Abstract
Aged individuals are more susceptible to infections due to a general decline in immune function broadly referred to as immune senescence. While age-related changes in the adaptive immune system are well documented, aging of the innate immune system remains less well understood, particularly in nonhuman primates. A more robust understanding of age-related changes in innate immune function would provide mechanistic insight into the increased susceptibility of the elderly to infection. Rhesus macaques have proved a critical translational model for aging research, and present a unique opportunity to dissect age-dependent modulation of the innate immune system. We examined age-related changes in: (i) innate immune cell frequencies; (ii) expression of pattern recognition receptors (PRRs) and innate signaling molecules; (iii) cytokine responses of monocytes and dendritic cells (DC) following stimulation with PRR agonists; and (iv) plasma cytokine levels in this model. We found marked changes in both the phenotype and function of innate immune cells. This included an age-associated increased frequency of myeloid DC (mDC). Moreover, we found toll-like receptor (TLR) agonists lipopolysaccharide (TLR4), fibroblast stimulating ligand-1 (TLR2/6), and ODN2006 (TLR7/9) induced reduced cytokine responses in aged mDC. Interestingly, with the exception of the monocyte-derived TNFα response to LPS, which increased with age, TNFα, IL-6, and IFNα responses declined with age. We also found that TLR4, TLR5, and innate negative regulator, sterile alpha and TIR motif containing protein (SARM), were all expressed at lower levels in young animals. By contrast, absent in melanoma 2 and retinoic acid-inducible gene I expression was lowest in aged animals. Together, these observations indicate that several parameters of innate immunity are significantly modulated by age and contribute to differential immune function in aged macaques.
This paper carries supplementary material (Supplemental Table S1, Supplemental Figure S1).
The immune system is comprised of two major arms: innate and adaptive immunity. The ancestral innate immune system is characterized by its ability to rapidly respond to pathogen insult, its use of germline-encoded receptors, and its key role in modulating the quality, magnitude, and timing of adaptive immunity Citation1. On the other hand, adaptive immunity is characterized by its diversity in antigen recognition by T and B lymphocytes, and generation of lifelong memory. Both infants and the elderly are exquisitely susceptible to infection and mount weaker immune responses to vaccination, with shorter magnitude and delayed kinetics [reviewed by Siegrist and Aspinall Citation2 and Siegrist Citation3]. However, our understanding of mechanisms underlying this immune deficiency at the extremes of age has been varyingly successful. It is increasingly clear that the adaptive immune system undergoes profound age-dependent changes. For example, infants have a preponderance of naïve lymphocytes and mount limited IgG responses and exhibit Th2 biased cellular immunity to several vaccines [reviewed by Adkins et al. Citation4]. In contrast, the elderly exhibit an accumulation of terminally differentiated effector memory (EM) T cells, with dysregulated cytokine production and effector function Citation2, Citation5, Citation6.
Quantitative or qualitative changes in innate immune responses with age can significantly impact the immune response to infection by interfering with the development of a protective adaptive immune response – through defects in antigen presentation and/or cytokine production. In parallel, the autonomous role of innate immunity in effective microbial recognition, inflammatory signaling, and pathogen removal may also decline with age. For instance, monocytes/macrophages and dendritic cell (DC) subsets such as myeloid and plasmacytoid DC have the ability to present antigens and are susceptible to activation by microbe-associated molecular patterns (MAMPs) through their ubiquitous expression of pattern recognition receptors (PRRs) Citation7. Despite growing knowledge of the cell types, cellular receptors, downstream signaling pathways, and effector cytokines integral to efficient innate immunity, their modulation with age is less well defined – particularly in nonhuman primates. In the elderly, antigen-presenting cells (APC) may exhibit a more inflammatory phenotype, with increased spontaneous cytokine production Citation8. Coupled with the accumulation of differentiated memory T cells, these inflammatory changes may contribute to increased inflammation in the elderly, a phenotype described as ‘inflammaging’ Citation8 Citation9. By contrast, existing studies varyingly report that infant APC may express altered levels of toll-like receptors (TLR), exhibit diminished inflammatory signaling to microbial stimuli and have a diminished capacity to produce cytokines Citation10 Citation11 Citation12 .
In this study, we sought to determine age-related changes in innate immune function in rhesus macaques, a long-lived species of nonhuman primates with a high degree of genetic, physiological, and genetic relatedness to humans. We were particularly interested to establish whether there were numerical and/or functional differences in innate APC populations. Moreover, age-related changes in T cell homeostasis in rhesus macaques exhibit significant homology with those reported in humans Citation13. Similarly, the phenotype of APC subsets and TLR expression are similar in rhesus macaques and humans Citation14 Citation15. Therefore, rhesus macaques provide a robust model to dissect age-related changes in the phenotype and function of the innate immune compartment.
We report several age-related changes in cytokine responses by monocytes, myeloid DC (mDC), and plasmacytoid DC (pDC) to TLR agonism. We also find an age-dependent increase in the frequency of mDC (as a percentage of total DCs). Moreover, we find TLR4 and TLR5 expression is lower in young NHPs, juxtaposed by a decreased expression of innate negative regulator sterile alpha and TIR motif containing protein (SARM1), in this age group. In older animals, we also found decreased expression of PRRs absent in melanoma 2 (AIM2) and retinoic acid-inducible gene I (RIG-I). With respect to circulating plasma cytokine concentrations, IL-6 levels were highest in older animals, consistent with the concept of ‘inflammaging’, as was IL-1Ra. In contrast, IL-15 and IL-12 levels were highest in young animals, whereas plasma concentrations of TNFα, MCP-1, and sCD40L were age-independent.
To our knowledge, this is the first study to examine age-related changes in innate immune function at both extremes of age in this highly translational animal model of human aging. The findings presented in this study indicate that several phenotypic and functional features of innate immunity change significantly throughout life. Understanding underlying mechanisms that perturb innate immune function in infants and the elderly may offer new therapeutic avenues aimed at improving immune ‘fitness’ in these vulnerable populations.
