Abstract
Super-paramagnetic iron oxide nanoparticles (SPIONs) have been widely explored as novel magnetic resonance imaging (MRI) contrast agents because of a combination of favorable super-paramagnetic properties, biodegradability, and surface properties. Among the numerous MNPs under examination, SPIONs have involved considerable interest due to their outstanding magnetic properties, biocompatibility, and biodegradability. This review debates the ABCs of MR imaging, reports and informs the state of the art of magnetic nanoparticles in cancer dealing the source of SPIONs’ exclusive magnetic properties, recent progresses in MRI acquisition methods for detection of SPIONs.
Introduction
Nanotechnology has given researchers new tools for the advance of progressive materials for the detection and diagnosis of ailment. Magnetic nanoparticles (MNPs) have involved much attention because of their exclusive physical properties, magnetic susceptibility, biocompatibility, stability, and many more relevant features. In addition, MNPs are easily controlled by an exterior magnetic field application, which offer the releasing of the anticancer agent at an exact rate and at a specific site, overcoming the problems of conformist techniques for diagnosis and therapy.
MNPs, due to their distinctive physical properties and ability to role at the cellular and molecular level of biological interactions, are a major class of nano-scale materials with the potential to be useful in biomedical fields, such as, drug delivery, hyperthermia treatment, and magnetic resonance imaging (MRI) of cancer cells (Sun et al. Citation2008). Due to their nano-size, and their increasing surface-to-volume ratio, scientists have focused on the study of the relationship between structure and properties of MNPs. Configuration, size, morphology, and surface chemistry can be made-to-order by various processes to not only improve magnetic properties but also affect the performance of nanoparticles in vivo.
MRI has developed one of the most extensively used and powerful tools for non-invasive clinical diagnosis due to its high soft tissue contrast, three-dimensional resolution, and permeation depth (Lauffer et al. Citation1996). Furthermore, images are acquired without the use of ionizing radiation or radiotracers that would cause unwelcome harmful side effects. A considerable amount of research in medical MR imaging is focused on the development of contrast agents that can offer better delineation between healthy and diseased tissue. MNPs are a major class of nano-scale material currently under extensive development for improved diagnosis of a wide range of diseases, including cancer (Ferrari Citation2005), cardiovascular disease (Wickline et al. Citation2007), and neurological disease (Corot et al. Citation2004).
A number of polymers, already accepted by FDA, have been applied for the combination of iron oxide, such as polycaprolactone (PCL), polyethylene glycol (PEG), polylactic acid (PLA), polylactic-co-glycolic acid (PLGA), dextran, chitosan, and so on (Filippousi et al. Citation2013). The nano-scale magnitudes of MNPs give rise to unique magnetic properties and the ability to purpose on a cellular and molecular level. By way of contrast agents, MNPs can help refining imaging methods resolution, developing as powerful probes for both in vivo imaging in medical and biological diagnostics.
Concerning cancer ailment, MNPs can be applied also for therapy purpose such as hyperthermia and drug delivery. Regarding the hyperthermia therapy, when the particles are targeted to the cancer cells, it is conceivable to heat the tumor by fluctuating the applied magnetic field. In place of it, it is likely to use MNPs for hyperthermia-based drug molecule is released because of the heating ().
Figure 1. Present application of magnetic nanoparticles in cancer disease: diagnosis (MRI) and therapy (hyperthermia, drug delivery and controlled release). Reproduced with permission from Elsevier (Lima-Tenoria et al. Citation2015), Copyright 2015.
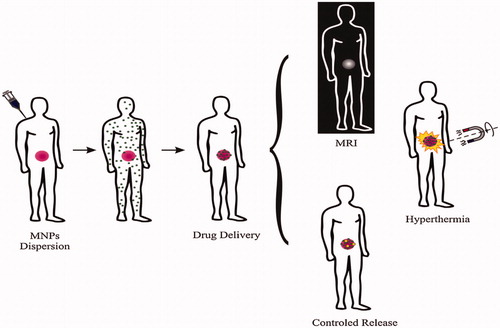
This review debates the special attention is devoted to the preparation, functionalization of MNPs and their exact functionalization in order to be used for cancer disease treatment.
Philosophies of MRI contrast
MRI applies a strong magnetic field that line up the magnetic moments of protons in a sample creating a balance magnetization along the z-axis (Mz) with a magnitude of M0. A radio frequency (RF) pulse, at a resonant frequency talented of transporting energy to protons, switches their magnetic moments away from the z-axis, in phase, to an angle called the flip angle (Gogola et al. Citation2013, Lauffer et al. Citation1996). The time essential for the magnetic moments to relax to the equilibrium state, which is generally termed the relaxation time, is tissue-reliant. MRI contrast in soft tissue is as a result of variances in the proton density, spin-net relaxation time (T1) and spin-spin relaxation time (T2) of the protons. T1 is the time constant of the exponential retrieval procedure of M0 along the z-axis after an RF pulse. For protons that relax more gradually (extended T1), full magnetization is not recovered before following RF pulses, and so they produce less signal and cause the saturation effect. T2 is the time constant of the exponential decline of the latitudinal magnetization (Mxy) after an RF pulse (Gogola et al. Citation2013). T2 is linked to the amount of time for the processing magnetic moments of protons to become randomly aligned in the xy-plane after an RF pulse, finally resulting in a net magnetic moment of zero in the xy-plane.
Spin-echo (SE) pulse arrangements are used to remove exterior magnetic field effects and can create T1 or T2 weighted images based on molecular interplay (Lauffer et al. Citation1996). In its most fundamental method, the SE scans apply two RF pulses with flip angles of 90° and 180° that create a spin echo. The 180° pulse helps to change the transverse magnetization, repealing DE phasing effects result of heterogeneity’s in the local magnetic field. The time among the requisition of the 90° pulse and the climax of the echo signal is labeled the echo time (TE) (Stephen et al. Citation2011). Inherent variances of T1 and T2 are minor and mostly objective materials are used clinically to improve the contrast between the tissue and the surrounding tissue.
T1 contrast agents are applied to increment signal strength providing positive contrast enhancement in T1-weighted images, whilst, T2 contrast agents reduction signal strength cause a negative contrast improvement in T2-weighted images (Budde and Frank Citation2009). The attendance of paramagnetic ions nearby water protons abbreviates their T1 relaxation time via coordination with water molecules providing enhanced contrast. Despite gadolinium chelates extensive usage, toxicity worries, low detection sensitivity, and their short blood flow times have caused to the continued advance of super-paramagnetic iron oxide nanoparticles (SPIONs) for T2 contrast improvement (Clarkson Citation2002, Kamaly and Miller Citation2010).
Provision of magnetic nanoparticles
It is clear that the confidants of the MNPs are connected to the procedure used for its preparation due to the strong affiliation of the magnetic properties on their morphology and structure. Different techniques have been planned for the synthesis of MNPs of different morphologies, surface chemistry, with precise consideration on synthesis of particles nearly of unvarying size and shape (Ahmed et al. Citation2012). Produce of uniform nanostructures and reproducibility are the essential challenge for chemical techniques. A short review of few typical procedures lead to the efficient preparation of the magnetic nanoparticles is debated beneath.
