Abstract
In this study, human serum albumin (HSA) was used as a protein-based material and poly (3-hydroxybutyrate) (PHB)–carboxymethyl chitosan (CMCh) as a polysaccharide-based material for the production of nanoparticles to be used as nanocarriers in cancer therapy. HSA and PHB–CMCh nanoparticles were prepared and characterized with a Zeta Sizer, Fourier transform infrared spectroscopy, scanning electron microscopy, and atomic force microscope. The effects of the pH value of the suspending medium and the amounts of crosslinker and polymer concentration on nanoparticle size and size distribution were investigated. The anticancer-agent etoposide was used as a model drug and encapsulated in nanoparticles to obtain drug release profiles. The entrapment efficiency of HSA nanoparticles was found to be greater than that of PHB–CMCh nanoparticles. To achieve “active” targeting of cancer cells, the nanoparticles were modified with concanavalin A. In the final step of the study, the interaction of nanoparticles with cancer cells was investigated in cytotoxicity and cellular uptake studies.
Introduction
Cancer is the result of cells that grow uncontrollably and invade neighboring tissues, causing illness. According to a World Health Organization report (2014), cancer is one of the most important disease causes of death. Although there are already many classical approaches such as physical, chemical, radiological, surgical, and immunological applications for cancer diagnosis and treatment, all these methods have potential side effects. In view of their potential harm to healthy organs and tissue, a new generation of treatments is required. In addition, in many clinical and preclinical applications, two or more different treatments such as chemotherapy together with radiotherapy can be applied to cancer patients instead of a single treatment. Although this combined treatment may be more effective, it may exert negative effects on healthy cells.
Nanostructured drug delivery systems such as nanocapsules, nanospheres (Tosi et al. Citation2008), nanoliposomes (Hattori et al. Citation2009), and nanomicelles (Onyuksela et al. Citation2009) can target a tumor-specific agent to a selected region, preventing damage to healthy cells. In addition to diagnosis, they permit treatment. Small dimensions and biodegradable properties of nanoparticles are the main reasons for their use. Nanoparticles can be simplified to receive in cell and provide an elective drug accumulation. Its biodegradable property removes the risk of accumulation in an organism and allows the controlled release of drugs.
The main purpose of targeting is to improve the transport of the active substance at the cellular level, improve the therapeutic index by increasing the efficacy of a drug, reduce the toxicity of the drug, ensure the long duration of the therapeutic steady-state level, improve drug solubility and stability, resolve or at least reduce toxic and immunogenic properties, and help achieve the desired level of pharmacological response in the target region without interfering with other parts of the body.
There has been a great interest in developing nanoparticles from synthetic and natural materials carrying small and large molecules for drug delivery, especially protein- and polymer-based nanoparticles. In general, after the preparation of a particle with required size and zeta potential, it is conjugated with a ligand that binds to a cell membrane receptor that is overexpressed by tumor cells. In this way, toxicity can be reduced with drug targeting and treatment efficiency can be increased by the direct delivery of the drug to the tumor site. Various encapsulation processes are used to achieve controlled delivery of an anticancer drug or gene. In this way, drug efficiency is increased and toxicity is decreased.
Proteins are biodegradable, readily metabolized, non-toxic, and biocompatible. Given their large numbers of functional groups, it is easy to modify their surfaces for targeted drug delivery applications. This property makes them suitable candidates as drug delivery vehicles. Human serum albumin (HSA), the most abundant protein in the body, is preferred for its non-toxicity and ready modifiability (Carter and Ho Citation1994, Cohen et al. Citation2013). Nanoparticles prepared from HSA have many advantages for use in cancer treatment. They are cheap and easily available. They are also non-antigenic, enzymatically degradable, and easy to prepare using promising techniques (such as coacervation), and it is possible to obtain HSA nanoparticles in a defined size range by manipulating process parameters such as the concentration of protein, pH of the protein solution, and amount of non-solvent added. To stabilize protein-derived nanoparticles, glutaraldehyde is commonly used (Das et al. Citation2005, Langer et al. Citation2008, Merodio et al. Citation2001). Also HSA was approved by FDA (Food and Drug Administration) as nanoparticles with its paclitaxel bounded form under the trade name Abraxane® in Citation2005 to treat breast cancer.
Poly (3-hydroxybutyrate) (PHB) is a member of the polyhydroxyalkanoate (PHA) family. The PHB polymer is isolated from the bacterium Alcaligenes eutrophus and has attracted much interest for various medical applications because of its biodegradation and biocompatibility (Zinn et al. Citation2001). It is resistant to hydrolytic degradation and has potential to be used as a copolymer with other polymers. On the other hand, chitosan is a preferred polymeric material because it is biodegradable and tends to chemical modification in the study of the cell targeting. It is FDA approved and many different specific molecules can be connected to nanoparticles surface with its amino, carboxyl, primary, and secondary hydroxyl groups (Dumont et al. Citation2016). However, limited solubility of chitosan in water is a problem which can be corrected with chemical modification (Anitha et al. Citation2012, Muzzarelli Citation1988). Therefore, in this study chitosan was methylated to overcome solubility problems. O-carboxymethyl chitosan, produced by the methylation of chitosan, was efficient for anticancer-agent loading and controlled delivery (Aiping et al. Citation2006). Here the advantages of PHB and carboxymethyl chitosan (CMCh) were combined to produce a novel polysaccharide-based biopolymer for the development of nanoparticles for cancer therapy.
In targeted cancer therapy, some targeting molecules are used for recognition, as ligands that recognize the surface receptors of cancer cells. Concanavalin A (ConA) is a tetravalent plant lectin that has anti-proliferative and anti-tumor activities against various cancer cells. It selectively and chemically binds glycoproteins on cell surfaces containing alpha-mannopyranosyl and alpha-glucopyranosyl residues (Senear and Teller Citation1981, Tiegs et al. Citation1992). ConA is thus a suitable candidate for biomedical applications.
The purpose of the present investigation was to develop two different nanoparticles for delivering etoposide, an inducer of apoptosis, which has been used in cancer biology and clinical experiments (Rello-Varona et al. Citation2006). An HSA nanoparticle was prepared as a protein-based nanoparticle by desolvation and a PHB–CMCh nanoparticle was formed with a blend of natural polymers. Each type of nanoparticle was modified with ConA and loaded with etoposide. At the end of the experiments, activities of these two different nanoparticles in the MCF-7 breast cancer cell line were investigated and compared with respect to psychochemical properties and efficiency.
