ABSTRACT
A new type of a combined ultraviolet (UV)-biofilter system for air pollution control is developed. In this paper, two conceptual mathematical submodels of the UV reactor and standalone biofilter are developed. All model parameters have been determined by independent experiments or have been taken from literature. Results from UV and the standalone biofilter submodels are in a good agreement with experimental data. However, the performance of the combined system has significantly deviated from those of the UV or standalone submodels because of the stimulating effects of UV irradiation products on the subsequent biofilter performance. A modified model that considers the stimulating effects has agreed well with experimental data over a wide range of operating conditions. Further analysis of the primary parametric sensitivity of the model has shown that inlet chlorobenzene concentrations, gas empty-bed residence time in the UV reactor, and light intensity are important operating conditions.
This study focuses on the model development of a combined UV-biofilter system to treat gaseous chlorobenzene. The results of this study are useful in understanding the effects of UV pretreatment on the performance of the subsequent biofilter. It can also help predict chlorobenzene removal performance in combined UV-biofilter systems.
INTRODUCTION
Biofiltration is widely considered a cost-effective technology because of its low operating costs and convenient maintenance.Citation1 However, biofilters can not achieve satisfactory performance in treating some recalcitrant volatile organic compounds (VOCs) given the low biodegradability of these compounds. Several efforts have been proposed to address these challenges.Citation2–4 One of the efforts comes from combination of ultraviolet (UV) photodegradation and biofiltration technology.
Previous studies have mentioned that the combination of UV photodegradation and biofiltration technologies can be successful for treating some recalcitrant gaseous contaminants. Koh et al.Citation5 and Wang et al.Citation6 have shown that UV photodegradation transformed some recalcitrant VOCs into more biodegradable products. Moussavi et al.Citation7 and Wang et al.Citation8,Citation9 produced biofilters with improved performance by incorporating ozone-producing UV lamps for pretreatment. Another study that explored the mechanisms of UV pretreatment on biofilters has shown that ozone produced by UV irradiation might be responsible for the performance enhancement of biofilters.Citation10
Combined UV-biofilter systems are complex, and detailed mathematical modeling is necessary to quantitatively describe the stimulating effects of the UV pretreatment on the subsequent biofilter and in optimizing or scaling up the combined system. In this study, a conceptual model on gaseous chlorobenzene degradation in a combined UV-biofilter system is presented and validated with experimental results. Model parametric sensitivity studies are also discussed in this paper.
MODEL DEVELOPMENT
Submodel of the UV Reactor
In the gas phase, photons are absorbed to initiate photo-chemical reaction with chlorobenzene. Quantum yield (Φ) is defined as follows (Equationeq 1)Citation11:
The removal rate constant k (sec−1) can be defined as (Equationeq 2)
On the basis of the Beer-Lambert law, the kinetic model for the photochemical reaction can be deduced asCitation11
The flow pattern inside of the UV reactor used in this study can be considered an ideal plug flow. Thus, the following equation is given:
From Equationeqs. 3 and Equation4,
Submodel of the Biofilter
In the gas phase, chlorobenzene is transported from the bulk gas stream into the water surface. The differential mass balance for chlorobenzene in the gas phase is (Equationeq 6)
At the gas-water interface, chlorobenzene concentrations in gas and liquid phases can be defined according to Henry's law equilibrium (Equationeq 7),
Because no chlorobenzene biodegradation occurs in the water phase (i.e., chlorobenzene is degraded in the biofilm), the mass transport can be described according to Fick's first law,
The chlorobenzene flux and chlorobenzene concentration profile can be expressed by truly closed-form analytical solutions. The differential mass balance in the biofilm is
A boundary condition corresponds to no flux into the attachment surface (Equationeq 10).
An analytical solution to Equationeq 9 is available when concentrations at both boundaries of the biofilm are known. Combining Equationeqs 8 and Equation9 gives
There are two simplifications for the submodel. The first is that the concentration at attachment surface approached zero (Equationeq 12).
The liquid phase is quite thin, given the application of water.Citation12 Therefore, in this study, it was reasonable to disregard water layer thickness. If such is the case, the concentrations in the gas phase and biofilm could be defined as (13)
Finally, Equationeq 11 yields (Equationeq 14)
Parameter Determination
Average light intensity (I ave) inside of the reactor is calculated by the Line Source with Spherical Emission (LSSE) model (Equationeq 15).Citation13
Other parameters in UV and biofilter submodels are shown in and , respectively.