Hydrothermal synthesis
Synthesis of MNPs in hydrothermal method is founded on aqueous reactions going on under high pressure and high temperature to make or affect the formation of nano-crystals. This process is based on an overall phase transmission and departure mechanism happening at the interfaces of the liquid-solid, and solution phases present during the synthesis in at high temperature (in the range from 122 to 260 °C) and high steam pressure (0.3–4 MPa) (Wang et al. Citation2005).When the reaction is done, it is chilled to room temperature and washed with several solvents to eradicate non-conjugated stability and impurities agents (Lam et al. Citation2013). The size repartition and particle size enhancement with the forerunner concentration. But, the domicile time had a more important influence on the normal particle size than feed concentration (Hasany et al. Citation2013). Noble crystallization and easy control of product morphology, amid many other privileges of the hydrothermal synthesis, make it one of the most hopeful ways for nanoparticles manufacture (Li et al. Citation2014, Li et al. Citation2013). Studies on MNPs synthesis (by hydrothermal technique), and surface functionalization presented that the manufactured particles have a great potential application in MR imaging (Cai et al. Citation2013).
Thermal parsing
MNPs with controlled size can be produce through the thermal parsing technique. This procedure needs comparatively higher temperatures and intricate operation (Wu et al. Citation2008). Highly uniform MNPs are generally attained with thermal parsing of organometallic composites followed by oxidation in high-boiling temperature organic solvents with stabilizing surfactants (Shokrollahi Citation2013). Mostly oleic acid and hexadecylamine are used as role of surfactants (Lu et al. Citation2007). For the accurate control of morphology and particle size, the rein of the reaction time, temperature, furthermore to the proportion of the starting reagents. For example, produced iron oxide nanoparticles by thermal parsing technique of Fe(acac)3 (Prashant et al. Citation2010). After covering, heterograft tumor model MRI certified the benefits of the manufactured MNPs against Lumiren® through the improved permeation and maintenance effect of the tumor (Lai et al. Citation2012). The gained particles exhibit possibility to be used as contrast agent for the MRI. The main disadvantage of this method is that the prepared NPs are commonly only dissolved in non-polar solvents (Wu et al. Citation2008).
Sedimentation technique
Sedimentation technique is an easy and convenient method to make MNPs. This technique is based on the resuscitation of metallic elements, from salt solution, by surcharging NaOH solution, at room temperatures or at high temperatures. It has been proved that the attributes of the MNPs like size, figure depend on the type of salts used, the reaction temperature, the ionic power of the media and pH (Lu et al. Citation2007, Thanh Citation2012). The main benefit of the sedimentation technique is that a great amount of nanoparticles can be produced (Laurent et al. Citation2008). Because only kinetic doers are monitoring the growth of the crystal it is problematic to control particle size distribution (Salunkhe et al. Citation2014). In spite of such matters, uniform particles are usually made through homogeneous sedimentation reactions through a procedure that includes the differentiation of the nucleation and development of the nuclei (Hao et al. Citation2010). There are two phases are involved in this procedure: first a short blast of nucleation when the concentration of the sample attains critical super-saturation and then a slow development of the nuclei by dissemination of the solutes to the surface on the crystal (Bitar et al. Citation2014). A produced Fe3O4 nanoparticle by a sedimentation technique for hyperthermia applications is a best example for this method (Zhao et al. Citation2006). Dodecyl benzene sulfonic acid sodium salt was applied to avoid accumulation between the particles. It is revealed that the MNPs size, manufactured by co-sedimentation technique, changes from 8 to 16 nm by changing the forerunner to surfactant concentration (Surendra et al. Citation2014, Wang et al. Citation2011). Prepared magnetic fluid (MF) by this method showed excellent constancy and rapid magneto-temperature reaction, with possible application in both MRI and hyperthermia. This method’s extensive use can be clarified by the comfort of implementation and need for less risky materials and actions (Kossatz et al. Citation2014, Sanjai et al. Citation2014). Despite of being cost-effective, co-sedimentation is extensively applied for the production of MNPs for biomedical applications, like cancer diagnosis and therapy (Tiwari and Tiwari Citation2013).
Sol–gel
This technique is suitable for MNPs synthesis, and is founded on the hydrolysis and poly-condensation reactions of metal forerunners, metalloid element besieged by various reactive ligands to shape a colloidal system called “sol”. Then the “sol” is dehydrated by eliminating of solvent or by chemical reaction cause to the shaping of a liquid phase, named “gel” (Kalia et al. Citation2014) (). Since these reactions are done at low temperatures (25 °C), more heat treatments are essential for the concluding crystalline nanoparticles.
Figure 2. Scheme of an oil-in-water (O/W) micro-emulsion reaction method for synthesis of nanoparticles. Reproduced with permission from Elsevier (Lima-Tenoria et al. Citation2015), Copyright 2015.
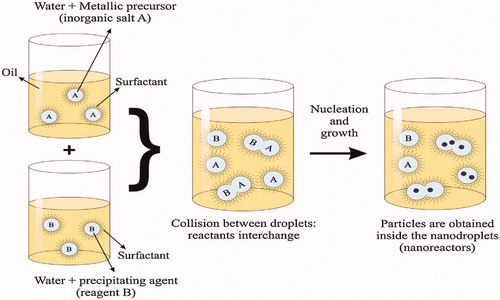
Sol–gel technique can be applied to produce unadulterated, stoichiometric, uniform and extensive size nanoparticles with comparatively slim size sharing in a large scale. Related advantages to this procedure of synthesis of MNPs are the ability to preset the structure of the nanoparticles, excellent size control of the nanoparticles, by the experimental circumstance, and the ability to attain particles with pure amorphous phases (Salunkhe et al. Citation2014). This method is best choice for making magnetic particles under solid circumstance (powder) and not very appropriate for making MRI contrast agents because of their low stability hydrous solution (Qiao et al. Citation2009). However magnetic materials prepared by sol–gel technique have presented potential applications especially in tumor therapy through heating (hyperthermia) (Saldívar-Ramírez et al. Citation2014, Sánchez et al. Citation2014).
Micro-emulsion technique
Micro-emulsion process based on a macroscopically analogous, isotropic and thermodynamically constant solution shaped by generally three phase 1. A polar phase commonly water 2. A non-polar phase usually oil 3. A surfactant The constancy of these emulsions is because of the surfactant molecules, which shape, on superficial film separating detach the polar and the non-polar areas. Various microstructures are created by the superficial layer, from a “sponge” phase to water droplets dispersed in a persistent oil phase (W/O – reverse micelle) to droplets of oil dispersed in a persistent water phase (O/W – normal micelle).