Materials and methods
Materials
Albumin (human, Applichem, Almanya), PHB [average molecular weight (Mw) 70,000–90,000 g/mol], glutaraldehyde, polyvinyl alcohol (PVA) [average Mw 30,000–90,000 g/mol], ConA (Canavalia ensiformis, type IV), etoposide, MES (2-(N-morpholino) ethanesulfonic acid) buffer, 1-ethyl-3-(3′-dimethylaminopropyl) carbodiimide HCl (EDC), phosphate buffered saline (PBS, pH 7.4), 3-(4,5-dimethyldiazol-2-yl)-2,5 diphenyltetrazolium bromide (MTT), growth medium (Dulbecco’s modified Eagle’s medium (DMEM F-12) supplemented with 10% fetal calf serum (FCS), 1% penicillin–streptomycin), and trypsin-EDTA were purchased from Sigma-Aldrich (St. Louis, MO). Acetone, chloroform, and glycine were purchased from Merck (Darmstadt, Germany). MCF-7 cell line was provided by Gulhane Military Medical Academy Cancer Research Center in Turkey. All reagents were of analytical grade and were used as received without further purification.
Preparation of albumin nanoparticles
HSA nanoparticles were prepared by desolvation as described previously with minimal modification (Lin et al. Citation1993). In a typical procedure, 50 mg of albumin was dissolved in 1-M 1-mL NaCl solution and pH was adjusted to 8.5. Acetone (3 mL) was added dropwisely into the albumin solution under constant stirring at 550 rpm. Glutaraldehyde (8%) was then added to achieve nanoparticle crosslinking. The crosslinking process was performed for 24 h at room temperature with constant stirring at 600 rpm (). Finally, the resulting suspension was centrifuged at 12,000 rpm for 30 min and nanoparticles were washed three times with distilled water to remove excess acetone and glutaraldehyde. The resulting nanoparticles were resuspended in 1 mL of distilled water for freeze-drying.
Synthesis of O-CMCh
O-CMCh was synthesized as reported previously by Ernest (Ernest Citation1986), low-molecular-weight chitosan (2.0 g) was suspended in 20 mL of isopropyl alcohol and stirred with a mechanical stirrer for 5 min at room temperature. NaOH solution (10 M, 5 mL) was added dropwisely to the solution, followed by mechanical stirring for 45 min at 24,000 rpm. The solution was then heated to 60 °C in an ultrasonic bath and 2.4 g of trichloroacetic acid was added. Stirring was continued for 4 h at room temperature. Finally, acetic acid was added to neutralize the pH of the reaction mixture. The mixture was filtered through filter paper and then washed with 30 mL of 30% methanol solution for 30 min. Finally, synthesized CMCh was vacuum-dried in the oven ().
Preparation of PHB–CMCh nanoparticles
PHB–CMCh nanoparticles were prepared by the double emulsion technique of Kumar et al. (Citation2004). In a typical procedure, 25 mg of PHB was dissolved in 10-mL chloroform to obtain the organic phase. The organic phase was then added to a solution containing 0.25% (w/v) PVA as stabilizing agent and 0.1% (w/v) CMCh under nitrogen gas. The resulting emulsion was stirred and dispersed with a homogenizer (Ultra-Turrax IKA T18, Germany) at 24,000 rpm for 15 min. To remove the organic solvent, the suspension was then stirred at a constant 600 rpm with a magnetic stirrer at room temperature for 18 h. As the last step, nanoparticles were recovered by centrifugation at 12,000 rpm for 30 min, washed three times with distilled water, and freeze-dried ().
Characterization of nanoparticles
The morphology and size of the HSA and PHB–CMCh nanoparticles were investigated using a scanning electron microscope (SEM) and atomic force microscope (AFM). SEM analysis was performed with a FEI Quanta 200 FEG (Japan) microscope operated with an electron beam energy of 10 keV. Topography images of nanoparticles were acquired in air with an AFM (Nanomagnetics Instruments, Oxford, United Kingdom) operated in the tapping mode using a silicon cantilever tip. The SEM and AFM images were evaluated for morphology and size distribution.
The average particle size distribution and zeta potential of the prepared nanoparticles were analyzed with a Zeta Sizer (Malvern Instruments, Model 3000 HSA, Malvern, England). The analysis was performed at 25 °C. For the measurements, samples were diluted with deionized water and subjugated to sonication for 2 min before analysis to avoid aggregation. The zeta potential of nanoparticles was determined in distilled water. FTIR studies were performed to identify the chemical structure of HSA, HSA nanoparticles, PHB and CMCh polymers, and PHB–CMCh nanoparticles (Shimadzu, DR8101, Kyoto, Japan). They were also performed for evaluation of drug encapsulation and ligand binding to the nanoparticle surface.
Etoposide loading and in vitro release studies
Etoposide loading onto HSA nanoparticles
To prepare etoposide-loaded HSA nanoparticles, a 50-mg/mL albumin was suspended in deionized water. HSA/etoposide blends were prepared in various ratios: 1/1, 1/0.5, and 1/0.25 mg, dissolved in 3 mL of acetone, and added dropwise to the albumin solution. Glutaraldehyde crosslinked and drug-loaded nanoparticles were stirred for 4 h with a magnetic stirrer at room temperature. Finally, the resulting nanoparticles were washed three times with distilled water and freeze-dried.
Etoposide loading onto PHB–CMCh nanoparticles
For drug loading onto PHB–CMCh nanoparticles, 1/1, 1/0.5, and 1/0.25 (mg/mg) proportions of the polymer/etoposide were added to PHB-chloroform solution to obtain a homogeneous mixture. Applying the synthesis procedure (Kumar et al. Citation2004), etoposide-loaded nanoparticles were yielded.
Etoposide-loaded nanoparticles were centrifuged for 30 min at 14,000 rpm. After centrifugation, the etoposide amount in the supernatant was determined by spectrophotometer (Thermo Scientific, Waltham, MA) at 280 nm.
Encapsulation of drug activity (EE %) was calculated by EquationEquation (1)(1) in which A denotes the total amount of drug used for the preparation of nanoparticles and B the amount of unloaded drug.