Methods
Animals and sample collection
This study was carried out under strict accordance with the recommendations outlined in the Guide for the Care and Use of Laboratory Animals of the National Institute of Health, the Office of Animal Welfare and the United States Department of Agriculture. All animal studies were approved by the Oregon National Primate Research Center (ONPRC) Institutional Animal Care and Use Committee (IACUC). The ONPRC has been accredited by the American Association for Accreditation of Laboratory Animal Care since 1974 (PHS/OLAW Animal Welfare Assurance # A3304-01). All procedures were performed in the presence of qualified veterinary staff and all efforts made to minimize animal suffering. Animals used in this study were housed in indoor small group housing, and were free of overt signs of disease (neoplasms, acute infections, severe arthritis or wasting disease). Blood samples were collected from male and female young (1–4 yrs old, n=69), adult (5–18 yrs old, n=163), and aged (≥19 yrs old, n=85) rhesus macaque of Indian origin bred and maintained at the ONPRC. Peripheral blood mononuclear cells (PBMC) and plasma were isolated by centrifugation over histopaque (Sigma, St Louis, MO) as per manufacturer's protocol.
Flow cytometric analysis of immune cell subset frequencies
Peripheral blood mononuclear cell from our entire age cohort (age 1–30, n=317) were surface stained with antibodies against CD8β (Beckman Coulter, Brea, CA), CD4 (eBioscience, San Diego, CA), CD28, and CD95 (BioLegend, San Diego, CA) to delineate naive, central memory (CM), and EM CD4 and CD8 T cell subsets. For analysis of innate immune cells PBMC isolated from a subcohort of young (1–4 yrs, n=47), adult (5–18 yrs, n=119) and aged (≥19 yrs, n=43) animals were separately stained for CD3 (BD Pharmingen, San Diego, CA) CD20 (Beckman Coulter) CD14, HLA (Human Leukocyte Antigen)-DR, CD11c, and CD123 (Biolegend, San Diego, CA).
RNA isolation and cDNA synthesis
Total RNA was isolated from 1–2×106 PBMC (collected from ≥12 animals per age group) with Trizol reagent (Invitrogen, Grand Island, NY) as per manufacturer's instructions and stored at −80°C. Subsequently cDNA was synthesized using 0.5 µM T7 oligo(dT)-T7 primer and 250 U SuperScript III reverse transcriptase (Invitrogen). All cDNA samples were diluted in DNA buffer and stored at −80°C.
Real-time PCR
Quantitative real-time reverse transcriptase PCR (qRT-PCR) was used to quantify gene expression using primer and probe sets specific for each gene designed with Primer Express software (Applied Biosystems, Foster City, CA), using the published Macaca mulatta genomic reference sequence submitted by the Macaca mulatta Genome Sequencing Consortium to NCBI. A list of the primer and probe sequences used for this study are presented in Supplementary . Specific TaqMan custom probes (Applied Biosystems), custom primers (Invitrogen), and Maxima Probe/ROX qPCR Master Mix (2×; Fermentas, Glen Burnie, MD) were used for RT-qPCR reactions. Following an initial 10 min 95°C step, 40 cycles of 15 s at 95°C, and 1 min at 60°C were completed using a StepOnePlus RT-qPCR machine (Applied Biosystems).
Table 1. Summary of TLR responses in Macaca mulatta PBMC myeloid subsets
Plasmids containing each target amplicon (created using the pJET1.2 cloning system, Fermentas) or synthesized amplicons (Invitrogen) were used as quantification standards. The normalized expression level was calculated for each gene relative to the housekeeping gene M. mulatta glutathione synthetase (GSS).
PBMC stimulations
For PBMC stimulations cells were collected from a subcohort (n = 12–15 animals/group) of young (≤4 yrs), adult (8–14 yrs), and aged (≥19 yrs) animals. PBMC (2×106 cells resuspended in 200µl of RPMI-10% FBS, supplemented with streptomycin/penicillin and l-Glu) were plated on a 96 well round bottom plate and incubated for 6 h at 37°C with media alone (unstimulated) or with the addition of one following TLR agonists (Invivogen, San Diego, CA): FSL-1 (synthetic lipoprotein of Mycoplasma salivarium; 1 µg/ml); LPS (Escherichia. coli K12 Lipopolysaccharide; 2 ng/ml), ODN2006 (synthetic oligonucleotide containing unmethylated CpG; 1.25 µM) and Imiquimod (imidazoquinoline amine analogue to guanosine, 10 µg/ml). Agonist concentrations were selected based on preliminary studies of maximal responses in PBMC collected from 10–12 year old rhesus macaques and the manufacturer's suggested ranges.
For the analysis of innate immune cell cytokine production, PBMC were stained with the innate surface panel described above, with the addition of Aqua LIVE/DEAD stain (Invitrogen) to exclude dead cells. Following surface staining, cells were fixed and permeabilized according to the manufacturer's instructions (Biolegend) and stained for TNFα, IL-6 (eBioscience) and IFNα (PBL InterferonSource, Piscataway, NJ). IFNα was conjugated beforehand with Fluorescein using Zenon Mouse IgG Labeling Kit (Invitrogen) as per the manufacturer's instructions. All samples were analyzed using the LSRII instrument (Beckton, Dickinson and Company, San Jose, CA) and FlowJo software (TreeStar, Ashland, OR). In the case of stimulations, cytokine frequencies represent the stimulated sample value minus that of its unstimulated control.
Plasma cytokine analysis
Plasma samples (stored at −80°C) were thawed and diluted 1:2 in serum matrix for analysis with Milliplex Non-Human Primate Magnetic Bead Panel as per the manufacturer's instructions (Millipore Corporation, Billerica, MA). Concentrations for TNFα, IL-6, IL-12/23p40, IL-8, MCP-1, IL1Ra, soluble CD40L, IL-15, IFNγ, IL-4, and IL-17 were determined for all samples. Values below the limit of detection of the assay were assigned a value half that of the lowest value recorded in that assay.
Statistical analysis
Statistical analysis and graphing was conducted with GraphPad Prism software (GraphPad Software, Inc, La Jolla, CA). Correlation analyses for were performed with the Spearman rank correlation test. Analyses that compared two age cohorts were done using a nonparametric Mann–Whitney U test or Kruskal–Wallis and Dunn's Multiple Comparison test appropriate for sample size.
Fig. 1. T cell frequencies in young, adult, and aged macaque. Young (≤4 yrs, n=69), adult (5–18 yrs, n=163), and aged (≥19 yrs, n=85) PBMC were stained to determine the frequency of naïve (A,B), central memory (C,D), and effector memory (E,F) CD4 or CD8 T cells. The total frequency of CD4 (G) and CD8 (H) T cells is also shown, as well as CD/CD8 ratios (I). Correlations between age and cell frequencies/ratios were determined by Spearman's rank correlation.