These nano-droplets can be employed as nano-reactors to perform the chemical reactions, and to create an appropriate condition for controlled nucleation and growing (Malik et al. Citation2012). By incessant impact of the nano-droplets, they incline to combine and break, blending the inorganic salt (A) and the precipitating agent (B), providing the precipitate, after nucleation and growth (). In final step, the nanoparticles are attained by removing the surfactants and, washing two times. The main benefit of this technique is that it has been shown to be suitable, multipurpose and, particle size can be easily controlled by moderating the parameters of the hydrous droplets. Though the low yield of synthesis nanoparticles compared with the co-sedimentation method, and the cleansing procedures required for removing the surfactants are some disadvantages of this technique (Qiao et al. Citation2009, Sharifi et al. Citation2012).
Figure 3. (a) Schematic depiction of ligand exchange strategy for very small-sized iron oxide nanoparticles using PO-PEG ligands and a TEM image of small-sized iron oxide nanoparticles before and after the ligand exchange. (b) Plot of 1/T1 over Fe concentration of iron oxide nanoparticles with diameters of 2.2 nm (▪), 3 nm (red •), and 12 nm (blue ▴). The slope designates the exact relaxivity (r1). (c) High resolution blood pool MR image following vein injection of small-sized iron oxide nanoparticles. Reproduced with permission from American Chemical Society (Kim et al. Citation2011), Copyright 2011.
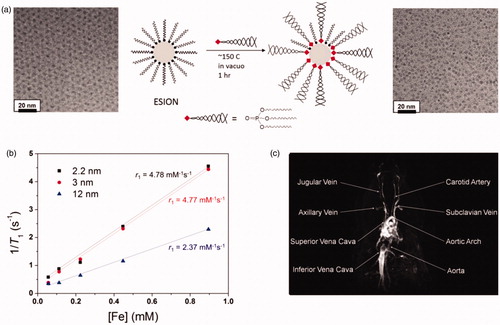
Important SPIONs design parameters
The SPIONs magnetic properties are affected by the size and crystallinity of the magnetite core. Furthermore, surface modifications comprising coating with polymers and tissue targeting agents can have a deep effect on the efficacy of SPIONs as MRI contrast agents.
The efficacy of these contrast agents to increment the rate of relaxation of nearby protons can be presented by the relaxivity (r1, r2), which is determined as the slope of the plot 1/T1 or 1/T2 as a subordinate of SPION concentration (Sun et al. Citation2004). These modifications can growth local concentrations of SPIONs in the tissue while declining the concentration in background tissue. Particle diameter reduction and increase of surface-to-volume ratios result in reduction in saturation magnetization which in reduces the T2 relaxation sufficiency of SPIONs (Lu et al. Citation2007). The SPIONs with greater relaxivity properties and in vivo kinetics can be attained by considering the parameters of core synthesis and post-synthesis modifications.
Surface modification
For numerous biomedical applications, mono-dispersed MNPs nanoparticles produced by the heat-up technique in hydrophobic solvents require surface modification procedures to attain biocompatibility and prolonged stability in biological environment. Ligand exchange and encapsulation with amphiphilic polymers, are the two most popular procedures, have been applied to produce hydrophilic and biocompatible iron oxide nanoparticles. Additionally, manifold interaction ligands have been created by incorporating beneficial features of the ligand exchange and the encapsulation procedures to get highly water dispersible iron oxide nanoparticles.
Ligand exchange
The most prevalent method to transform hydrophobic nanoparticles created in organic solvents into water-dispersible and biocompatible nanoparticles is ligand exchange of hydrophobic covering ligand with new hydrophilic ligand. In this method, the binding ability of the hydrophilic ligand to the nanoparticle surface is vital to warrant a stable scattering. Anchoring groups such as carboxylic acids, amines, dopamine. Phosphine are good examples that generally have a good dependence to iron oxide surface (Kim and Bawendi Citation2003, Xu et al. Citation2004). Though the many amounts of the ligands are often required for the ligand exchange reaction. Polyethylene glycol derived phosphine oxide (PO-PEG) ligand has been confirmed to relocate oleic acid ligand in the iron oxide nanoparticles made by the heat-up technique to produce water-dispersible iron oxide nanoparticles () (Na et al. Citation2007). Furthermore, a typical reaction with multiple functional reagents such as 1,2-ethylenediamine can donate these PO-PEG ligands with active functional groups that let them to conjugate with imaging or targeting agents. Very small-sized iron oxide nanoparticles can also be transported to hydrous environment through ligand exchange with PO-PEG ligands (Kim et al. Citation2011). The subsequent hydrophilic small-sized iron oxide nanoparticles with a diameter of 4 nm show a high r1 relaxivity of 4.78 mM−1 s−1 and a low r2/r1 ratio of 6.12 (). Furthermore to the effective T1 MR Contrast agent, PO-PEG covered small-sized iron oxide nanoparticles can also be applied as high-resolution MR imaging agent with their longer flow time than that of the gadolinium complex-based contrast agents that are presently used in the clinic (). For the ligand exchange of hydrophobic iron oxide nanoparticles catechol-functionalized polypeptides have been developed (Park et al. Citation2014). The subsequent multimodal transfection agents (MTAs) with a hydrodynamic diameter of 43 nm show greatly improved colloidal stability in hydrous media. The nanoparticles are talented of binding DNA molecules to stem cells for gene transition, and show an improved efficacy in human mesenchymal stem cells compared with prevalent transfection agents such as lipofectamine.
Figure 4. (a) Schematic representation of ligand encapsulation method for FIONs using lipid-PEG ligands. (b) TEM image of 31 nm FIONs applying lipid-PEG ligands. (b) TEM image of 22 nm FIONs dispersed in water (inset: HRTEM image). (c) Plots of r2 values of FIONs of different core sizes. FIONs with core dimension of 31 nm and encapsulated with 2100 Da phospholipid PEG exhibit an extremely high r2 relaxivity of 741 mM−1 s−1. (d) In vivo MR images of a tumor site before (left) and 1 h after (right) vein injection of FIONs (arrows indicate the tumor site) showing the significant reduction in the MR signal. Reproduced with permission from American Chemical Society (Lee et al. Citation2012), Copyright 2012.