(1)
In order to determine the amount of active agent released from the resulting etoposide-loaded HSA and PHB–CMCh nanoparticles, 10 mg etoposide-loaded nanoparticles were incubated in 10 mL of PBS with or without lysozyme (1 mg/mL) at pH 7.4 in a shaking water bath at 37 °C. In different time intervals (15, 30, 60 min…) nanoparticles were taken in sequence and vortexed upon addition of ethanol. Following this, the particles were precipitated at 12,000 rpm, absorbance values of dissolved etoposide in supernatant were analyzed by spectrophotometer. By using the calibration curve, the etoposide concentration in the release medium was determined.
HSA and PHB–CMCh nanoparticle model ligand (ConA) modification
Immobilization of ConA onto the PHB–CMCh nanoparticles was performed following Kavaz et al. (Citation2010).
With the help of MES buffer, nanoparticle surfaces were activated and nanoparticles were stirred with EDC to create the original active intermediate form of the amine groups of the structure.
ConA (500 mg) was dissolved in 1.0 mL of 0.1-M MES buffer (pH 5.2) (the “pre-binding” sample), mixed with 1-mL activated nanoparticle solution (50 mg/mL) and incubated at 25 °C for 24 h on a rotator. In the resulting supernatant (the “post-binding” sample), absorbance values were measured at a wavelength of 280 nm to determine the ligand-free amount. Binding efficiencies were determined by measuring the first and last (pre- and post-binding samples) concentrations of the respective ConA values within the medium using an UV spectrophotometer (Shimadzu, Japan). Binding efficiencies were estimated by EquationEquation (1)(1) , where A denotes the total amount of ConA used for binding to the nanoparticle surface and B the amount of unbound ConA.
Nanoparticles were washed once with MES buffer and to stop the reaction as a source of excess amine, 1.0-M glycine (pH 8.0) was added. Nanoparticles were stirred at room temperature for 30 min at constant speed (600 rpm) and precipitated at 12,000 rpm for 30 min via centrifugation. The precipitated nanoparticles were then washed three times with phosphate buffer and resuspended in 2.0 mL of phosphate buffer. Thus, nanoparticles modified with ConA were obtained. The resulting modified nanocarriers were stored at 4 °C for subsequent use.
In vitro cytotoxicity assay (MTT) for HSA and PHB–CMCh nanoparticles
In this study, cytotoxicity of the HSA and PHB–CMCh nanoparticles in a MCF-7 breast cancer cell line was evaluated by MTT assay. Cells were cultured in a humidified 5% CO2 incubator at 37 °C. DMEM supplemented with 10% FCS was used as a medium.
The cells were harvested after becoming confluent and 100 μL of the cell suspension (1 × 105 cells/mL) was pipetted to 96-well plates and incubated overnight. The nanoparticles were diluted (1, 10, 25, 50, and 100 μg/mL) and sterilized by passage through a 0.22-μm filter. The nanoparticles were added to cells and incubated overnight. After incubation, the nanoparticle-containing medium was removed and 200-μL culture medium containing 13-μL MTT solution (5 mg/mL, diluted with RPMI 1640 without phenol red) was added to each well. Following incubation for 4 h in the dark at 37 °C, 100-mL isopropanol–HCl was added to cells. Living cells metabolize the MTT to purple formazan crystals that are dissolved by isopropanol–HCl. The plate was read at 570 nm in a microplate reader (ASYS Biochrome, UK) to determine the percentage viability. Tests were performed in triplicate.
Particle cellular uptake studies
For the cellular uptake experiment, cells were seeded in the 96-well plate incubated with etoposide loaded nanoparticle suspension. First, cells were transferred to 96-well plate to be sure 1 × 104 cells per well. Medium was changed every day until 80% confluence was reached. The medium was then replaced with 100 μL medium with etoposide loaded nanoparticles. The plate was incubated for 0.5 and 2 h. For each type of particles, particle suspension was added to eight wells and blank wells as the positive control and negative control, respectively. At different time intervals, the suspension was removed and wells were washed three times using PBS. In the cell uptake experiment, 0.5% triton X-100 solution was added to well to break the cells. The cellular uptake efficiency of particles was determined by a microplate reader (ASYS Biochrome, UK) (Yong et al. Citation2007).
Results and discussion
In this present study, HSA and PHB–CMCh nanoparticles were prepared as a model anticancer drug carrier. HSA nanoparticles were produced by desolvation. A series of experiments were performed to examine the effects of pH value, amount of crosslinker (glutaraldehyde), and concentration of polymer on nanoparticle size. PHB–CMCh nanoparticles were also prepared by solvent evaporation. The nanoparticle size distribution was optimized by changing the stirring rate, emulsifier concentration, solvent concentration, surfactant concentration, and PHB–CMCh ratio. The optimized formulations for HSA and PHB–CMCh nanoparticles were used for drug release, targeting, and cellular interaction.
HSA nanoparticles
With the purpose of identifying morphological features of the prepared HSA nanoparticles, SEM (FEI, USA) and AFM images were investigated. SEM and AFM images of HSA nanoparticles are presented in and , respectively. In SEM and AFM images, HSA nanoparticles were mostly between the sizes of 53 and 180 nm. They showed smooth, spherical forms as well as monolayer size distribution.
Some conditions such as pH of the suspension medium and the amounts of polymer and crosslinker were changed separately to optimize the size of the HSA nanoparticles. The results are summarized in .
Table 1. Effect of the pH value, degree of crosslinking, polymer concentration on the size of HAS nanoparticles.
The pH of the suspension medium was varied between 6.5 and 9.5 to investigate the effect on particle size. During nanoparticle formation, splashed droplets caused aggregation, leading to the formation of larger-sized particles at low pH values. Because the medium has a more basic pH than the isoelectric point of HSA (5.3), the use of suspension medium led to the formation of smaller-sized particles, preventing aggregation and yielding smaller-sized particles (Kufleitner et al. Citation2010). As shown in , the optimal pH value was 8.5.
Nanoparticles were produced by varying the amount of glutaraldehyde (between 30 and 200%), used as a crosslinking agent. As shown in , different crosslinking percentages not only affected nanoparticle size but also changed the surface charge of the particles (Gulsu et al. Citation2012). After the desolvation process, addition of crosslinking agent to the medium affected primary amine groups on the surface of the albumin. Thus, with the increase in the amount of crosslinking agent, the surface charge increased in negative potential values. Glutaraldehyde, directly target through freely located lysine end groups on the albumin structure. Langer et al. (Citation2008) reported that, for 100 mg of albumin, containing 60 free lysine amino acids, 8% glutaraldehyde must be consumed, corresponding to binding of all of the free lysine residues.