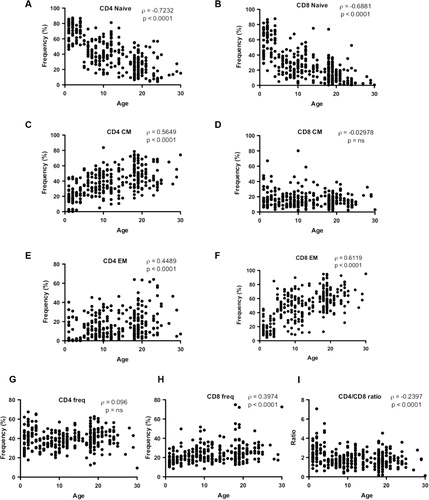
Results
Age-dependent changes in rhesus macaque T cell homeostasis mirror those of humans
Loss of naïve T cells and accumulation of memory T cells, especially terminally differentiated CD28– T cells is a hallmark of immune senescence Citation16. To confirm that our rhesus macaque cohort displayed known age-related changes in T cell subset distribution, we measured the frequency of naïve (CD28 + CD95–),CM (CD28 + CD95 + ), and EM (CD28–CD95 + ) circulating CD4 and CD8 T cells using flow cytometry (). We observed a highly significant loss of both CD4 and CD8 naïve T cells with age (A–F), with a concomitant accumulation of CD4 and CD8 effector memory cells (E,F). This gross acquisition of effector memory phenotype was accompanied by a CD4 T cell-specific accumulation of cells with a CM phenotype with age (C), a correlation that was not observed within the CD8 T cell pool (D). Another significant alteration amidst the T cell pool was the significant increase in total CD8 T cell frequency with age (G) whereas total CD4 frequency remained stable (H). This gradual increase in total CD8 frequency also correlated with a significant age-dependent decrease in the circulating CD4/CD8 ratio (F).
Age-dependent changes in the cellular composition of the innate immune compartment
We then investigated age-related changes in the cellular composition of the innate immune compartment. To this end, we determined the relative frequency of monocytes (CD3–CD20–DR + CD14 + ), mDC (CD3–CD20–CD14-DR + CD11c + ), pDC (CD3–CD20–CD14–DR + CD123 + ), and ‘other DC’ (CD3–CD20–CD14–DR + CD11c–CD123–; gating strategy A). The frequency of CD3–CD20– nonlymphocytes amongst PBMC was moderately reduced in aged animals (≥19 years) relative to adults (5–18 yrs) or juveniles (≤4 yrs; B). Despite broad decreases in the total numbers of circulating monocytes and DC with advancing age (Supplementary A–F), the frequency of these cells did not universally exhibit this trend (B–G). For example, we found a small, albeit significant, reduction in the frequency of monocytes in juveniles relative to older animals (C). By contrast, the total frequency of DC was independent of age group (D). Nonetheless, striking age-dependent differences where observed within the DC compartment. Notably, the frequency of mDC was significantly increased with each age group (E), with a reciprocal and significant decline in the ‘other DC’ population (G), while the frequency of pDC remained stable with age (F).
Fig. 2. Innate immune cell frequencies in young, adult, and aged macaque. (A) Flow cytometric gating strategy to identify monocytes and dendritic cell (DC) populations. (B–D) The frequency of nonlymph (CD3-CD20-), monocytes and DCs (as frequencies of nonlymph cells) was determined in young (≤4 yrs, n=47), adult (5–18 yrs, n=119), and aged (≥19 yrs, n=43) animals. The frequency of myeloid DC (E), plasmacytoid DC (F), and ‘other DC’ (G) amongst total DC (CD14-HLA-DR+ cells) is also shown. *p≤0.05 (as compared to adults).
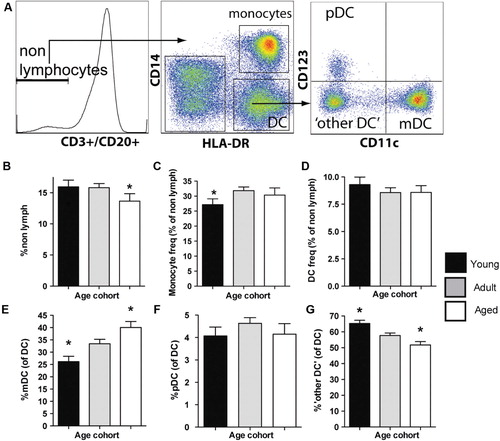
Pattern recognition receptor expression in juvenile, adult, and aged Peripheral blood mononuclear cell
Pattern recognition receptor-mediated pathogen recognition is a central mechanism with which innate immune cells sense an infectious insult and modulate the quality and magnitude of adaptive immune responses [reviewed by Schenten and Medzhitov Citation17]. We therefore evaluated global changes in the expression level of PRRs with age. We first examined expression of TLR family members TLR1–TLR9 in total PBMCs, using qRT-PCR with rhesus-specific primer and probe sets (). Membrane-bound TLRs recognize a diverse array of MAMPs, including those of bacteria, viruses, and parasites as well as endogenous ligands associated with cell stress or tissue damage (so called danger-associated molecular patterns) Citation17. Interestingly, the vast majority of TLRs exhibited comparable expression levels in juvenile, adult, and aged animals’ PBMC (). However, we did observe significantly reduced expression of both TLR4 and TLR5 in juvenile relative to adult PBMC ().
Fig. 3. Toll-Like Receptor expression in young, adult, and aged macaque PBMC. Expression levels of TLR1-9 relative to housekeeping gene MGSS were determined by qRT-PCR using primers and probes designed for Macaca mulatta. Young (1–4 yrs), adult (8–14yrs), and aged (≥19 yrs) cohorts are shown. Bars graphs represent group mean±SEM. n=17–28/age group. *p≤0.05.