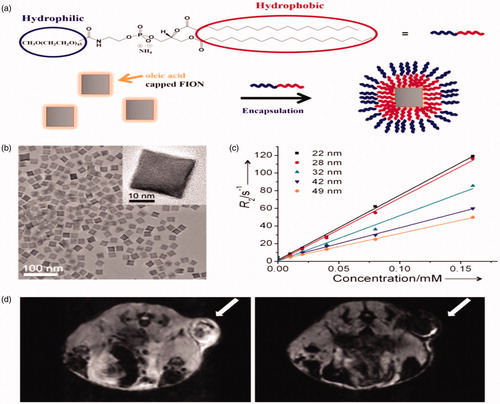
Ligand encapsulation
Another prevalent method to get biocompatible nanoparticles for numerous medical applications is encapsulation of nanoparticles within self-assembled amphiphilic polymers. Among many types of polymers, FDA accepted PEG-terminated phospholipid is broadly used, where the phospholipid-based ligand is attached to nano-crystal surface and then shapes micelles with hydrophobic van der Waals interaction, while the outer PEG chain provides colloidal stability in hydrous environment () (Dubertret et al. Citation2002). Since the molecular weight of the phospholipid-PEG ligands regulates the shell thickness of the subsequent nanoparticles, it has an significant role in characterizing the relaxivity of the iron oxide nanoparticles. For instance, the relaxivity of MNPs with core diameter of 15 nm and encapsulated within 1300 Da PEG-terminated phospholipid is 2.84 times higher than that encapsulated with 4500 Da PEG-phospholipid (Tong et al. Citation2010). This encapsulation technique can also be applied to make water-dispersible ferrimagnetic iron oxide nanocubes (FIONs), which are very labile in hydrous environment and easily form accumulates because of their high magnetic attractive force (Lee et al. Citation2012). These FIONs have been employed to image intrahepatically relocated pancreatic isles in a pig model by a 1.5 Tesla clinical MRI scanner, which has important role in the remedy of type 1 diabetes mellitus. Also, the hypothetically foretold maximum r2 relaxivity could be attained by improving the size of FIONs (). FIONs with core dimension of 31 nm and encapsulated with 2100 Da phospholipid-PEG show an very high r2 relaxivity of 741 mM−1 s−1 and high colloidal stability in aqueous media (Lee et al. Citation2012). Gathering of FIONs at tumor after vein injection allows the high resolution in vivo MR imaging of tumors using a clinical 2.1 T MR scanner (). Furthermore to PEG-based ligands, usual polymers such as polysaccharides and polypeptides have been applied as stabilizers for iron oxide nanoparticles.
Figure 5. Stabilization of nanoparticles through manifold interface ligands. (a) Formation of hydrophilic nanoparticles by manifold interface ligands. (a) Formation of water-dispersible nanoparticles by multiple interaction ligand (MIL) stabilization. (b) In vivo stability tests: blood circulation data (plasma iron concentration versus time relationships) of MIL0, MIL1, and MIL2-functionalized Fe3O4 nanoparticles (inset). (c) Time-dependent T2-weighted MR images of the lymph node (arrow) in a nude mouse before and after intravenous administration of MIL2-functionalized Fe3O4 nanoparticles. Reproduced with permission from Wiley-VCH (Ling et al. Citation2011), Copyright 2011.
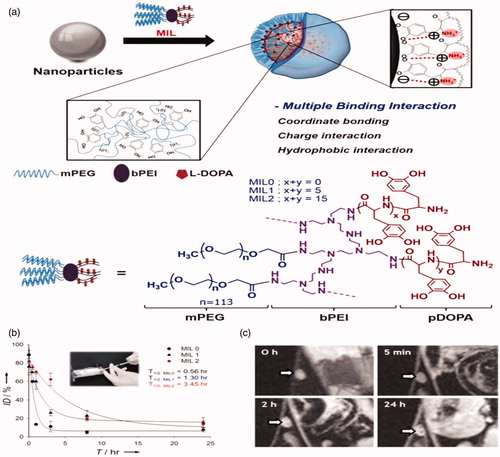
Manifold interface ligands
Ligand exchange and the encapsulation procedures are applied to change hydrophobic nanoparticles into hydrophilic and biocompatible nanoparticles, so grouping of these two characteristics within one ligand system will cause to highly hydrophilic nanoparticles for many biomedical applications.
Manifold interface ligands (MILs) such catechol and amine end groups like naturally occurring mussel-adhesive protein as basis, poly(l-3,4-dihydroxyphenylalanine) (poly DOPA)-based are using direct attaching with iron oxide nanoparticles, while amphiphilic branched block copolymer shapes micelles that encapsulate the nanoparticles.
Furthermore, the positive charge of the ligand permits for electrostatic interaction with most negatively charged nanoparticle surfaces (). The subsequent MIL-covered iron oxide nanoparticles show a very high stability in many harsh aqueous media, including highly acidic and basic environments, and even boiling water. Because of this outstanding stability, MIL-covered nanoparticles have a comparatively long blood half-life in rats compared with the already reported iron oxide-based nanoparticles (Gu et al. Citation2012, Jarzyna et al. Citation2009). Consequently, they can be gathered in the lymph nodes of a nude mouse 24 h after vein injection, which was obviously detected by T2-weighted MRI ().
Figure 6. (a) In vivo MR images of Bel-7402 subcutaneous xenograft in nude mice attained after administration of Gd-DTPA (top row), Feridex (the second row), unmodified (the third row), and FA-BSA-modified magnetic micelles (bottom row) at different time points (10 min, 1, 24, 48, and 72 h). (b) Tumor-to-muscle signal ratios observed on T1 images of the groups treated with Gd-DTPA at different time points different time points (10 min, 1, 24, 48, and 72 h). (c) Tumor-to-muscle signal ratios observed on T2 images of the groups treated with Feridex, unmodified magnetic micelles, and FA-BSA modified magnetic micelles at different time points (10 min, 1, 24, 48, and 72 h). TMR, tumor-to-muscle signal ratios. Tumor volume: 400–700 mm3, n = 3–5 for each group (Li et al., Citation2015). Reproduced with permission from Elsevier (Li et al. Citation2015), Copyright 2015.
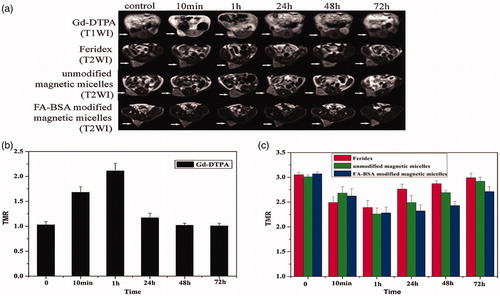
Cancer diagnosis with magnetic nanoparticles
Magnetic properties of hydrogen protons and its engagement with both a large robust external magnetic field and a transverse RF pulse to make the highly detailed images of the human body (Shokrollahi et al. Citation2014) are the basic principles of MRI. The use of radio waves tends to disturb the aligned protons, which return to their original state by a relaxation phenomenon. Through the relaxation phenomenon, a local disparity in proton density of a tissue that lead to the MRI image that could be observed. MNPs gathered in the tissue are responsible to creation MR contrast enhancement by reducing both T1 and T2 relaxation of surrounding protons (Singh and Sahoo Citation2014). Abdoscan®, GastroMARK®, Lumiren®, Resovist®, are some instances of iron oxide contrast agents which have been used as contrast agents to improve cancer detection, diagnosis, and also therapeutic management of solid tumors. Produced bio-functionalized chitosan and Fe3O4 nanoparticles by merging Fe3O4 and chitosan (CS) chemically improved with PEG and lactobionic acid in one step. These functional groups allow the nanoparticles more biocompatible (PEG) and the liver targeting (lactobionicacid acid groups) (Song et al. Citation2015). Before vein administration of MNPs, no change was detected to the parenchymal signal strength of in vivo MRI of the livers of SD rats. Though this signal was reduced after the nanoparticles administration, proving that these nanoparticles has liver targeting function and represents potential tools for applications in the biomedical field. Nickel–ferrite synthesis by a chemical co-precipitation technique with simultaneous chitosan coating showed to be appropriate as both T1 (22% signal enhancement in the T1-weighted image) and T2 (74% signal loss in the T2-weighted image) contrast agents in MRI in animal experimentation (Ahmad et al. Citation2015). It was observed that the T2 signal intensity in the mice treated with the SPIONs-loaded amphiphilic co-polymeric micelles based on mono methacrylate (PEGMA), functionalized with folate conjugated bovine serum albumin (FA-BSA) reduction most meaningfully between 2 h and 22 h, while the tumors with Feridex® and unmodified magnetic micelles show slight contrast change in the same period () (Li et al. Citation2015). Long circulation time and particles tumor tissue delivery could be increased by PEGMA and FA-BSA modification of the magnetic micelles and the uptake of magnetic micelles by the tumor could be improved by folate-mediated active targeting.