To evaluate the effect of polymer concentration on particle size, 50, 100, and 200 mg/mL concentrations were tested. As the concentration of polymer in the dispersion medium was increased, viscosity increased, causing the shear effect so that the deformation of the fluid resulted in larger nanoparticle in size (Jayakumar et al. Citation2010). For the formation of HSA nanoparticles, a standard albumin concentration was determined to be 50 mg/mL.
As optimum conditions for HSA nanoparticles, pH of suspension medium, amount of polymer, and degree of crosslinking were found to be 8.5, 50 mg/mL, and 60%, respectively. For each step, all other parameters except the variable group were kept constant.
Synthesis and characterization of CMCh
FTIR spectra of synthesized CMCh, PHB polymer, and PHB–CMCh nanoparticles were acquired for characterization and comparison with the literature.
Infrared spectra of the CMCh, PHB, and PHB–CMCh nanoparticles are presented in . The spectrum of CMCh showed an absorption peak at 1417 cm−1, which was assigned to the CH2 bending attached to the carbonyl group and a peak at 1595 cm−1 attributed to the asymmetric stretching vibration of –COO–. In the spectrum of PHB, a strong absorption peak at 1720 cm−1 was assigned to the symmetric stretching vibration of C=O and the peaks at 1454 cm-1 and 1380 cm-1 to CH3 bending. The spectrum of PHB–CMCh also showed these peaks, indicating that the fabricated nanoparticle was composed of PHB and CMCh (Anitha et al. Citation2009).
The PHB–CMCh nanoparticle spectra displayed the functional groups found in pure CMCh and neat PHB. This observation indicated that the two moieties have no chemical reaction, but form a blend (Nanda et al. Citation2011).
PHB–CMCh nanoparticles
SEM and AFM images were used to determine the morphological properties of the prepared PHB–CMCh nanoparticles. These images are shown in . Nanoparticles with narrow size distribution and fine spherical shape were obtained.
Figure 4. (A) SEM image of the PHB–CMCh nanoparticles and (B) 2D AFM image of the PHB–CMCh nanoparticles.
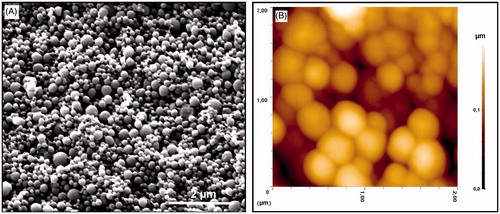
For optimizing the preparation procedure for PHB–CMCh nanoparticles, many different formulation parameters were investigated. Of these, the effect of the PHB–CMCh ratio, homogenization speed, and the amount of PVA for the emulsification process on the physicochemical characteristics of the resulting nanospheres have been studied. Data from the Zeta Sizer including the z-average size and polydispersity index (PDI) are summarized in .
Table 2. Effect of the concentration of surfactant, polymer and stirring rate on the size, zeta potential, and polydispersity of PHB–CMCh nanoparticles.
First, homogenizer speed was set to 15,500–20,000–24,000 rpm. The higher the homogenizer speed, the lower was the size of the nanoparticles observed. Reduction of the stirring rate, which reduces the amount of energy transferred to the suspension medium, led to particles of larger size. In agreement with our findings, Keck et al. reported that smaller particles were obtained as homogenization speed was increased. Enhancing shear during the emulsification process led to the formation of small nanoparticles. High speeds of homogenization produced large, uniform, and dispersible nanoparticles (Keck and Muller Citation2006). Moreover, Leroux et al. reported that the final size of prepared nanoparticles was related to the size of droplets transferred to the suspension medium. High stirring speed dispersed droplets of the polymer solution, leading to smaller particles (Leroux et al. Citation1996).
The emulsifying agent is an important parameter in the emulsification–solvent evaporation method. According to the literature, the amount of emulsifier is one of the important factors affecting final nanoparticle size: the higher the emulsifier amount, the lower is the nanoparticle size (Schork et al. Citation2005). Studies of Chen, Errico, and Mukerjee have shown the essentiality of PVA as an emulsifier which provides the needed amount of surface tension between the aqueous and organic phase, leading to stable nanoparticles (Chen and Davis Citation2002, Errico et al. Citation2009, Mukerjee and Vishwanatha Citation2009).
With the aim of demonstrating the effect of the emulsifier on the particle size and size distribution, three different amounts of PVA (100, 500, and 1000 mg) were used in our study. The concentration of PVA markedly changed the size and zeta potential of the nanoparticles (). The smallest nanoparticles (152.6 ± 10.1) with narrow size distribution (PDI =0.019 ± 0.011) were found at 100 mg, the value accepted as the optimum amount for PVA. When the amount of PVA in the aqueous phase was 1000 mg, the greatest nanoparticle size was observed. Stabilizing the droplets and nanoparticles during the fabrication of nanoparticles prevented stack formation, ensuring the formation of small particles (Kwon et al. Citation2001). Similar results were found using Span as an emulsifier (Medeiros et al. Citation2010, Saberi et al. Citation2013). In another study performed by Cetin et al. (Citation2010) using PVA as emulsifier, diclofenac-loaded PLGA nanoparticles were produced stably with a high entrapment ratio. In the same manner, high amounts of PVA retained drug entrapment owing to the thick layer surrounding the nanoparticle (Sanjeev et al. Citation2015). In a study of Cooper et al., diclofenac-loaded PLGA nanoparticles were synthesized with very low amounts of PVA and DMAB stabilizers (Dustin and Harirforoosh Citation2014). All of the above studies using PVA gave results consistent with ours and showed the importance of PVA in constructing stable and small nanoparticles.
PHB:CMCh ratios of 0.3, 0.5, 0.8, 1.25, and 2.5 were investigated as another effective parameter. Different ratios of PHB–CMCh had marked effects on the sizes of nanoparticles.
The amount of the polymer concentration in the organic phase is a critical factor for particle size. With all other variables fixed, as the polymer concentration increased, viscosity increased, inhibiting shear effects, making polymer scattering difficult in dispersion medium, and resulting in increased particle size (Schlicher et al. Citation1997). Also, with increasing PHB:CMCh ratios, both the average diameter and polydispersity of the particles increased. In addition, lower polymer concentrations resulted in uniform particle size distributions. The size and surface charge of nanoparticles play an indispensable role in transport and absorption into the body. According to Wilhelm et al., negatively charged particles adsorbed to positive sites on the cell surface while being repelled by the negative side of the cell membrane, causing the neutralization of the cell membrane (Wilhelm et al. Citation2003). Anionic nature of the polymer brings in negative charge to nanoparticles that could control the particle stability where zeta potential values were found in the range of −26.4 mV to −30.1 mV for PHB–CMCh nanoparticles.