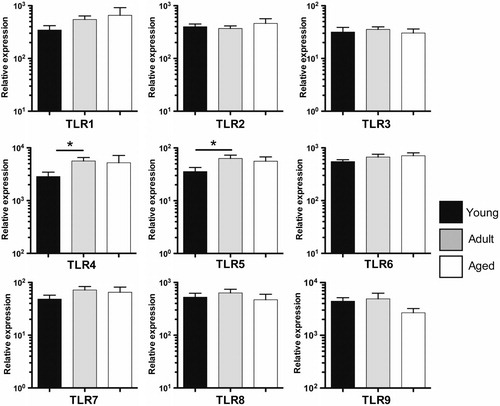
We extended our analysis of PBMC PRR expression to nucleic acid sensors. More specifically we examined expression of the intracellular RNA sensors: (i) RIG-I; and (ii) melanoma differentiation-associated gene 5 [MDA5; reviewed by Barbalat et al. (2011) Citation18]. We also examined expression of the intracellular DNA sensors: (i) DNA-dependent activator of IFN-regulatory factors (DAI); (ii) IFNγ-inducible protein 16 (IFI16); and (iii) AIM2 Citation18. These analyses revealed that RIG-I and AIM2 expression were both significantly reduced in aged animals as compared to adult controls (). In addition to these nucleic acid sensors, we also determined the age-related changes in expression of PRRs associated with the formation of the inflammasome innate signaling platform, notably NOD-like receptor (NLR) family member NLRP3 Citation19. Finally, we characterized changes in three major PRR signaling adaptors: (i) TLR-signaling adaptor myeloid differentiation primary response gene (MyD88); (ii) RIG-I-Like Receptor (RLR)-signalling molecule mitochondrial antiviral-signaling protein (MAVS); and (iii) stimulator of interferon genes protein (STING) – activated by DAI and IFI16. In contrast to RIG-I and AIM2, NLRP3-, MyD88-, STING-, and MAVS-expression was not altered with age (). Thus, although PRR expression is largely conserved with age, both juvenile animals (TLR4, TLR5) and aged animals (AIM2, RIG-I) exhibit specific defects in PRR expression.
Fig. 4. NLR/RLR/signaling adaptor expression in young, adult, and aged macaque PBMC. Expression levels of RIG-I, MDA5, DAI, IFI16, AIM2, NLRP3, MyD88, MAVS, and STING relative to housekeeping gene MGSS were determined by qRT-PCR using primers and probes designed for Macaca mulatta. Young (1–4 yrs), adult (8–14 yrs), and aged (≥19 yrs) cohorts are shown. Bars graphs represent group mean±SEM. n=12–18/age group. *p≤0.05.
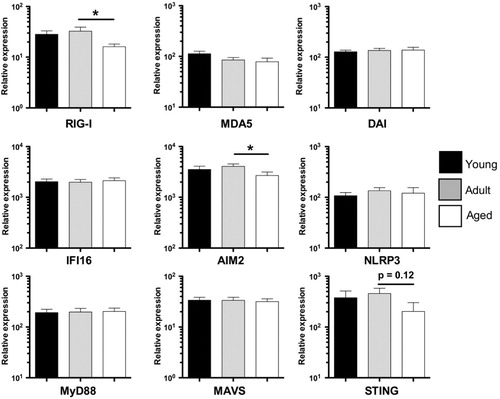
Expression of innate regulatory proteins with age
Since we did not detect major changes in PRR expression, we hypothesized that perturbed innate immune function in the young or aged might instead manifest as age-related changes in the expression of innate immune regulatory proteins. We selected target genes based on their known function in suppressing innate immune responses: Toll interacting protein (Tollip) and IL-1 receptor associated kinase M (IRAK-M) negatively regulate TLR responses Citation20 Citation21; NLR family member XI (NLRX1) inhibits both TLR and RLR signaling Citation22 Citation23; activating transcription factor 3 (ATF3) is induced by TLR agonists and inhibits IL-6/IL-12 productionCitation24; SARM1 negatively regulates TLR-adaptors TRIF and MyD88 Citation25 Citation26; and E3 ubiquitin ligase RBCC protein interacting with PKC1 (RBCK1) modulates the activity of NFκB, IRF3, and TNFα Citation27 Citation28 Citation29 Citation30 . Analysis of the innate negative regulators revealed that the expression of Tollip, IRAK-M, ATF3, and RBCK1 in total PBMC remained stable with increasing age (). On the other hand, NLRX1 and SARM expression was significantly reduced in juveniles relative to adult and aged animals ().
Fig. 5. Innate negative regulator expression in macaque PBMC Expression levels of innate negative regulators Tollip, IRAK-M, NLRX1, ATF3, SARM1, and RBCK relative to housekeeping gene MGSS were determined by qRT-PCR using primers and probes designed for Macaca mulatta. Young (1–4 yrs), adult (8–14 yrs), and aged (≥19 yrs) cohorts are shown. Bars graphs represent group mean±SEM. n=12–18/age group. *p≤0.05; ***p≤0.001.
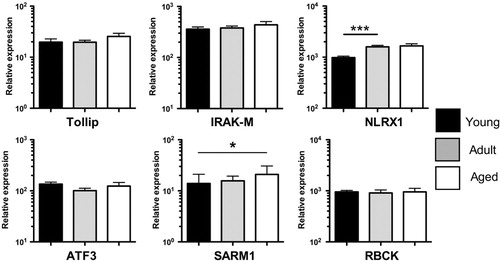
Age dependent PRR responses in rhesus macaque PBMC
In light of the differential expression of select PRRs and their regulators in juvenile, adult, and aged macaque PBMC, we next examined the cytokine response of peripheral myeloid cells to innate stimuli. To this end, we stimulated PBMC with PRR agonists to mimic exposure to either bacterial or viral MAMPs. PBMC were stimulated for 6 h in the presence of FSL-1 (TLR2/TLR6 agonist), LPS (TLR4 agonist), or ODN2006 (TLR9 agonist) and intracellular cytokine production assessed by flow cytometry. Strikingly, both monocytes and mDC showed marked age-dependent differences in MAMP-induced cytokine responses (A–E, representative staining A). Specifically, in response to TLR2/6 or TLR4 agonists, the frequency of TNFα+ or IL-6+ mDCs significantly declined as a function of age, with the highest responses observed in juvenile animals (D,E). This trend was also seen in response to TLR9 agonist ODN2006 (D,E).
Fig. 6. Age-dependent innate TLR responses in macaque monocytes and myeloid DC. Two million PBMC were stimulated for 6 h in the presence of brefeldin A with the following TLR agonists: TLR2/6 (FSL-1 – 1 µg/ml); TLR4 (LPS – 20 ng/ml); and TLR9 (ODN2006 – 10 µM). Cells were stained to distinguish monocytes and mDC and stained intracellularly for cytokines. Representative flow cytometry is shown for unstimulated and LPS-stimulated monocytes (A). Frequency of TNF+ or IL-6+ responding monocytes (B,C) and mDC (D,E) is shown for each agonist. Bar graphs represent mean frequency of cytokine producing cells ±SEM (adjusted for background using unstimulated controls). n=12–15 animals/age group. *p≤0.05; **p≤0.01; ***p≤0.001.