Figure 7. Schematic depiction of magnetic thermal therapy. Magnetic nanoparticles gather in tumors, aiming the cancer cells. When exposed to an alternating magnetic field, the nanoparticles incline to align with the applied field absorbing energy. MNPs fluctuating convert this electromagnetic energy into heat, increasing the temperature at this region. Reproduced with permission from Elsevier (Lima-Tenoria et al. Citation2015), Copyright 2015.
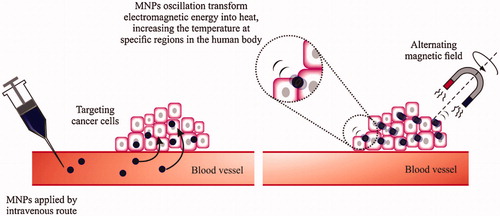
Role of magnetic nanoparticles in cancer treatment
Thermal therapies
Hyperthermia means unusually high body temperature that can be used as part of treatment, by employing heat at an affected site for remedial purposes. In this method tissue is exposed to high temperatures (43–49 °C) that can harm and destroy cancer cells. In the nineteenth century, the useful effects of thermal therapy in the cure of cancer were observed for the first time (Hervault and Thanh Citation2014).
It was revealed that the cancer cells show more sensitivity to hyperthermia (undertake apoptosis at temperatures of 41–46 °C), compared with the normal cells in the 1970s. Though some damaging side effects was detected at the surrounding healthy tissues by increasing the intracellular temperature. With the MF hyperthermia (MFH), a key to this problem was possible, where MNPs are used as intermediaries of heat for a local treatment. MFH is based on the injection of MNPs colloidal suspension that gathers at the tumor both actively (by the use of ligands at MNPs surface, exact for the surface receptors present on cancer cells) or inactively (by the improved permeability and retention (EPR) effect of MNPs). The MNPs accumulate at tumor cells can convert the electromagnetic energy into heat by fluctuating of the exterior magnetic field. The benefit of using MNPs is to evade the surrounding healthy tissue heating (). This technique is suggested as a comparatively non-toxic and a non-invasive method for cancer treatment, different from the old-style therapy methods.
Some advantages of this method over the traditional ones are (i) more permeable through the biological cells wall and less aggressive of its injection; (ii) the encapsulation process could be useful in the proper MNPs targeting properties; (iii) MNPs can be used as both treatment and diagnostic agent for MRI Simultaneously; (iv) no damage to normal cell (Hervault and Thanh Citation2014).
It was observed that synthesized paramagnetic iron oxide nanoparticles and loaded into mouse monocyte/macrophage-like cells, which have been shown to be tumor homing cells, penetrate pancreatic tumors when injected without penetrating other organs. Then mice were exposed to an intermittent magnetic field for 15 min to cause the cell delivered nanoparticles to produce heat after the injection (2 d) (Basel et al. Citation2012). These consequences claim that this hyperthermia system using nanoparticles is able to heat the tumor precisely without distressing healthy tissue.
Drug delivery based on MNPs
Being non-specificity of the drugs, damaging both sick and healthy cells is the main disadvantage on chemotherapy treatment method. MNPs can be used to solve this problem by delivering the healing agents to the chosen target area, keeping the particles at the specific site during the drug release, when combined with an external magnetic field. Keep drug levels in the required concentration range for therapy, evading the over dosage and dipping side effects are the factors must be considered in a controlled drug delivery based on MNPs. The therapeutic agent joined to the surface of the MNPs or encapsulated inside a nano-composites combination of a polymer and MNP drug delivery systems.
By this technique the cytotoxic drug can be protected till reaching the cancer cells, where the drug molecule will provide its therapeutic effect. Condition-dependent manner like heat or pH or degradation of the transporter particles can activate releasing of the encapsulated anticancer drug in polymer-based magnetic drug delivery. To enhance the outcome of drugs, the release of the active agent must befall over period of time, consequently increasing the efficacy of the drug. It is reported that magnetic field shows a reduction on drug release rates because of the accumulation of the magnetic nanohybrid particles (Zhang et al. Citation2009), it is also reported that the MNPs based in Fe3O4 and SiO2 nanoparticles revealed to be sensitive to both pH environment and magnetic field (Yu et al. Citation2013).
There are some advantages of MNPs such as (i) easy control of the drug release (ii) the fluctuating of magnetic field can produce hyperthermia and, thus, trigger drug release (as therapeutic agents) (iii) the same particles injected in a patient can be used as contrast in MRI either (as diagnostic agents). In another study of the application of MNPs on drug delivery it is reported that 5-fluorouracil loaded magnetic PLGA nan capsules were attained for magnetically target toward CT26 colon tumor model in mice (Shakeri-Zadeh et al. Citation2015). The outcomes confirmed that the synthesized nanoparticles showed outstanding anti-tumor activity against the colon cancer tumor. Dipping the amount of the required dosages of drugs because of its concentration at the cancer cells, and consequently, falling the side effects of the drug are the results of apply magnetic drug targeting procedure. Dual drug-loaded nanocapsules can be produced by preparation of a core–shell nanostructure, amassed from iron oxide nanoparticles, polyacrylic acid (PAA) and polyvinyl alcohol (PVA). This nanostructure could accommodate both curcumin and doxorubicin and As an exciting outcome vein injection followed by magnetic targeting in brain tumor of mice, allowed the high accretion at the targeted site, and repressed cancer growth in vivo more efficiently than does the delivery of either drug distinctly. Magnetic particles loading into liposome allows for magnetic targeting. Thus, lipid vesicles are getting to be more like the lipid-polymer membrane artificial cells (Chang, 1969d, 1972a) and no longer pure lipid vesicles. One major advantage of lipid vesicles is its ability to fuse with cellular membranes or membranes of intracellular organelles. This allows for much versatility in their ability to deliver drugs to different sites of the cells (Abbasi et al. Citation2016a, Abbasi et al. Citation2016b, Alizadeh et al. Citation2016, Chang Citation1966, Chung et al. Citation2016, Daraee et al. Citation2016a, Daraee et al. Citation20146b, Davoudi et al. Citation2014, Eatemadi et al. Citation2016, Ebrahimi et al. Citation2016, Fekri Aval et al. Citation2016, Hosseininasab et al. Citation2014, Nasrabadi et al. Citation2016, Sadat Tabatabaei Mirakabad et al. Citation2016).