Characterization of etoposide entrapment
Drug loading efficiency was determined by the quantity of etoposide dissolved in the aqueous phase. Released etoposide absorbance values from nanoparticles were measured at 280 nm. In initial, if the amount of active agent was much then the quantity of loaded drug was increased. Furthermore, as the active agent was decreased, the entrapment efficiency increased. The reason for this result is that in the preparation stages of the nanoparticles, concentration density occurred between the polymer matrix and the outer aqueous phase. Thus, when the initial etoposide quantity was increased, some of the drug was not encapsulated and was left in the aqueous phase, reducing the entrapment ratio (Yun Lu et al. Citation2010). The FTIR spectra of etoposide-loaded HSA and PHB–CMCh nanoparticles were acquired (data not shown).
In vitro etoposide release study
Etoposide was encapsulated into the HSA and PHB–CMCh nanoparticles at different polymer/drug ratios of 1/1, 1/0.5, and 1/0.25 (w/w). For determining the etoposide loading capacity and the amount of drug released from the nanoparticles, the absorbance values were recorded using a spectrophotometer at 280 nm for samples taken at different time intervals. The released amount of drug was estimated from a derived calibration curve, the absorbance values, and the entrapment efficiencies.
Etoposide was successfully encapsulated physically within the nanoparticles, owing to its hydrophobic character. The entrapment ratio (%) of HSA nanoparticles was greater than that of the PHB–CMCh nanoparticles. The drug ratio of HSA nanoparticles for a 1/1 polymer/etoposide ratio was 52.6%, that for 1/0.5 was 63.7%, and that for 1/0.25 was 80%, whereas the ratios of PHB–CMCh were 17.3, 27.2, and 30.6%, respectively. As the amount of active agent added to the polymer solution for entrapment decreased, the nanoparticle loading ratio increased. This result was observed because of the concentration density difference between polymer matrix and the outer active agent, meaning that while active agent concentration increased in the production phase, more active agent was lost and the entrapping efficiency of the nanoparticles decreased (Yun Lu et al. Citation2010).
Initially, the amount of etoposide added to the polymer solution was increased, while the encapsulation efficiency decreased. Etoposide loaded in a 1/1 ratio diffused out through the supernatant during the washing process, reducing the entrapment efficiency. As a result, when we compared the initial amounts of etoposide loaded into the nanoparticles, the 1/1 ratio seemed to be lower than other ratios, but because in fact a higher drug concentration was used, the loading efficacy was higher (Ha et al. Citation2011).
The drug is being released in controlled manner and finally the release becomes sustained. From the drug-release curve it is clear that at 12 h there was a 44 and 38% etoposide release from HSA and PHB–CMCh nanoparticles, respectively, without lysozyme and it was 53 and 43% etoposide release with lysozyme ( and ). In the presence of lysozyme, release of etoposide was enhanced which resulted in a sustained release of etoposide and 62% and 44% etoposide was released after 2 days from HSA and PHB–CMCh nanoparticles, respectively. Lysozyme acts on chitosan and its derivatives by hydrolyzing glucosamine–glucosamine linkages and also it acts on albumin by disulfide bond thus, by means of lysozyme, drug release takes place by diffusion after enzymatic degradation of the polymer matrix of nanoparticles (Kean and Thanou Citation2010, Kim et al Citation2006, Yang et al Citation2015).
To compare the drug release kinetics of PHB–CMCh nanoparticles with those of HSA nanoparticles, the same amounts of active agent were loaded. The released amount of etoposide per unit time from nanoparticles changed according the different loaded ratios. According to the plot, the 1/1 ratio nanoparticles contained more drug than those with other ratios, and also showed higher released amounts. However, the release rate showed an inverse pattern. The HSA nanoparticles showed higher entrapment efficiency than the PHB–CMCh nanoparticles and had much higher amounts of released drug from the nanoparticles per unit time.
ConA loading capacity
The nanoparticle loading capacity of ConA was determined with a spectrophotometer from differences between pre-sampling and post-sampling absorbance at 280 nm. The ConA loading capacity for HSA nanoparticles was 73%, whereas that for PHB–CMCh nanoparticles was 65%.
ConA antibody was covalently bound to the amino groups on the HSA and PHB–CMCh nanoparticles surface using EDC and NHS. On the unconjugated HSA nanoparticle surface (for 156 ± 25 nm particle diameters), 499 ± 17 mol/g amino groups are present (Ulbrich et al. Citation2011). On the non-conjugated PHB–CMCh nanoparticles, the surface had fewer amino groups. For this reason, the ConA loading capacity for HSA nanoparticles is higher than that for PHB–CMCh nanoparticles.
To evaluate the results, FTIR spectra of ConA were acquired. ConA was attached to HSA and PHB–CMCh nanoparticles via carboxyl (–COOH) groups on albumin and CMCh polymers. Here the nanoparticles were acting as a glycoenzyme. Covalent binding occurred between carboxyl (–COOH) termini of the nanoparticles and the amine (–NH2) terminus of ConA.
In vitro cytotoxicity
The cytotoxic effects of the HSA nanoparticles, ConA-conjugated HSA nanoparticles, etoposide-loaded HSA nanoparticles, and etoposide-loaded and ConA conjugated HSA nanoparticles were evaluated in the MCF-7 cell line by MTT assay at several concentrations (1, 10, 25, 50, and 100 μg/mL). Nanoparticles were incubated with MCF-7 cells for 24 h and untreated cells were used as negative control.
The HSA nanoparticles showed no marked toxicity, but the etoposide-loaded and ConA-conjugated HSA nanoparticles showed highly toxic effects on the MCF-7 cells. Cell viability was calculated as 61.1 ± 3.2% for 100-μg/mL of the etoposide-loaded and ConA-conjugated HSA nanoparticles, and 101.8 ± 5.1 for the HSA nanoparticles. Cytotoxicity of the etoposide-loaded and ConA-conjugated HSA nanoparticles increased with the nanoparticle concentration. Cell viability was calculated as 73.8 ± 5.1% for 1 μg/mL and 61.1 ± 3.2% for 100 μg/mL ().
