Abstract
The McMurdo Dry Valleys are one of the most arid environments on Earth. Over the soil landscape for the majority of the year, biological and ecosystem processes in the dry valleys are constrained by the low temperatures and limited availability of water. The prevalence of these physical limitations in controlling biological and ecosystem processes makes the dry valleys a climate-sensitive system, poised to experience substantial changes following projected future warming. Short-duration increases in summer temperatures are associated with pulses of water from melting ice reserves, including glaciers, snow and permafrost. Such pulses alter soil geochemistry by mobilizing and redistributing soil salts (via enhanced weathering, solubility and mobility), which can alter habitat suitability for soil organisms. Resulting changes in soil community composition or distribution may alter the biogeochemical processes in which they take part. Here, we review the potential impacts of meltwater pulses and present new field data documenting instances of meltwater pulse events that result from different water sources and hydrological patterns, and discuss their potential influence on soil biology and biogeochemistry. We use these examples to discuss the potential impacts of future climate change on the McMurdo Dry Valley soil ecosystem.
The McMurdo Dry Valleys (MDV), the region between the Trans-Antarctic Mountains and the Ross Sea in South Victoria Land, Antarctica (76°30′–78°30′ S, 160–164° E), constitute the largest ice-free area in Antarctica and one of the southernmost functional terrestrial ecosystems. The MDV are among the most climatically extreme terrestrial habitats on Earth. As a polar desert, temperatures are very low (annual average −20°C) and precipitation is minimal (ranging from 3 to 55 mm water equivalent per year) with high sublimation rates, making it one of the coldest and driest deserts on Earth (Fountain et al. Citation1999; Fountain et al. Citation2010). Though these physical constraints limit biological communities to low diversity and biomass (Freckman & Virginia Citation1998; Virginia & Wall Citation1999), the MDV maintain functioning foodwebs and ecosystem processes with fewer metazoan taxa than any other natural terrestrial ecosystem (Barrett et al. Citation2006). Notably, the MDV also constitute one of the few well-studied terrestrial habitats on the Antarctic continent. Research conducted within the USA's National Science Foundation-funded McMurdo Long-Term Ecological Research Program (MCM LTER), Antarctica New Zealand, the Italian National Antarctic Program and the British Antarctic Survey maintain records of study for the Antarctic terrestrial ecosystems. Polar regions such as the Antarctic continent provide valuable insight into the consequences of global climate change, as they exhibit amplified ecosystem responses and provide early evidence of responses expected for ecosystems at lower latitudes (Chapin et al. Citation2005; Bernstein et al. Citation2007). It is therefore critical to understand how projected future climate change (Chapman & Walsh Citation2007; Steig et al. Citation2009; Walsh Citation2009) will influence terrestrial ecosystem processes in the MDV region.
The McMurdo Dry Valleys: a polar desert at a tipping point
The MDV are comprised of distinct landscape units that are connected by a hydrological regime: soils, glaciers, streams and lakes (Moorhead & Priscu Citation1998; Foreman et al. Citation2004; Barrett et al. Citation2007). However, soils are by far the most extensive of the components, with arid soils covering 95% of ice-free surfaces below 1000 m (Burkins et al. Citation2001). Soils in the dry valleys are poorly-developed and coarse (typically 95–99% sand), dry (largely <1–5% moisture), high in salt content and pH and low in organic matter (typically 0.1–0.3 mg organic C g soil−1), with a relatively shallow active layer (10–70 cm) underlain by 200–600 m of either ice-cemented or dry permafrost (Campbell & Claridge Citation1987; Campbell et al. Citation1997; Burkins et al. Citation2000; Bockheim et al. Citation2007). Despite the inhospitable conditions, biological activity is possible during the summer when air and soil temperatures are near or above freezing. However, the physical constraints (e.g., water and temperature) limit productivity to low levels relative to other terrestrial ecosystems (Friedmann et al. Citation1993; Parsons et al. Citation2004) and biological diversity and biomass to relatively “simple” soil communities that are dominated by microbes with a few taxa of invertebrates and no vascular plants (Freckman & Virginia Citation1998; Virginia & Wall Citation1999; Adams et al. Citation2007). The species distributions of those communities are generally determined by the interactions of soil chemistry, temperature and water availability rather than biotic interactions (e.g., competition and predation [Hogg et al. Citation2006]). For example, some invertebrate species (rotifers, tardigrades and two nematode species) dominate in wetter, higher-carbon soils while a single soil nematode species (Scottnema lindsayae) is widely distributed across dry, more saline and low-carbon soils (Virginia & Wall Citation1999; Courtright et al. Citation2001; Moorhead et al. Citation2002; Convey et al. Citation2003; Barrett et al. Citation2004; Barrett, Virginia, Wall et al. Citation2008). Thus habitat suitability, or the ability of the biota to achieve viable populations across a landscape, is largely dependent on the physical variation in the contemporary soil environment (Barrett et al. Citation2006) modified by the legacy of soil resources derived from past MDV climates (Moorhead et al. Citation1999; Burkins et al. Citation2000). Therefore, understanding the environmental factors that influence soil properties is important for understanding soil biology and the biogeochemical cycles for which they are responsible.
