, Kale VP. 2001. Cryopreservation of human hematopoietic cells with membrane stabilizers and bioantioxidants as additives in the conventional freezing medium. J Hematother Stem Cell Res. 10:709–718.
- Martín-Ibáñez R, Hovatta O, Canals JM. 2012. Cryopreservation of human pluripotent stem cells: are we going in the right direction? In: Katkov I, Ed. Current Frontiers in Cryobiology. Rijeka, Croatia: Intech Europe. [cited 2016 Mar 7]. Available from: http://www.intechopen.com/books/current-frontiers-incryobiology/cryopreservation-of-human-pluripotent-stem-cells-are-we-going-in-the-right-direction- for free access.
- Mobed-Miremadi M, Djomehri S, Keralapura M, McNeil M. 2014. Fickian-based empirical approach for diffusivity determination in hollow alginate-based microfibers using 2D fluorescence microscopy and comparison with theoretical predictions. Materials. 7:7670–7688.
- Pebworth MP, Cismas SA, Asuri P. 2014. A novel 2.5D culture platform to investigate the role of stiffness gradients on adhesion-independent cell migration. PLoS One. 9:e110453.
- Puhlev I, Guo N, Brown DR, Levine F. 2001. Desiccation tolerance in human cells. Cryobiology. 42:207–217.
- Rao W, Huang H, Wang H, Zhao S, Dumbleton J, Zhao G, He X. 2015. Nanoparticle-mediated intracellular delivery enables cryopreservation of human adipose-derived stem cells using trehalose as the sole cryoprotectant. ACS Appl Mater Interfaces. 7:5017–5028.
- Roy RK. 2001. Design of Experiments Using the Taguchi Approach: 16 Steps to Product and Process Improvement. New York City: John Wiley & Sons.
- Serra M, Correia C, Malpique R, Brito C, Jensen J, Bjorquist P, et al. 2011. Microencapsulation technology: a powerful tool for integrating expansion and cryopreservation of human embryonic stem cells. PLoS One. 6:e23212.
- Tapia H, Young L, Fox D, Bertozzi CR, Koshland D. 2015. Increasing intracellular trehalose is sufficient to confer desiccation tolerance to Saccharomyces cerevisiae. Proc Natl Acad Sci USA. 112:6122–6127.
- Verdanova M, Pytlik R, Kalbacova MH. 2014. Evaluation of sericin as a fetal bovine serum-replacing cryoprotectant during freezing of human mesenchymal stromal cells and human osteoblast-like cells. Biopreserv Biobank. 12:99–105.
- Varma VP, Devi L, Venna NK, Murthy CLN, Idris MM, Goel S. 2015. Ocular fluid as a replacement for serum in cell cryopreservation media. PLoS One. 10:e0131291.
- Wikström J, Elomaa M, Nevala L, Räikkönen J, Heljo P, Urtti A, Yliperttula M. 2012. Viability of freeze dried microencapsulated human retinal pigment epithelial cells. Eur J Pharm Sci. 47:520–526.
- Wong H, Chang TMS. 1986. Bioartificial liver: implanted artificial cells microencapsulated living hepatocytes increases survival of liver failure rats. Int J Artif Organs. 9:335–336.
- Zhang W, Yang G, Zhang a, Xu Lx, He X. 2010. Preferential vitrification of water in small alginate microcapsules significantly augments cell cryopreservation by vitrification. Biomed Microdevices. 12:89–96.
- Zotin AI. 1990. Thermodynamic Bases of Biological Processes: Physiological Reactions and Adaptations. Berlin, New York: Walter de Gruyter.