Figure 7. Cytotoxicity of different formulation of HSA nanoparticles (n = 3). The nanoparticles were incubated with MCF-7 cells for 24 h. After incubation, cell viability was measured by MTT assay. Data are expressed as percent of control mean ± SD of three independent experiments.
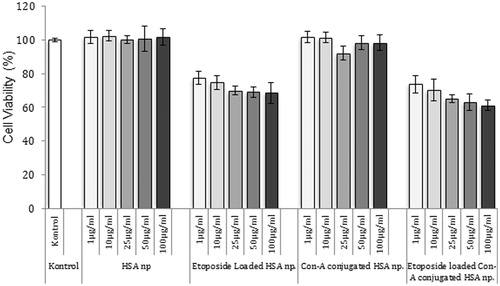
Also, it was assumed that drug entrapment efficiency was 50% for nanoparticles, therefore to compare free drug with loaded amount, the free drug concentration was calculated as 0.5, 5, 15, 25, and 50 μg/mL and cell viability was calculated as 73.2 ± 2.7%, 70 ± 2.4%, 66 ± 1.1%, 64 ± 3.3%, and 57.3 ± 2.1%, respectively, for free etoposide which shows higher toxicity than etoposide loaded nanoparticles as expected.
Mo et al. prepared HSA nanoparticles with a mean diameter of 120 nm as a nonviral vector for ocular delivery. They assessed the cytotoxic effects of the nanoparticles by MTT assay on the ARPE 19 cell line. According to the viability studies, more than 90% of the ARPE 19 cells were alive after 96 h of incubation at concentrations up to 5 mg/mL (Mo et al. Citation2007).
In another study, sustained and controlled release of 2-meth-oxyestradiol (2-ME) in folic acid-modified BSA nanoparticles provided treatment efficacy against cancer cells with very low cytotoxicity (Zhang et al. Citation2014).
PHB–CMCh nanoparticles were used with the same modification and concentration as HSA nanoparticles. PHB–CMCh, ConA-conjugated PHB–CMCh, etoposide-loaded PHB–CMCh, and etoposide-loaded and ConA-conjugated PHB–CMCh nanoparticles were prepared at several concentrations (1, 10, 25, 50, and 100 μg/mL).
Etoposide-loaded and ConA-conjugated PHB–CMCh nanoparticles showed high cytotoxicity effect on the MCF-7 cells. Cell viability was 93.3 ± 8.3% for PHB/CMCh, 82.6 ± 2.2% for ConA conjugated PHB–CMCh, 79.2 ± 5.2% for etoposide-loaded PHB–CMCh, and 68.2 ± 4.5% for etoposide-loaded and ConA-conjugated PHB–CMCh nanoparticles at a concentration of 100 μg/mL. Both HSA and PHB–CMCh nanoparticles exerted toxic effects on cancer cells with overexpressed ConA receptors and etoposide (). In a study, Erdal et al. prepared ConA-attached etoposide-loaded PHB nanoparticles for cancer therapy and showed that increasing ConA-attached nanoparticle concentrations led to very little cytotoxicity (Erdal et al. Citation2012).
Figure 8. Cytotoxicity of different formulation of PHB–CMCh nanoparticles (n = 3). The nanoparticles were incubated with MCF-7 cells for 24 h. After incubation, cell viability was measured by MTT assay. Data are expressed as percent of control mean ± SD of three independent experiments.
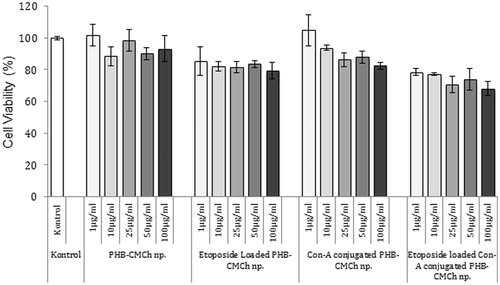
Kilicay et al. prepared etoposide-loaded and folic acid-conjugated PHBHHX nanoparticles and assessed the cytotoxic effects of the nanoparticles by MTT assay in the HeLa cell line. Etoposide-loaded and folic acid-conjugated PHBHHX nanoparticles were more toxic than PHBHHX nanoparticles. Although more than 90% of the cells were alive at concentrations up to 5 mg/mL, PHBHHX nanoparticles having a 1/0.5 polymer/drug ratio gave 44.2 ± 4.68% cytotoxicity in HeLa cells. In the same manner, our study showed results coherent with those from the literature (Kilicay et al. Citation2011).
Cellular uptake of nanoparticles
It is clear that the cellular uptake efficiency of drug-loaded nanoparticles affects the therapeutic effects. Etoposide was incorporated into HSA and PHB–CMCh nanoparticles and the corresponding cellular uptake efficiency was evaluated by microplate reader. shows MCF-7 cells after 0.5 h and 2 h incubation with etoposide loaded nanoparticles at 37 °C. It was evident that many more particles were engulfed by the cells after 2 h incubation than 0.5 h because of the extended exposure time. Particle cellular uptake could be affected by many factors, such as particle size (Zauner et al. Citation2001) different cell lines, different compositions of the particles, and surface properties (surface hydrophobic/hydrophilic balance, zeta potential) (Jung et al. Citation2000). The attachment of the particles to the cell membrane is the first step of the particle uptake process by the cell and this step seems to be mostly affected by the surface charge of the particles (Lorenza et al. Citation2006). In our experiment, the zeta potential of the both HSA and PHB–CMCh nanoparticles are nearly the same and therefore nanoparticle size is considered to be the key character to determine the cellular uptake efficiency. Because HSA nanoparticles are much smaller than PHB–CMCh nanoparticles this has enabled more of the HSA nanoparticles taken into the cells.
Conclusion
Etoposide-loaded ConA-attached HSA and PHB–CMCh nanoparticles were prepared for targeted cancer therapy. According to the results, the size of the HSA nanocarriers was less than that of the PHB–CMCh nanoparticles and also their structures were more spherical and monodisperse. The anticancer-agent etoposide was used as a model drug and encapsulated in nanoparticles to obtain drug release profiles. The entrapment efficiency of the HSA nanoparticles was found to be greater than that of the PHB–CMCh nanoparticles. To achieve “active” targeting of cancer cells, the nanoparticles were modified with ConA. MCF-7 cells prefer smaller nanoparticles if these nanoparticles have similar structure. Furthermore, preliminary investigations show that the particle size and zeta potential have great effect on the cellular uptake efficiency. Cytotoxicity and cellular uptake studies showed that ConA-attached etoposide-loaded HSA nanoparticles were highly effective against cancerous cells. Both drug nanoparticle systems were found to be effective therapeutic agents and are promising for targeted cancer therapy.