Table 1. A list of symbols and parameters and their values for UV submodel
Table 2. A list of symbols and parameters and their values for biofilter submodel
MATERIALS AND METHODS
Experimental Setup and Operation
Combined UV-biofilter and control biofilter processes were established, as shown in .Citation12,Citation14 The UV reactor was made of stainless steel with an effective volume of 2.3 L. An ozone-producing low-pressure mercury vapor lamp was inserted into the airtight reactor. Both biofilters were identical in configuration with heights of 45 cm and internal diameters of 12 cm. The packing was made of nonbiodegradable bamboos (a depth of 30 cm), which could be easily acquired.
Experiments were conducted for 280 days under various operating conditions, including varying c A0 and gas flow rates (). Biofilters were supplied with approximately 80 mL of nutrient solution every 1 hr. Leachate from the filter bed was discharged directly without recirculation. No pH control was integrated with the experimental system. Temperature was maintained relatively constant at 20–30 °C.
Table 3. Operating conditions of the UV-biofilter system
Analytical Methods
Concentrations of chlorobenzene were analyzed by a gas chromatograph (Shimadazu, GC-14B) with an flameionization detector (FID) detector and a capillary column (Ulbon HR-1, 0.25 mm × 30 m).
The ozone content in the gas was measured using the gas detection tube method. The 100-mL sample was injected into a tube containing detecting agents at a flow rate of 50 mL min−1. The concentration of ozone was read on-site on the basis of the color change of the detecting agents (Beijing LaoBaoSuo Science and Technology Company).
RESULTS AND DISCUSSION
Comparison of UV Submodel Simulations and Experimental Results
shows the UV submodel simulation and UV reactor experimental data at different c A0 and gas flow rates. The model prediction was in good agreement with experimental data. Differences beyond the experimental error bars were observed between the model and experimental reactor at lower flow rates. However, for a model without any fitted parameter, small deviations might arise.
Figure 3. Model simulations (lines) and experimental data (symbols) of chlorobenzene removal efficiencies for (a) c A0 changes and (b) gas flow rate variations.
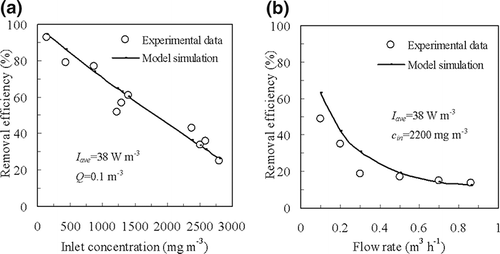
presents a comparison of the model with the experimental data obtained when the c A0 in the UV reactor varied from 100 to 2800 mg m−3 at an I ave of 38 W m−3 and a flow rate of 0.1 m3 hr−1. The model appropriately predicted the experimental data, corresponding to a linear decrease in chlorobenzene removal efficiency with increasing c A0. The model also accurately predicted removal efficiencies at various flow rates. Once again, the comparison of the model and the experimental data has revealed that the UV submodel describes the results well.
Comparison of Biofilter Submodel Simulations and Experimental Results
illustrates chlorobenzene removal efficiencies for a 280-day simulation of the standalone biofilter (no UV pretreatment). Simulation results for the removal efficiencies were consistent with experimental performance.
Figure 4. Model simulations (lines) and experimental data (symbols) of chlorobenzene removal efficiencies of the control biofilter (no UV treatment).
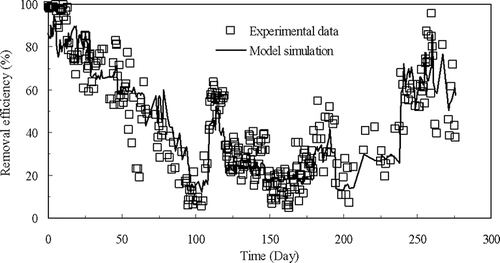
In the first 100 days of operation, the residence time inside of the standalone biofilter decreased with an increases in gas flow rate, resulting in a slow decline of removal efficiencies. The model simulation also clearly demonstrated the gradual decline in removal efficiency. On day 100 in the simulation, a removal efficiency of approximately 10% was observed. From days 180 to 194, remarkable climbing removal efficiency was observed, and this might have been caused by c A0 reduction. These findings were consistent with predicted results. In other operating conditions, the biofilter submodel yielded relatively accurate predictions of the standalone biofilter performance.