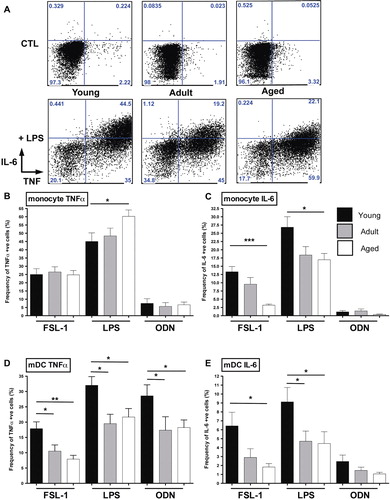
Similarly, IL-6 production by monocytes also declined with age in response to either FSL-1 or LPS (C). In contrast, LPS-stimulated monocytes from aged animals showed the highest frequency of TNFα production (B). The frequency of monocytes producing TNFα in response to the other TLR agonists, however, was equivalent in all three age groups (B). Together these findings indicate the increased cytokine response in monocytes is TLR4-specific and restricted to increased TNFα rather than IL-6 induction.
We next extended our analysis to nonconventional or ‘other DC’ and pDC (A–D). Whereas the TNFα response of the ‘other DC’ population to FSL-1, LPS, and ODN2006 was age-independent, IL-6 production by ‘other DC’ following FSL-1 and LPS stimulation was reduced in aged animals relative to young animals (A,B). Since pDCs have a highly restricted expression of TLRs (TLR7 and TLR9), we limited our analysis to PBMC stimulated with Imiquimod (TLR7) or ODN2006 (TLR9; C,D). As expected, pDC from all age groups produced a robust TNFα-response to both agonists, with older animals hyporesponsive to TLR9 agonism ( C,D). Relatively few pDCs made IL-6 in response to Imiquimoid and ODN2006 stimulation, albeit with a trend for decreased IL-6 production with age (D). Finally, we also analyzed the IFNα response by pDC. The mean frequency of IFNα+ cells in response to imiquimod was highest in juvenile animals and declined with increasing age, although this difference was not statistically significant (A,B). We did not observe a detectable IFNα response by pDC to ODN2006 (data not shown). Together these observations suggest that aging is associated with a reduced response of pDC to TLR agonism.
Fig. 7. Age-dependent innate TLR responses in macaque DC subpopulations. Two million PBMC were stimulated for 6 h in the presence of brefeldin A with agonists for the following TLR: TLR2/6 (FSL-1 – 1 µg/ml); TLR4 (LPS – 20 ng/ml); TLR7/8 (Imiquimod − 10 µg/ml); and TLR9 (ODN2006 − 10 µM). Cells were stained to distinguish CD11c-CD123-DC (‘other’ DC) and pDC and stained intracellularly for cytokines. Frequency of TNF+ or IL-6+ responding ‘other DC’ (A,B) and pDC (C,D) is shown for the agonists described. Bar graphs represent mean frequency of cytokine producing cells ±SEM (adjusted for background using unstimulated controls). n=12–15 animals/age group. *p≤0.05; ***p≤0.001.
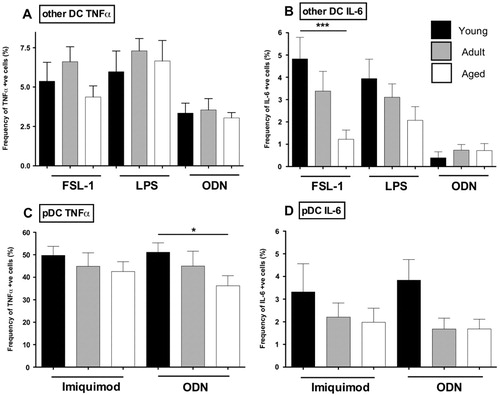
Fig. 8. IFNα responses in young, adult, and aged macaque plamacytoid DC. Two million PBMC were stimulated with Imiquimod (TLR7 agonist; 10 µg/ml) for 6 h in the presence of brefeldin A. Frequency of IFNα responding plasmacytoid DC was subsequently measured by FCS (A), with representative flow cytometry also shown (B). Bar graphs represent mean frequency of cytokine producing cells ±SEM (adjusted for background using unstimulated controls). n=12–15 animals/age group.
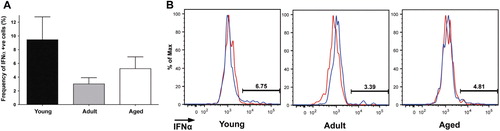
Age-dependent circulating cytokine levels
Since we found marked age-dependent differences in the cytokine responses of innate immune cells, we next determined if global circulating cytokine levels also changed with age. We analyzed serum cytokine levels of TNFα, IL-6, IL-12/23(p40), IL-8, monocyte chemoattractant protein 1 (MCP-1), IL-1Ra, and soluble CD40L, all of which were selected for their close association with innate immune effector function/acute systemic inflammatory responses (). We also included IL-15, IFNγ, IL-4, and IL-17 () in light of the strong modulation of the CD4/CD8 T cell compartment with age (). Interestingly, the mean serum concentrations for several cytokines were highly age-dependent. Both IL-6 and IL-1Ra levels were highest in aged animals, whereas juvenile animals exhibited high levels of IL-8 and IL-12/23(p40). By contrast, the plasma concentrations of TNFα, MCP-1, and sCD40L did not change with age (). In addition we observed a trend toward increasing IL-17, and a significant decrease in IL-15 concentration with advancing age (), whereas Th1/Th2 cytokines IFNγ and IL-4 levels were not significantly elevated. These findings support the hypothesis that circulating basal cytokine levels in the rhesus macaque exhibit changes throughout life.