Disclosure statement
The authors report no conflicts of interest. The authors alone are responsible for the content and writing of this article.
References
- Abraxane®. 2005. Abraxane Package Insert. Available from: http://www.fda.gov/ohrms/dockets/ac/06/briefing/2006-4235B2-01-01AbraxisBioscience-background.pdf.
- Aiping Z, Jianhong L, Wenhul Y. 2006. Effective loading and controlled release of camptothecin by O-carboxymethylchitosan aggregates. Carbohydr Polym. 63:89–96.
- Anitha A, Maya S, Deepa N, Chennazhi KP, Nair SV, Jayakumar R. 2012. Curcumin-loaded N,O-carboxymethyl chitosan nanoparticles for cancer drug delivery. J Biomater Sci Polym Ed. 23:1381–1400.
- Anitha A, Rani VVD, Krishna R, Sreeja V, Selvamurugan N, Nair SV, Tamura H, Jayakumar R. 2009. Synthesis, characterization, cytotoxicity and antibacterial studies of chitosan, O-carboxymethyl and N, O-carboxymethyl chitosan nanoparticles. Carbohydr Polym. 78:672–667.
- Carter DC, Ho JX. 1994. Structure of serum-albumin. Adv Protein Chem. 45:153–203.
- Cetin M, Atila A, Kadioglu Y. 2010. Formulation and in vitro characterization of Eudragit® L100 and Eudragit® L100-PLGA nanoparticles containing diclofenac sodium. AAPS PharmSciTech. 11:1250–1256.
- Chen J, Davis SS. 2002. The release of diazepam from poly(hydroxybutyrate-hydroxyvalerate) microspheres. J Microencapsul. 19:191–201.
- Cohen S, Pellach M, Kam Y, Grinberg I, Corem-Salkmon E, Rubinstein A, Margel S. 2013. Synthesis and characterization of near IR fluorescent albumin nanoparticles for optical detection of colon cancer. Mater Sci Eng C Mater Biol Appl. 33:923–931.
- Das S, Banerjee R, Bellare J. 2005. Aspirin loaded albumin nanoparticles by coacervation: implications in drug delivery. Trends Biomater Artif Organs. 18:203–212.
- Dumont VC, Mansur AAP, Carvalho SM, Borsagli FGLM, Pereira MM, Mansur HS. 2016. Chitosan and carboxymethyl-chitosan capping ligands: effects on the nucleation and growth of hydroxyapatite nanoparticles for producing biocomposite membranes. Mater Sci Eng C. 59:265–277.
- Dustin LC, Harirforoosh S. 2014. Design and optimization of PLGA-based diclofenac loaded nanoparticles. PLoS One. 9:e87326.
- Erdal E, Kavaz D, Sam M, Demirbilek M, Demirbilek ME, Saglam N, Denkbas EB. 2012. Preparation and characterization of magnetically responsive bacterial polyester based nanospheres for cancer therapy. J Biomed Nanotechnol. 8:1–9.
- Ernest HR. N, O-carboxymethyl chitosan and preparative method therefore, United States Patents. US4619995, Oct 28, 1986.
- Errico C, Bartoli C, Chiellini F, Chiellini E. 2009. Poly(hydroxyalkanoates)-based polymeric nanoparticles for drug delivery. J Biomed Biotech. 2009:571702.
- Gulsu A, Ayhan H, Ayhan F. 2012. Preparation and characterization of ketoprofen loaded albumin microspheres. Turk J Biochem. 37:120–128.
- Ha W, Wu H, Wang XL, Peng SL, Ding LS, Zhang S, Li BJ. 2011. Self-aggregates of cholesterol-modified carboxymethyl konjac glucomannan conjugate: preparation, characterization, and preliminary assessment as a carrier of etoposide. Carbohydr Polym. 86:513–519.
- Hattori Y, Shi L, Ding W, Koga K, Kawano K, Hakoshima M, Maitani Y. 2009. Novel irinotecan-loaded liposome using phytic acid with high therapeutic efficacy for colon tumors. J Control Release. 136:30–37.
- Jayakumar R, Prabaharan M, Nair SV, Tokura S, Tamura H, Selvamurugan N. 2010. Novel carboxymethyl derivatives of chitin and chitosan materials and their biomedical applications. Prog Mater Sci. 55:675–709.
- Jung T, Kamm W, Breitenbach A, Kaiserling E, Xiao JX, Kissel T. 2000. Biodegradable nanoparticles for oral delivery of peptides: is there a role for polymers to affect mucosal uptake? Eur J Pharm Biopharm. 50:147–160.
- Kavaz D, Odabas S, Guven E, Demirbilek M, Denkbas EB. 2010. Bleomycin loaded magnetic chitosan nanoparticles as multifunctional nanocarriers. J Bioact Compat Polym. 25:305–318.
- Kean T, Thanou M. 2010. Biodegradation, biodistribution and toxicity of chitosan. Adv Drug Deliv Rev. 62:3–11.
- Keck CM, Muller RH. 2006. Drug nanocrystals of poorly soluble drugs produced by high pressure homogenisation. Eur J Pharm Biopharm. 62:3–16.
- Kilicay E, Demirbilek M, Turk M, Guven E, Hazer B, Denkbas EB. 2011. Preparation and characterization of poly(3-hydroxybutyrate-co-3-hydroxyhexanoate) (PHBHHX) based nanoparticles for targeted cancer therapy. Eur J Pharm Sci. 44:310–320.
- Kim DG, Jeong YI, Choi C, Roh SH, Kang SK, Jang MK, Nah JW. 2006. Retinol-encapsulated low molecular water-soluble chitosan nanoparticles. Int J Pharm. 319:130–138.
- Kufleitner J, Worek F, Kreuter J. 2010. Incorporation of obidoxime into human serum albumin nanoparticles: optimisation of preparation parameters for the development of a stable formulation. J Microencapsul. 27:594–601.
- Kumar MNVR, Bakowsky U, Lehr CM. 2004. Preparation and characterization of cationic PLGA nanospheres as DNA carriers. Biomaterials. 25:1771–1777.