Conclusion
A great number of multifunctional nanomaterials have also appeared, with special focus in biomedical applications with the advances in nanotechnology. MNPs have been widely studied as MRI contrast agents and numerous formulations have been accepted for clinical use. Though, their extensive utility has yet to be comprehended because of boundaries of current detection limits and a lack of tissue specificity. New progresses in both MRI achievement approaches and SPION synthesis and post-synthesis alterations have led to important developments in SPION detection sensitivity and in vivo bio-distribution. The enhancement of existing detection restrictions is essential for the successful translation of newly designed MNPs to extensive clinical application. Due to their magnetic properties and easiness of functionalization, SPIONs have increased interest as theranostic agents able to both providing delivering therapeutics and diagnostic information. Ligand exchange and encapsulation with amphiphilic polymers, are the two most popular procedures, have been employed to produce water-dispersible and biocompatible iron oxide nanoparticles. Advance new approaches to decrease side effects related to both old-style diagnosis and therapy for cancer disease is one of the biggest challenges facing the medical research. There is a unceasing advancement in the development of MNPs with potential to be useful in cancer disease. MNPs presented great potential to be functional in MRI, as contrast agents. Furthermore by fluctuating of an external magnetic field the magnetic energy of the particles is transformed to thermal energy (heat), destroying the cancer cells by the temperature increasing (hyperthermia) or releasing of the anticancer healing agents at the specific targeted cells by the drug delivery base on hyperthermia technique. Incorporate cancer diagnosis and therapy to enable these to perform as theranostic agent in a dual-purpose nanoparticles is one of the future aims on MNPs applications.
Authors’ contributions
M.F. and S.S.K. conceived the study and participated in its design and coordination. A.A. and S.D. participated in the sequence alignment and drafted the manuscript. All authors helped in drafting the manuscript. All authors read and approved the final manuscript.
Funding information
This work is funded by 2016 Drug Research Center, Tabriz University of Medical Sciences Grant.
Disclosure statement
The authors declare that they have no competing interests.
References
- Abbasi E, Akbarzadeh A, Kouhi M, Milani M. 2016a. Graphene: synthesis, bio-applications, and properties. Artif Cells Nanomed Biotechnol. 44:150-156.
- Abbasi E, Milani M, Fekri Aval S, Kouhi M, Akbarzadeh A, Tayefi Nasrabadi H, et al. 2016b. Silver nanoparticles: synthesis, properties, bio-applications and limitations. Crit Rev Microbiol. 42:173-180.
- Ahmad T, Bae H, Iqbal Y, Rhee I, Hong S, Chang Y, et al. 2015. Chitosan-coated nickel-ferrite nanoparticles as contrast agents in magnetic resonance imaging. J Magnet Magn Mater. 381:151–157.
- Ahmed N, Michelin-Jamois M, Fessi H, Elaissari A. 2012. Modified double emulsion process as a new route to prepare submicron biodegradable magnetic/polycaprolactone particles for in vivo theranostics. Soft Matter. 8:2554–2564.
- Alizadeh E, Zarghami N, Eslaminejad M, Reza B, Akbarzadeh A, Barzegar A, Abolghasem Mohammadi S. 2016. The effect of dimethyl sulfoxide (DMSO) on hepatic differentiation of mesenchymal stem cells. Artif Cells Nanomed Biotechnol. 44:157-164.
- Basel MT, Balivada S, Wang H, Shrestha TB, Seo GM, Pyle M, et al. 2012. Cell-delivered magnetic nanoparticles caused hyperthermia-mediated increased survival in a murine pancreatic cancer model. Int J Nanomedicine. 7:297–306.
- Bitar A, Kaewsaneha C, Eissa MM, Jamshaid T, Tangboriboonrat P, Polpanich D, et al. 2014. Ferrofluids: from preparation to biomedical applications. J Colloid Sci Biotechnol. 3:3–18.
- Budde MD, Frank JA. 2009. Magnetic tagging of therapeutic cells for MRI. J Nucl Med. 50:171–174.
- Cai H, An X, Cui J, Li J, Wen S, Li K, et al. 2013. Facile hydrothermal synthesis and surface functionalization of polyethyleneimine-coated iron oxide nanoparticles for biomedical applications. ACS Appl Mater Interfaces. 5:1722–1731.
- Chang TMS. 1966. Semipermeable aqueous microcapsules (“artificial cells”): with emphasis on experiments in an extracorporeal shunt system. Trans Am Soc Artif Intern Organs. 12:13–19.
- Chung J-H, Kim YK, Kim K-H, Kwon T-Y, Ziba Vaezmomeni S, Samiei M, et al. 2016. Synthesis, characterization, biocompatibility of hydroxyapatite-natural polymers nanocomposites for dentistry applications. Artif Cells Nanomed Biotechnol. 44:277-284.
- Clarkson R. 2002. Blood-pool MRI Contrast Agents: Properties and Characterization. Springer, pp. 2012–2035.
- Corot C, Petry KG, Trivedi R, Saleh A, Jonkmanns C, Le Bas J-F, et al. 2004. Macrophage imaging in central nervous system and in carotid atherosclerotic plaque using ultrasmall superparamagnetic iron oxide in magnetic resonance imaging. Investig Radiol. 39:619–625.
- Daraee H, Eatemadi A, Abbasi E, Fekri Aval S, Kouhi M, Akbarzadeh A. 2016a. Application of gold nanoparticles in biomedical and drug delivery. Artif Cells Nanomed Biotechnol. 44:410-422.
- Daraee H, Etemadi A, Kouhi M, Alimirzalu S, Akbarzadeh A. 2016b. Application of liposomes in medicine and drug delivery. Artif Cells Nanomed Biotechnol. 44:381-391.
- Davoudi Z, Akbarzadeh A, Rahmatiyamchi M, Akbar Movassaghpour A, Alipour M, Nejati-Koshki K, et al. 2014. Molecular target therapy of AKT and NF-kB signaling pathways and multidrug resistance by specific cell penetrating inhibitor peptides in HL-60 cells. Asian Pacific J Cancer Prev. 15:4353.
- Dubertret B, Skourides P, Norris DJ, Noireaux V, Brivanlou AH, Libchaber A. 2002. In vivo imaging of quantum dots encapsulated in phospholipid micelles. Science. 298:1759–1762.
- Eatemadi A, Daraee H, Zarghami N, Melat Yar H, Akbarzadeh A, Hanifehpour Y. 2016. Nanofiber: synthesis and biomedical applications. Artif Cells Nanomed Biotechnol. 44:111-121.
- Ebrahimi E, Abbasi E, Akbarzadeh A, Ahmad Khandaghi A, Davaran S. 2016. Novel drug delivery system based on doxorubicin-encapsulated magnetic nanoparticles modified with PLGA-PEG1000 copolymer. Artif Cells Nanomed Biotechnol. 44:290-297.