Discussion
Immunological changes associated with advancing age have been largely studied within the context of adaptive immunity. In contrast, age-associated changes in the cellular composition and effector function of the innate arm of the immune system remain poorly understood. To address this knowledge gap, we characterized changes in the frequency and function of innate immune cells in the rhesus macaque, a highly relevant model of human aging. As previously described [reviewed by Arnold et al. Citation31 and Messaoudi and Ingram Citation32], we report striking changes in the phenotype of the T cell pool with advancing age in this cohort, with an accumulation of effector T cells, decreased CD4/CD8 ratio, and loss of naïve T cells. We also report that monocyte frequencies are comparable in adult and aged animals, consistent with previous reports in humans and NHPs Citation33 Citation34. Interestingly, Nguyen et al. recently found aged human PBMC have a higher frequency of CD16-expressing monocyte subsets within the CD14+ monocyte compartment Citation35. This indicates population changes within the monocyte compartment itself may still occur with age. Similarly, although we did not find a difference in total DC frequency (CD3–CD20–HLA–DR + CD14+ cells), we did find significant differences within the cellular composition of this compartment, with an age-dependent shift toward mDC and decrease in CD123–CD11c–DR+ DCs.
We also investigated the cytokine response of specific innate immune cells following stimulation with PRR agonists such as LPS. Previous studies in baboons and rhesus macaques found comparable TNFα and IL-6 production in response to LPS between adult and aged PBMC Citation36 Citation37. However, studies of global cytokine responses with age may mask functional differences within distinct innate immune cell populations. Therefore in our study, we examined IL-6 and TNFα production by specific myeloid cell subsets using flow cytometry. These responses are summarized in . Using a comparable strategy of PBMC stimulation and flow cytometric analysis to study TNFα and IL-6 production on a per cell basis, van Duin et al. Citation38 found no decrease in TLR2/6 (FSL-1), TLR4 (LPS), and TLR9 (ODN2006) responsiveness in monocytes from aged (65+ yrs) versus adult (21–30 yrs) donors. Thus our findings collectively suggest that several monocyte TLR responses are comparable between adulthood and old age. Contrary to their findings, we found that the TNFα response of monocytes was highest in aged animals. This difference may reflect differences in age cohorts used in our respective studies or species-specific differences.
With respect to DCs, Panda et al. similarly stimulated PBMC from young (21–30yrs) and aged (65+ yrs) human donors to examine DC cytokine responses on a per cell basis by flow cytometry Citation39. Partially mirroring our findings, mDC and pDC TNF/IL-6 responses to multiple TLR agonists were highest in the younger age group. We propose that this age group may have behaved more similarly to our young animals (aged 1–4) than our adult cohort (aged 8–14), since TNF/IL-6 responses were broadly maintained between adult and aged animals, whereas young animals had significantly higher mDC/pDC cytokine responses than both groups.
In our youngest age group, we find that TLR responses are broadly conserved, if not higher, than adult or aged animals. Previous studies of TLR responses in the young have largely focused on those of neonatal cord blood cells Citation10 Citation11 Citation12 Citation40. More recent longitudinal studies in infants indicate that initially hyporesponsive TLR responses in neonates rapidly increase in the first 1–2 years of life Citation41 Citation42 Citation43 . Interestingly, perhaps the most similar analysis to our own (in which cell type-specific TLR responses were also analyzed) found TLR1/2, TLR4, TLR7/8, and TLR9 agonists induced monocyte and mDC cytokine responses (as measured by frequency of TNFα, IL-6, and IL-12p40 production) equivalent or higher in infants (aged 1–2) than adults (aged 23–48) Citation41. This study and our own also found that the frequency of IFNα+ pDC following TLR7 agonism was not diminished in the young compared to adults Citation41. Together these findings indicate that rather than being impaired, TLR responses in infants are strikingly robust.
In addition, our global analysis of PBMC PRR expression with age revealed several age-dependent differences. We found that TLR4 and TLR5 expression is lower in young macaques compared to adult and aged animals. Strikingly, we also found that the expression of innate negative regulator SARM was lowest in the juvenile animals. SARM is a negative regulator of TRIF-driven TLR3 and TLR4 signaling Citation26 Citation44. Thus reduced innate negative regulation, despite lower TLR4 expression, may be mechanistically important for the maintenance of robust LPS responses in infants. With respect to TLR-independent pattern recognition, we found RIG-I and AIM2 expression significantly reduced in our aged cohort compared to our adult cohort. RIG-I is reported to mediate anti-viral responses against a number of clinically significant pathogens such as influenza type A and West Nile Virus Citation45 Citation46 Citation47 , which cause increased morbidity and mortality in the elderly Citation48 Citation49. AIM2 is thought to respond to the dsDNA of bacteria and viruses such as Listeria monocytogenes Citation50 Citation51, the causative agent of Listeriosis that also disproportionately affects the elderly population Citation52. It will, therefore, be of interest to establish whether diminished RIG-I or AIM2 expression in humans may contribute to enhanced susceptibility to these pathogens in the aged.
Differences in PRR expression level appear restricted to specific PRR rather than broad changes in the innate immune receptor repertoire. It is important to be mindful however that age-dependent changes in total PBMC PRR expression may not mirror those at the population level. In future studies we plan to examine PRR expression at the population level to better determine how age-dependent PRR expression may modulate cell-specific innate immune responses.
Our analysis of circulating cytokine levels also yielded interesting findings. With respect to TNFα, MCP-1, IL-8, and IL-12 we did not observe elevated plasma levels of these cytokines in aged animals relative to adults. This conflicts with the paradigm stemming from early studies of immune senescence that suggested aging is associated with higher levels of circulating proinflammatory cytokines [reviewed in Ref. Citation8 Citation53]. Indeed, our data support the growing consensus that once co-morbidities and nutritional status are controlled for, enhanced circulating proinflammatory cytokine levels are not intrinsic per se to the aging process Citation54. Nonetheless, we did find that the plasma levels of IL-12 and IL-15 decreased with age, whereas IL-6, IL-17, and IL-1Ra increased. Circulating levels of IL-12 were highest in younger animals. Since this cytokine, alongside TNFα and IL-6, is induced by NFκB activation Citation55, this may be consistent with the broadly stronger TLR responses observed in juvenile animals. While IL-6 plays several inflammatory roles, e.g. promoting monocyte recruitment and up-regulating adhesion molecule expression, it is also regarded as a regulatory cytokine Citation56. Thus, the parallel rise in IL-1Ra with advancing age may even represent a shift toward regulatory cytokines in elderly NHPs. On the other hand, both IL-6 and IL-17 are associated with the induction and effector function of Th17 cells respectively Citation57, and increased levels of these cytokines could also indicate a shift of the effector T cell pool toward a Th17 profile with advancing age. IL-15 is another innate cytokine that modulates effector T cell activity Citation58 and plays an important role in maintaining central and effector memory T cells Citation59. It is, therefore, striking that these subsets accumulate with age, despite declining levels of this cytokine. This may indicate that IL-15 independent mechanisms or highly localized effects of this cytokine contribute to the maintenance of the memory T cell pool in aged macaques.