- Kwon HY, Lee JY, Choi SW, Jang Y, Kim JH. 2001. Preparation of PLGA nanoparticles containing estrogen by emulsification-diffusion method. Colloids Surf A: Physicochem Eng Aspects. 182:123–130.
- Langer K, Anhorn MG, Steinhauser I, Dreis S, Celebi D, Schrickel N, Faust S, Vogel V. 2008. Human serum albumin (HSA) nanoparticles: reproducibility of preparation process and kinetics of enzymatic degradation. Int J Pharm. 347:109–113.
- Leroux JC, Allemann E, Jaeghere FD, Doelker E, Gurny R. 1996. Biodegradable nanoparticles from sustained release formulations to improved site specific drug delivery. J Control Release. 39:339–350.
- Lin W, Coombes A, Davies M, Davis S, Illum L. 1993. Preparation of sub-100 nm human serum albumin nanospheres using a pH-coacervation method. J Drug Target. 1:237–243.
- Lorenza MR, Holzapfel V, Musyanovych A, Nothelfer K, Walther P, Frank H, et al. 2006. Uptake of functionalized, fluorescent-labeled polymeric particles in different cell lines and stem cells. Biomaterials. 27:2820–2828.
- Medeiros SF, Santos AM, Fessi H, Elaissari A. 2010. Synthesis of biocompatible and thermally sensitive poly(N-vinylcaprolactam) nanogels via inverse miniemulsion polymerization: effect of the surfactant concentration. J Polym Sci Pol Chem. 48:3932–3941.
- Merodio M, Arnedo A, Renedo MJ, Irache JM. 2001. Ganciclovir-loaded albumin nanoparticles: characterization and in vitro release properties. Eur J Pharm Sci. 12:251–259.
- Mo Y, Barnett ME, Takemoto D, Davidson H, Kompella UB. 2007. Human serum albumin nanoparticles for efficient delivery of Cu, Zn superoxide dismutase gene. Mol Vis. 13:746–757.
- Mukerjee A, Vishwanatha JK. 2009. Formulation, characterization and evaluation of curcumin-loaded PLGA nanospheres for cancer therapy. Anticancer Res. 29:3867–3876.
- Muzzarelli RAA. 1988. Carboxymethylated chitin and chitosans. Carbohydr Polym. 8:1–21.
- Nanda R, Sasmal A, Nayak PL. 2011. Preparation and characterization of chitosan-polylactide composites blended with Cloisite 30B for control release of the anticancer drug paclitaxel. Carbohydr Polym. 83:988–994.
- Onyuksela H, Mohantya PS, Rubinstein I. 2009. VIP-grafted sterically stabilized phospholipid nanomicellar 17-allylamino-17-demethoxy geldanamycin: a novel targeted nanomedicine for breast cancer. Int J Pharm. 365:157–161.
- Rello-Varona S, Gamez A, Moreno V, Stockert JC, Cristobal J, Pacheco M, et al. 2006. Metaphase arrest and cell death induced by etoposide on HeLa cells. Int J Biochem Cell Biol. 38:2183–2195.
- Saberi AH, Fang Y, McClements DJ. 2013. Fabrication of vitamin E-enriched nanoemulsions: factors affecting particle size using spontaneous emulsification. J Colloid Interface Sci. 391:95–102.
- Sanjeev KP, Ghosh S, Maiti P, Haldar C. 2015. Therapeutic efficacy and toxicity of tamoxifen loaded PLA nanoparticles for breast cancer. Int J Biol Macromol. 72:309–319.
- Schlicher EJAM, Postma NS, Zuidema J, Talsma H, Hennink WE. 1997. Preparation and characterisation of poly (D, L-lactic-co-glycolic acid) microspheres containing desferrioxamine. Int J Pharm. 153:235–245.
- Schork FJ, Luo YW, Smulders W, Russum JP, Butte A, Fontenot K. 2005. Miniemulsion polymerization. Adv Polym Sci. 175:129–255.
- Senear DF, Teller DC. 1981. Thermodynamics of concanavalin A dimer-tetramer self association: sedimentation equilibrium studies. Biochemistry. 20:3076–3083.
- Tiegs G, Hentschel J, Wendel AAT. 1992. A T cell-dependent experimental liver injury in mice inducible by concanavalin A. J Clin Invest. 90:196–203.
- Tosi G, Costantino L, Ruozi B, Forni F, Vandelli MA. 2008. Polymeric nanoparticles for the drug delivery to the central nervous system. Expert Opin Drug Deliv. 5:155–174.
- Ulbrich K, Michaelis M, Rothweiler F, Knobloch T, Sithisar P, Cinatl J, Kreuter J. 2011. Interaction of folate-conjugated human serum albumin (HSA) nanoparticles with tumour cells. Int J Pharm. 406:128–134.
- Wilhelm C, Billotey C, Roger J, Pons JN, Bacri JC, Gazeau F. 2003. Intracellular uptake of anionic superparamagnetic nanoparticles as a function of their surface coating. Biomaterials. 24:1001–1011.
- Yang M, Dutta C, Tiwari A. 2015. Disulfide-bond scrambling promotes amorphous aggregates in lysozyme and bovine serum albumin. J Phys Chem B. 119:3969–3981.
- Yong H, Jingwei X, Yen WT, Chi-Hwa W. 2007. Effect of PEG conformation and particle size on the cellular uptake efficiency of nanoparticles with the HepG2 cells. J Control Release. 118:7–17.
- Yun Lu X, Zhang Y, Wang L. 2010. Preparation and in vitro drug-release behavior of 5-fluorouracil-loaded poly(hydroxybutyrate-co-hydroxyhexanoate) nanoparticles and microparticles. J Appl Polym Sci. 116:2944–2950.
- Zauner W, Farrow NA, Hainess AMR. 2001. In vitro uptake of polystyrene microspheres: effect of particle size, cell line and cell density. J Control. 71:39–51.
- Zhang N, Xia Y, Guo X, Wang P, Yan S, Lu C, Cao D, Zhang Z. 2014. Preparation, characterization, and in vitro targeted delivery of folate-conjugated 2-methoxyestradiol-loaded bovine serum albumin nanoparticles. J Nanopart Res. 16:2390.
- Zinn M, Witholt B, Egli T. 2001. Occurrence, synthesis and medical application of bacterial polyhydroxyalkanoate. Adv Drug Deliv Rev. 53:5–21.