- Fekri Aval S, kbarzadeh AA, Yamchi MR, Zarghami F, Nejati-Koshki K, Zarghami N. 2016. Gene silencing effect of SiRNA-magnetic modified with biodegradable copolymer nanoparticles on hTERT gene expression in lung cancer cell line. Artif Cells Nanomed Biotechnol. 44:188-193.
- Ferrari M. 2005. Cancer nanotechnology: opportunities and challenges. Nat Rev Cancer. 5:161–171.
- Filippousi M, Papadimitriou SA, Bikiaris DN, Pavlidou E, Angelakeris M, Zamboulis D, et al. 2013. Novel core–shell magnetic nanoparticles for Taxol encapsulation in biodegradable and biocompatible block copolymers: preparation, characterization and release properties. Int J Pharm. 448:221–230.
- Gogola D, Štrbák O, Škrátek M, Frollo I. 2013. Contrast agents based on magnetic nanoparticles and its interaction with surrounding environment during contrast imaging. In: MEASUREMENT 2013, Proceedings of the 9th International Conference, Smolenice, Slovakia; pp. 299–302.
- Gu L, Fang RH, Sailor MJ, Park J-H. 2012. In vivo clearance and toxicity of monodisperse iron oxide nanocrystals. ACS Nano. 6:4947–4954.
- Hao R, Xing R, Xu Z, Hou Y, Gao S, Sun S. 2010. Synthesis, functionalization, and biomedical applications of multifunctional magnetic nanoparticles. Adv Mater. 22:2729–2742.
- Hasany SF, Abdurahman NH, Sunarti AR, Jose R. 2013. Magnetic iron oxide nanoparticles: chemical synthesis and applications review. Curr Nanosci. 9:561–575.
- Hervault A, Thanh NTK. 2014. Magnetic nanoparticle-based therapeutic agents for thermo-chemotherapy treatment of cancer. Nanoscale. 6:11553–11573.
- Hosseininasab S, Pashaei-Asl R, Ahmad Khandaghi A, Tayefi Nasrabadi H, Nejati-Koshki K, Akbarzadeh A, et al. 2014. Synthesis, characterization, and in vitro studies of PLGA‐PEG nanoparticles for oral insulin delivery. Chem Biol Drug Design. 84:307–315.
- Jarzyna PA, Skajaa T, Gianella A, Cormode DP, Samber DD, Dickson SD, et al. 2009. Iron oxide core oil-in-water emulsions as a multifunctional nanoparticle platform for tumor targeting and imaging. Biomaterials. 30:6947–6954.
- Kalia S, Kango S, Kumar A, Haldorai Y, Kumari B, Kumar R. 2014. Magnetic polymer nanocomposites for environmental and biomedical applications. Colloid Polym Sci. 292:2025–2052.
- Kamaly N, Miller AD. 2010. Paramagnetic liposome nanoparticles for cellular and tumour imaging. Int J Mol Sci. 11:1759–1776.
- Kim S, Bawendi MG. 2003. Oligomeric ligands for luminescent and stable nanocrystal quantum dots. J Am Chem Soc. 125:14652–14653.
- Kim BH, Lee N, Kim H, An K, Park YI, Choi Y, et al. 2011. Large-scale synthesis of uniform and extremely small-sized iron oxide nanoparticles for high-resolution T1 magnetic resonance imaging contrast agents. J Am Chem Soc. 133:12624–12631.
- Kossatz S, Ludwig R, Dähring H, Ettelt V, Rimkus G, Marciello M, et al. 2014. High therapeutic efficiency of magnetic hyperthermia in xenograft models achieved with moderate temperature dosages in the tumor area. Pharm Res. 31:3274–3288.
- Lima-Tenório MK, Gómez Pineda EA, Ahmad NM, Fessi H, Elaissari A. 2015. Magnetic nanoparticles: in vivo cancer diagnosis and therapy. Int J Pharm. 493:313–327.
- Lai S-M, Hsiao J-K, Yu H-P, Lu C-W, Huang C-C, Shieh M-J, et al. 2012. Polyethylene glycol-based biocompatible and highly stable superparamagnetic iron oxide nanoclusters for magnetic resonance imaging. J Mater Chem. 22:15160–15167.
- Lam T, Pouliot P, Avti PK, Lesage F, Kakkar AK. 2013. Superparamagnetic iron oxide based nanoprobes for imaging and theranostics. Adv Colloid Interface Sci. 199–200:95–113.
- Lauffer R, Edelman R, Zlatkin M, Hesselink J, Saunders W. 1996. MRI Clinical Magnetic Resonance Imaging. Philadelphia (PA): WB Saunders Co.
- Laurent S, Forge D, Port M, Roch A, Robic C, Vander Elst L, et al. 2008. Magnetic iron oxide nanoparticles: synthesis, stabilization, vectorization, physicochemical characterizations, and biological applications. Chem Rev. 108:2064–2110.
- Lee N, Choi Y, Lee Y, Park M, Moon WK, Choi SH, et al. 2012. Water-dispersible ferrimagnetic iron oxide nanocubes with extremely high r2 relaxivity for highly sensitive in vivo MRI of tumors. Nano Lett. 12:3127–3131.
- Li J, Zheng L, Cai H, Sun W, Shen M, Zhang G, et al. 2013. Polyethyleneimine-mediated synthesis of folic acid-targeted iron oxide nanoparticles for in vivo tumor MR imaging. Biomaterials. 34:8382–8392.
- Li J, He Y, Sun W, Luo Y, Cai H, Pan Y, et al. 2014. Hyaluronic acid-modified hydrothermally synthesized iron oxide nanoparticles for targeted tumor MR imaging. Biomaterials. 35:3666–3677.
- Li H, Yan K, Shang Y, Shrestha L, Liao R, Liu F, et al. 2015. Folate-bovine serum albumin functionalized polymeric micelles loaded with superparamagnetic iron oxide nanoparticles for tumor targeting and magnetic resonance imaging. Acta Biomater. 15:117–126.
- Ling D, Park W, Park YI, Lee N, Li F, Song C et al. 2011. Multiple-interaction ligands inspired by mussel adhesive protein: synthesis of highly stable and biocompatible nanoparticles. Angewandte Chemie International Edition. 50:11360–11365.
- Lu A-H, Salabas EL, Schüth F. 2007. Magnetic nanoparticles: synthesis, protection, functionalization, and application. Angewandte Chem Int Ed. 46:1222–1244.
- Malik MA, Wani MY, Hashim MA. 2012. Microemulsion method: a novel route to synthesize organic and inorganic nanomaterials: 1st nano update. Arab J Chem. 5:397–417.
- Na HB, Lee IS, Seo H, Park YI, Lee JH, Kim S-W, et al. 2007. Versatile PEG-derivatized phosphine oxide ligands for water-dispersible metal oxide nanocrystals. Chem Commun. 48:5167–5169.