In summary, the use of a broad rhesus macaque age cohort has enabled us to dissect several age-related changes in innate immune phenotype and function. We propose that modulating myeloid cell numbers, PRR expression, and innate cytokine responses are promising avenues for improving immune function in the vulnerable young and elderly populations.
Conflict of interest and funding
The authors have not received any funding or benefits from industry or elsewhere to conduct this study.
Supplementary Table S1
Download PDF (123.8 KB)Supplementary Figure S1
Download PDF (164.1 KB)Acknowledgements
We would like to thank Jose Vasquez, Spencer Chang, and Craig Kreklywich for their technical assistance, as well as the Division of Animal Resources (DAR) at the Oregon National Primate Research Center for expert animal care. We would also like to thank the Program for Aging Studies at the ONPRC especially Dr Steven Kohama. Our gratitude goes to Alfred Legasse, Miranda Fischer, and Jesse Dewane for the collection of blood and BAL samples. This work was supported by NIH R01AG037042, 5T32 A1078903-02, NIH 8P51 OD011092-53, and the Brookdale Foundation.
References
- Iwasaki A, Medzhitov R. Regulation of adaptive immunity by the innate immune system. 2010; 327: 291-5.
- Siegrist CA, Aspinall R. B-cell responses to vaccination at the extremes of age. 2009; 9: 185-94.
- Siegrist CA. Neonatal and early life vaccinology. 2001; 19: 3331-46.
- Adkins B, Leclerc C, Marshall-Clarke S. Neonatal adaptive immunity comes of age. 2004; 4: 553-64.
- Yoshikawa TT. Epidemiology and unique aspects of aging and infectious diseases. 2000; 30: 931-33.
- Yoshikawa TT. Perspective: aging and infectious diseases: past, present, and future. 1997; 176: 1053-57.
- Kawai T, Akira S. Toll-like receptors and their crosstalk with other innate receptors in infection and immunity. 2011; 34: 637-50.
- Plackett TP., Boehmer ED, Faunce DE, Kovacs EJ. Aging and innate immune cells. 2004; 76: 291-9.
- Franceschi C, Capri M, Monti D, Giunta S, Olivieri F, Sevini F et al. Inflammaging and anti-inflammaging: a systemic perspective on aging and longevity emerged from studies in humans. 2007; 128: 92-105.
- Levy O, Zarember KA, Roy RM, Cywes C, Godowski PJ, Wessels MR. Selective impairment of TLR-mediated innate immunity in human newborns: neonatal blood plasma reduces monocyte TNF-alpha induction by bacterial lipopeptides, lipopolysaccharide, and imiquimod, but preserves the response to R-848. 2004; 173: 4627-34.
- Wynn JL., Levy O. Role of innate host defenses in susceptibility to early-onset neonatal sepsis. 2011; 37: 307-37.
- Angelone DF, Wessels MR, Coughlin M, Suter EE, Valentini P, Kalish LA et al. Innate immunity of the human newborn is polarized toward a high ratio of IL-6/TNF-alpha production in vitro and in vivo. 2006; 60: 205-9.
- Pitcher CJ, Hagen SI, Walker JM, Lum R, Mitchell BL, Maino VC et al. Development and homeostasis of T cell memory in rhesus macaque. 2002; 168: 29-43.
- Ketloy C, Engering A, Srichairatanakul U, Limsalakpetch A, Yongvanitchit K, Pichyangkul S et al. Expression and function of Toll-like receptors on dendritic cells and other antigen presenting cells from non-human primates. 2008; 125: 18-30.
- Coates PT, Barratt-Boyes SM, Zhang L, Donnenberg VS, O'Connell PJ, Logar AJ et al. Dendritic cell subsets in blood and lymphoid tissue of rhesus monkeys and their mobilization with Flt3 ligand. 2003; 102: 2513-21.
- Pawelec G, Akbar A, Caruso C, Solana R, Grubeck-Loebenstein B, Wikby A. Human immunosenescence: is it infectious?. 2005; 205: 257-68.
- Schenten D, Medzhitov R. The control of adaptive immune responses by the innate immune system. 2011; 109: 87-124.
- Barbalat R, Ewald SE, Mouchess ML, Barton GM. Nucleic acid recognition by the innate immune system. 2011; 29: 185-214.
- Agostini L, Martinon F, Burns K, McDermott MF, Hawkins PN, Tschopp J. NALP3 forms an IL-1beta-processing inflammasome with increased activity in Muckle-Wells autoinflammatory disorder. 2004; 20: 319-25.
- Zhang G, Ghosh S. Negative regulation of toll-like receptor-mediated signaling by Tollip. 2002; 277: 7059-65.
- Kobayashi K, Hernandez LD, Galan JE, Janeway CA Jr., Medzhitov R, Flavell RA. IRAK-M is a negative regulator of Toll-like receptor signaling. 2002; 110: 191-202.
- Allen IC, Moore CB, Schneider M, Lei Y, Davis BK, Scull MA et al. NLRX1 protein attenuates inflammatory responses to infection by interfering with the RIG-I-MAVS and TRAF6-NF-kappaB signaling pathways. 2011; 34: 854-65.
- Xia X, Cui J, Wang HY, Zhu L, Matsueda S, Wang Q et al. NLRX1 negatively regulates TLR-induced NF-kappaB signaling by targeting TRAF6 and IKK. 2011; 34: 843-53.
- Gilchrist M, Thorsson V, Li B, Rust AG, Korb M, Roach JC et al. Systems biology approaches identify ATF3 as a negative regulator of Toll-like receptor 4. 2006; 441: 173-8.
- Peng J, Yuan Q, Lin B, Panneerselvam P, Wang X, Luan XL et al. SARM inhibits both TRIF- and MyD88-mediated AP-1 activation. 2010; 40: 1738-47.
- Carty M, Goodbody R, Schroder M, Stack J, Moynagh PN, Bowie AG. The human adaptor SARM negatively regulates adaptor protein TRIF-dependent Toll-like receptor signaling. 2006; 7: 1074-81.
- Ikeda F, Deribe YL, Skanland SS, Stieglitz B, Grabbe C, Franz-Wachtel M et al. SHARPIN forms a linear ubiquitin ligase complex regulating NF-kappaB activity and apoptosis. 2011; 471: 637-41.