Stimulating Effects of UV Pretreatment on Subsequent Biofilter Performance
A previous study has reported that some photodegradation products (e.g., chlorophenol, phenol, and low-molecular-weight acids) and the byproduct of ozone can be produced by UV irradiation.Citation7 These products are soluble and biodegradable, resulting in low pH of the filter bed, which threatens the biofilter performance. In addition, ozone has been proven to reduce the extra-cellular polymer substance (EPS) content of biofilm in a biofilter.Citation10 Reducing EPS content can influence the mass transfer in the biofilm, resulting in a different diffusion coefficient of chlorobenzene (D f) compared with that in a control biofilter. Ozone and other photodegradation products also play key roles in the microbial metabolic activity of biofilms and changed the structure of the microbial communities.Citation10 These differences can alter some model parameters, such as the maximum substrate utilization rate and half-saturation constant.
Some parameters in the biofilter submodel of the combined UV-biofilter system were different from those of the standalone biofilter. Accordingly, significant deviations were observed between the combined UV-biofilter system experiments and predictions based on the two submodels ().
To accurately simulate the performance of the combined UV-biofilter system, a new parameter (i.e., stimulating factor [ψ]) was defined to determine the stimulating effects of the ozone on subsequent biofilter performance.Citation15
Some factors (e.g., gas flow rate or light intensity) can affect ozone concentrations at the UV outlet. A series of independent experiments was conducted to calculate the average ψ at according to Equationeq 17. The relationship between ψ and the measured ozone concentrations at the outlet of the UV reactor in these experiments was also analyzed ().
As shown in , an empirical formula similar to the Monod model was acquired.
According to and Equationeq 18, higher ozone concentrations would result in higher ψ. Further increases in ozone concentration led to a plateau, an indication of the stimulating limitation of the ozone.
Thus, the final predicted results of the combined system could be obtained as (Equationeq 19)
Model Simulation of Steady-State Performance of the Combined UV-Biofilter System
Modified model simulations (including ψ) were compared with combined model simulations (excluding ψ) and experimental results. Some parameters in the modified model and combined models were determined independently ().
shows the model simulations of outlet concentration, removal efficiencies, and removal rates and experimental data at different operating conditions. The modified model predictions were in better agreement with experimental data compared with the combined model predictions, showing the stimulating effects of UV pretreatment on subsequent biofilter performance. One explanation for the discrepancy between the two models could be that the ozone produced by UV irradiation has stimulated the performance of the biofilter.Citation10
Parametric Sensitivity
The sensitivity of the primary parameter in the combined UV-biofilter model used to control gaseous chlorobenzene was examined. To show the parametric sensitivity, the relative sensitive index (RSI) was defined as (Equationeq 20)
In this study, the sensitivity of chlorobenzene removal to the model parameters was examined using the following conditions: c A0 of 1000 mg m−3; empty-bed residence time in the UV reactor and biofilter of 27 sec (τUV) and 41 sec (τBF), respectively; and an I ave of 38 W m−3. The sensitivity of chlorobenzene removal efficiency of the above-mentioned operating parameters was studied by varying the values of operating parameters (P 0) to 1.2 times (P = 1.2P 0) of the initial values.
The final sensitivity results of c A0, τUV, τBF, and I ave were 0.34, −0.12, −0.03, and −0.15, respectively. Results showed that c A0, τUV, and I ave played important roles in the overall performance of the combined system, whereas τBF did not.
CONCLUSIONS
A conceptual mathematical model was developed for a combined UV-biofilter system to treat gaseous chlorobenzene. All model parameters were taken from literature or determined by independent experiments; thus, the model was fully predictive. Standalone UV and biofilter submodels were in a good agreement with experimental data. However, the performance of the combined model showed significant deviations from either of the two sub-models. The stimulating effects of ozone were considered, and the modified model was in good agreement with experimental data over a wide range of operating conditions. Further analysis of the primary parametric sensitivity of the model showed that c A0, τUV, and I ave were important operating conditions.
ACKNOWLEDGMENTS
This study was supported by the National Natural Science Foundation of China (Grant No. 50708049).
REFERENCES
- Kennes , C. and Veiga , M.C. 2004 . Fungal Biocatalysts in the Biofiltration of VOC-Polluted Air . J. Biotechnol. , 113 : 305 – 319 .
- Watanabe , K. , Hino , S. and Takahashi , N. 1996 . Responses of Activated Sludge to an Increase in Phenol Loading . J. Ferment. Bioeng. , 82 : 522 – 524 .