- Nasrabadi HT, Abbasi E, Davaran S, Kouhi M, Akbarzadeh A. 2016. Bimetallic nanoparticles: preparation, properties, and biomedical applications. Artif Cells Nanomed Biotechnol. 44:376-380.
- Park W, Yang HN, Ling D, Yim H, Kim KS, Hyeon T, et al. 2014. Multi-modal transfection agent based on monodisperse magnetic nanoparticles for stem cell gene delivery and tracking. Biomaterials. 35:7239–7247.
- Prashant C, Dipak M, Yang C-T, Chuang K-H, Jun D, Feng S-S. 2010. Superparamagnetic iron oxide – Loaded poly (lactic acid)-d-α-tocopherol polyethylene glycol 1000 succinate copolymer nanoparticles as MRI contrast agent. Biomaterials. 31:5588–5597.
- Qiao R, Yang C, Gao M. 2009. Superparamagnetic iron oxide nanoparticles: from preparations to in vivo MRI applications. J Mater Chem. 19:6274–6293.
- Sadat Tabatabaei Mirakabad F, Akbarzadeh A, Milani M, Zarghami N, Taheri-Anganeh M, Zeighamian V, Badrzadeh F, Rahmati-Yamchi M. 2016. A comparison between the cytotoxic effects of pure curcumin and curcumin-loaded PLGA-PEG nanoparticles on the MCF-7 human breast cancer cell line. Artif Cells Nanomed Biotechnol. 44:423-430.
- Saldívar-Ramírez M, Sánchez-Torres C, Cortés-Hernández D, Escobedo-Bocardo J, Almanza-Robles J, Larson A, et al. 2014. Study on the efficiency of nanosized magnetite and mixed ferrites in magnetic hyperthermia. J Mater Sci Mater Med. 25:2229–2236.
- Salunkhe AB, Khot VM, Pawar SH. 2014. Magnetic hyperthermia with magnetic nanoparticles: a status review. Curr Top Med Chem. 14:572–594.
- Sánchez J, Cortés-Hernández D, Escobedo-Bocardo J, Jasso-Terán R, Zugasti-Cruz A. 2014. Bioactive magnetic nanoparticles of Fe–Ga synthesized by sol–gel for their potential use in hyperthermia treatment. J Mater Sci Mater Med. 25:2237–2242.
- Sanjai C, Kothan S, Gonil P, Saesoo S, Sajomsang W. 2014. Chitosan-triphosphate nanoparticles for encapsulation of super-paramagnetic iron oxide as an MRI contrast agent. Carbohydr Polym. 104:231–237.
- Shakeri-Zadeh A, Khoee S, Shiran M-B, Sharifi AM, Khoei S. 2015. Synergistic effects of magnetic drug targeting using a newly developed nanocapsule and tumor irradiation by ultrasound on CT26 tumors in BALB/c mice. J Mater Chem B. 3:1879–1887.
- Sharifi I, Shokrollahi H, Amiri S. 2012. Ferrite-based magnetic nanofluids used in hyperthermia applications. J Magnet Magn Mater. 324:903–915.
- Shokrollahi H. 2013. Structure, synthetic methods, magnetic properties and biomedical applications of ferrofluids. Mater Sci Eng C Mater Biol Appl. 33:2476–2487.
- Shokrollahi H, Khorramdin A, Isapour G. 2014. Magnetic resonance imaging by using nano-magnetic particles. J Magnet Magn Mater. 369:176–183.
- Singh A, Sahoo SK. 2014. Magnetic nanoparticles: a novel platform for cancer theranostics. Drug Discov Today. 19:474–481.
- Song X, Luo X, Zhang Q, Zhu A, Ji L, Yan C. 2015. Preparation and characterization of biofunctionalized chitosan/Fe3O4 magnetic nanoparticles for application in liver magnetic resonance imaging. J Magnet Magn Mater. 388:116–122.
- Stephen ZR, Kievit FM, Zhang M. 2011. Magnetite nanoparticles for medical MR imaging. Mater Today. 14:330–338.
- Sun S, Zeng H, Robinson DB, Raoux S, Rice PM, Wang SX, et al. 2004. Monodisperse MFe2O4 (M = Fe, Co, Mn) nanoparticles. J Am Chem Soc. 126:273–279.
- Sun C, Lee JSH, Zhang M. 2008. Magnetic nanoparticles in MR imaging and drug delivery. Adv Drug Deliv Rev. 60:1252–1265.
- Surendra MK, Annapoorani S, Ansar EB, Varma PH, Rao MR. 2014. Magnetic hyperthermia studies on water-soluble polyacrylic acid-coated cobalt ferrite nanoparticles. J Nanopart Res. 16:1–14.
- Thanh NT. 2012. Magnetic Nanoparticles: From Fabrication to Clinical Applications. eBook: CRC press (Taylor & Francis Group).
- Tiwari A, Tiwari A. 2013. Bioengineered Nanomaterials. eBook: CRC Press(Taylor & Francis Group).
- Tong S, Hou S, Zheng Z, Zhou J, Bao G. 2010. Coating optimization of superparamagnetic iron oxide nanoparticles for high T2 relaxivity. Nano Lett. 10:4607–4613.
- Wang X, Zhuang J, Peng Q, Li Y. 2005. A general strategy for nanocrystal synthesis. Nature. 437:121–124.
- Wang YM, Cao X, Liu GH, Hong RY, Chen YM, Chen XF, et al. 2011. Synthesis of Fe3O4 magnetic fluid used for magnetic resonance imaging and hyperthermia. J Magnet Magn Mater. 323:2953–2959.
- Wickline SA, Neubauer AM, Winter PM, Caruthers SD, Lanza GM. 2007. Molecular imaging and therapy of atherosclerosis with targeted nanoparticles. J Magn Reson Imaging. 25:667–680.
- Wu W, He Q, Jiang C. 2008. Magnetic iron oxide nanoparticles: synthesis and surface functionalization strategies. Nanoscale Res Lett. 3:397–415.
- Xu C, Xu K, Gu H, Zheng R, Liu H, Zhang X, et al. 2004. Dopamine as a robust anchor to immobilize functional molecules on the iron oxide shell of magnetic nanoparticles. J Am Chem Soc. 126:9938–9939.
- Yu S, Wu G, Gu X, Wang J, Wang Y, Gao H, et al. 2013. Magnetic and pH-sensitive nanoparticles for antitumor drug delivery. Colloids Surf B Biointerfaces. 103:15–22.
- Zhang H, Pan D, Zou K, He J, Duan X. 2009. A novel core-shell structured magnetic organic-inorganic nanohybrid involving drug-intercalated layered double hydroxides coated on a magnesium ferrite core for magnetically controlled drug release. J Mater Chem. 19:3069–3077.
- Zhao D-L, Zhang H-L, Zeng X-W, Xia Q-S, Tang J-T. 2006. Inductive heat property of Fe3O4/polymer composite nanoparticles in an AC magnetic field for localized hyperthermia. Biomed Mater. 1:198–201.