- Tokunaga F, Nakagawa T, Nakahara M, Saeki Y, Taniguchi M, Sakata S et al. SHARPIN is a component of the NF-kappaB-activating linear ubiquitin chain assembly complex. 2011; 471: 633-36.
- Gerlach B, Cordier SM, Schmukle AC, Emmerich CH, Rieser E, Haas TL et al. Linear ubiquitination prevents inflammation and regulates immune signalling. 2011; 471: 591-6.
- Zhang M, Tian Y, Wang RP, Gao D, Zhang Y, Diao FC et al. Negative feedback regulation of cellular antiviral signaling by RBCK1-mediated degradation of IRF3. 2008; 18: 1096-1104.
- Arnold CR, Wolf J, Brunner S, Herndler-Brandstetter D, Grubeck-Loebenstein B. Gain and loss of T cell subsets in old age – age-related reshaping of the T cell repertoire. 2011; 31: 137-46.
- Messaoudi I, Ingram DK. Overview of aging research using nonhuman primate models. Age (Dordr). 2011..
- Xia HJ, Zhang GH, Wang RR, Zheng YT. The influence of age and sex on the cell counts of peripheral blood leukocyte subpopulations in Chinese rhesus macaques. 2009; 6: 433-40.
- Takahashi I, Ohmoto E, Aoyama S, Takizawa M, Oda Y, Nonaka K et al. Monocyte chemiluminescence and macrophage precursors in the aged. 1985; 39: 447-51.
- Nyugen J, Agrawal S, Gollapudi S, Gupta S. Impaired functions of peripheral blood monocyte subpopulations in aged humans. 2010; 30: 806-13.
- McFarlane D, Wolf RF, McDaniel KA, White GL. Age-associated alteration in innate immune response in captive baboons. 2011; 66: 1309-17.
- Mascarucci P, Taub D, Saccani S, Paloma MA, Dawson H, Roth GS et al. Cytokine responses in young and old rhesus monkeys: effect of caloric restriction. 2002; 22: 565-71.
- van Duin D, Mohanty S, Thomas V, Ginter S, Montgomery RR, Fikrig E et al. Age-associated defect in human TLR-1/2 function. 2007; 178: 970-5.
- Panda A, Qian F, Mohanty S, van Duin D, Newman FK, Zhang L et al. Age-associated decrease in TLR function in primary human dendritic cells predicts influenza vaccine response. 2010; 184: 2518-27.
- Philbin VJ, Levy O. Developmental biology of the innate immune response: implications for neonatal and infant vaccine development. 2009; 65: 98R-105R.
- Corbett NP, Blimkie D, Ho KC, Cai B, Sutherland DP, Kallos A et al. Ontogeny of Toll-like receptor mediated cytokine responses of human blood mononuclear cells. 2010; 5: e15041.
- Nguyen M, Leuridan E, Zhang T, De Wit D, Willems F, Van Damme P et al. Acquisition of adult-like TLR4 and TLR9 responses during the first year of life. 2010; 5: e10407.
- Belderbos ME, van Bleek GM, Levy O, Blanken MO, Houben ML, Schuijff L et al. Skewed pattern of Toll-like receptor 4-mediated cytokine production in human neonatal blood: low LPS-induced IL-12p70 and high IL-10 persist throughout the first month of life. 2009; 133: 228-37.
- Mink M, Fogelgren B, Olszewski K, Maroy P, Csiszar K. A novel human gene (SARM) at chromosome 17q11 encodes a protein with a SAM motif and structural similarity to Armadillo/beta-catenin that is conserved in mouse Drosophila, and Caenorhabditis elegans. 2001; 74: 234-44.
- Hornung V, Ellegast J, Kim S, Brzozka K, Jung A, Kato H et al. 5′-Triphosphate RNA is the ligand for RIG-I. 2006; 314: 994-7.
- Kato H, Takeuchi O, Sato S, Yoneyama M, Yamamoto M, Matsui K et al. Differential roles of MDA5 and RIG-I helicases in the recognition of RNA viruses. 2006; 441: 101-5.
- Fredericksen BL, Gale M Jr. West Nile virus evades activation of interferon regulatory factor 3 through RIG-I-dependent and -independent pathways without antagonizing host defense signaling. 2006; 80: 2913-23.
- Gubler DJ. The continuing spread of West Nile virus in the western hemisphere. 2007; 45: 1039-46.
- La Gruta NL, Kedzierska K, Stambas J, Doherty PC. A question of self-preservation: immunopathology in influenza virus infection. 2007; 85: 85-92.
- Rathinam VA, Jiang Z, Waggoner SN, Sharma S, Cole LE, Waggoner L et al. The AIM2 inflammasome is essential for host defense against cytosolic bacteria and DNA viruses. 2010; 11: 395-402.
- Fernandes-Alnemri T, Yu JW, Juliana C, Solorzano L, Kang S, Wu J et al. The AIM2 inflammasome is critical for innate immunity to Francisella tularensis. 2010; 11: 385-93.
- Lund BM, O'Brien SJ. The occurrence and prevention of foodborne disease in vulnerable people. 2011; 8: 961-73.
- Alvarez-Rodriguez L, Lopez-Hoyos M, Munoz-Cacho P, Martinez-Taboada VM. Aging is associated with circulating cytokine dysregulation. Cell Immunol. 2012.
- Kim HO, Kim HS, Youn JC, Shin EC, Park S. Serum cytokine profiles in healthy young and elderly population assessed using multiplexed bead-based immunoassays. 2011; 9: 113.
- Bonizzi G, Karin M. The two NF-kappaB activation pathways and their role in innate and adaptive immunity. 2004; 25: 280-8.
- Scheller J, Chalaris A, Schmidt-Arras D, Rose-John S. The pro- and anti-inflammatory properties of the cytokine interleukin-6. 2011; 1813: 878-88.
- McGeachy MJ, Cua DJ. Th17 cell differentiation: the long and winding road. 2008; 28: 445-53.
- Jakobisiak M, Golab J, Lasek W. Interleukin 15 as a promising candidate for tumor immunotherapy. 2011; 22: 99-108.
- Berger C, Berger M, Hackman RC, Gough M, Elliott C, Jensen MC et al. Safety and immunologic effects of IL-15 administration in nonhuman primates. 2009; 114: 2417-26.