- Oliveira , T.A.C. and Livingston , A.G. 2003 . Bioscrubbing of Waste Gas Substrate Absorber to Avoid Instability Induced by Inhibition Kinetics . Biotechnol. Bioeng. , 84 : 552 – 563 .
- Mohseni , M. and Zhao , J.L. 2006 . Coupling Ultraviolet Photolysis and Biofiltration for Enhanced Degradation of Aromatic Air Pollutants . J. Chem. Technol. Biotechnol. , 81 : 146 – 151 .
- Koh , L.H. , Kuhn , D.C. , Mohseni , M. and Allen , D.G. 2004 . Utilizing Ultraviolet Photooxidation as a Pre-Treatment of Volatile Organic Compounds Upstream of a Biological Gas Cleaning Operation . J. Chem. Technol. Biotechnol. , 79 : 619 – 625 .
- Wang , C. , Xi , J.Y. and Hu , H.Y. 2008 . Chemical Identification and Acute Biotoxicity Assessment of Gaseous Chlorobenzene Photodegradation Products . Chemosphere , 73 : 1167 – 1171 .
- Moussavi , G. and Mohseni , M. 2007 . Using UV Pretreatment to Enhance Biofiltration of Mixtures of Aromatic VOCs . J. Hazard. Mater. , 144 : 59 – 66 .
- Wang , C. , Xi , J.Y. , Hu , H.Y. and Yao , Y. 2009 . Effects of UV Pretreatment on Microbial Community Structure and Metabolic Characteristics in a Subsequent Biofilter Treating Gaseous Chlorobenzene . Bioresource Technol. , 100 : 5581 – 5587 .
- Wang , C. , Xi , J.Y. , Hu , H.Y. and Yao , Y. 2009 . Advantages of Combined UV Photodegradation and Biofiltration Processes to Treat Gaseous Chlorobenzene . J. Hazard. Mater. , 171 : 1120 – 1125 .
- Wang , C. , Xi , J.Y. , Hu , H.Y. and Yao , Y. 2009 . Stimulative Effects of Ozone on a Biofilter Treating Gaseous Chlorobenzene . Environ. Sci. Technol. , 43 : 9407 – 9412 .
- Kang , X.H. and Liu , H.Q. 1995 . Principle and Application on Photochemical Reaction , 88 – 89 . Tianjing , , People's Republic of China : Tianjing University .
- Wang , C. , Xi , J.Y. and Hu , H.Y. 2008 . A Novel Integrated UV-Biofilter System to Treat High Concentration of Gaseous Chlorobenzene . Chinese Sci. Bull. , 53 : 2712 – 2716 .
- Orlando , M.A. , Roberto , L.R. and Alberto , E.C. 1986 . Radiation Field Modeling in Photoreactors . I. Homogeneous Media , 41 : 421 – 444 . Chem. Eng. Sci.
- Wang , C. , Xi , J.Y. and Hu , H.Y. 2009 . Reduction of Toxic Products and Bioaerosol Emission of a Combined UV-Biofilter Process for Chlorobenzene Treatment . Journal of the Air & Waste Management Association , 59 : 405 – 410 . doi:10.3155/1047-3289.59.4.405
- Sitanggang , A.B. , Wu , H.S. , Wang , S.S. and Ho , Y.C. 2010 . Effect of Pellet Size and Stimulating Factor on the Glucosamine Production Using Aspergillus sp. BCRC 31742 . Bioresource Technol. , 101 : 3595 – 3601 .
- Wang , S.J. 2000 . Colleague Physics , 2nd , 343 – 344 . Shanghai , , People's Republic of China : Tongji University .
- Lyman , W.J. , Rael , W.F. and Rosenblatt , D.H. 1991 . Handbook of Chemical Property Estimation Methods: Environmental Behavior of Organic Compounds (Chinese Edition) , 153 – 154 . Beijing , , People's Republic of China : Chemical Engineering .
- Rittmann , B.E. and McCarty , P.L. 2004 . Environmental Biotechnology: Principle and Application (Chinese Edition) , 8 Beijing , , People's Republic of China : Tsinghua University .
- Mackay , D. and Shiu , W.Y. 1981 . A Critical Review of Henry's Law Constants for Chemicals of Environmental Interest . J. Phys. Chem. Ref. Data. , 10 : 1175 – 1199 .
- Christos , J.M. and Basil , C.B. 1998 . An Experimental and Modeling Study on the Removal of Mono-Chlorobenzene Vapor in Biotrickling Filters . Biotech. Bioeng. , 59 : 328 – 343 .