Notably, water is abundant in the MDV terrestrial system; however, the vast majority of this water is frozen in alpine glacial ice, snow packs that quickly ablate and ice-cemented permafrost. Though significant amounts of water are stored as ice, liquid water is available only at limited distances from those frozen sources during the short summer season when ice and snowmelt is possible (Barrett et al. Citation2009). For example, re-occurring glacial-fed meltwater streams are a major summer water resource (Fountain et al. Citation1999), where the underlying hyporheic zone is the site of high rates of biogeochemical cycling between soils and streams (Runkel et al. Citation1998; Nezat et al. Citation2001; Gooseff et al. Citation2002; Barrett et al. Citation2009). Biological communities found in these areas are distinct from those found in the dry soil (Treonis et al. Citation1999; Ayres et al. Citation2007). Snowmelt is also a potential source of water for soils. Though snowfall in the MDV is low (averaging 3–50 mm water-equivalent annual precipitation; Fountain et al. Citation2010), as much as 10% or more of MDV soil may be covered by snow patches accumulated in the winter months (Gooseff et al. Citation2003). Snow that covers the landscape in single storms (at most a few centimetres) rapidly sublimates, and only a minimal amount of water is transported to the soil (Campbell & Claridge Citation1982). However, the fate of the larger snow patches that collect during the fall, winter and spring is unknown though recent work suggests that some of this water is available in the underlying soil (Gooseff et al. Citation2003; Gooseff & Barrett unpubl. data) and may contribute to distinct water tracks or seeps, which form due to down slope movement of meltwater from some large snow patches, is being recognized as an important vector of salts across the landscape (Harris et al. Citation2007; Levy et al. Citation2011). Subnivian soils and those within water tracks are wetter than neighboring exposed soils, leading to greater mobilization of soil ions and altered habitat suitability for soil biota (Claridge et al. Citation1999; Courtright et al. Citation2001; Gooseff et al. Citation2003).
Biological and ecosystem processes in the MDV are constrained by the cold and dry climatic conditions. During the summer, when biological activity occurs, air temperatures in the MDV are at or near the freezing point, so the amount of meltwater is subject to small fluctuations in temperature (Ebnet et al. Citation2005). Thus, the MDV is a climate-sensitive system, where small changes in climate can greatly impact the hydrologic regime and therefore ecosystem processes (Fountain et al. Citation1999; Lyons et al. Citation2005; Barrett, Virginia, Wall & Adams Citation2008). Indeed, climate is recognized as a dominant factor in structuring the MDV over short-to-long time scales, with correlations between past climate legacies and nutrient supply, geochemistry, organic matter and biodiversity (Priscu Citation1995; Fountain et al. Citation1999; Burkins et al. Citation2000; Lyons et al. Citation2000; Wall Citation2007). Given the potential for small changes in climate to have amplified influences on biological and ecosystem processes in polar regions (Chapin et al. Citation2005; Montes-Hugo et al. Citation2009), the MDV may therefore be near a tipping point (Wall Citation2007), where the projected changes in climate may have profound effects on biological communities and biogeochemical cycling, especially if stored sources of water as ice or permafrost melt.
In recent decades, the MDV have experienced periodic discrete melt events, characterized by short intervals of above-average summer temperatures that result in meltwater pulses from dry valley ice reserves (Lyons et al. Citation2005; Barrett, Virginia, Wall & Adams 2008; Doran et al. Citation2008). Notably, these summer warming events have been followed by a return to baseline temperatures the following season. For example, through MCM LTER monitoring, we have observed two recent warming events (the summers of 2001/02 and 2008/09 [e.g., Foreman et al. Citation2004; Lyons et al. Citation2005]), and there is evidence of decadal events prior to MCM LTER (McKnight et al. Citation1999). These events, lasting a period of days to weeks, contribute to an increased extent of wet habitats via expansion of existing water sources (e.g., increased streamflow and lake levels due to enhanced glacial melt [Doran et al. Citation2008]), or the creation of transient new patches of inundated soil habitat from subsurface sources (Lyons et al. Citation2005; Wall Citation2007). The influence of melt pulses differs with the source of water and its interaction with local topography. If wetted margins and hyporheic zones around water bodies expand, their role as important biological and biogeochemical exchange sites will increase (; Barrett et al. Citation2009). Enhanced melting rates of snowfall and wind-blown snow patches will also increase water availability (). Increases in radiation balance and resulting increases in air temperatures may cause melt of subsurface ice (Harris et al. Citation2007), such as the ice-cemented permafrost underlying the majority of soils in the dry valleys (Bockheim Citation2002). With melt of subsurface ice, there is both the appearance of highly variable, discontinuous seep patches scattered across the landscape () and the re-activation of relict stream channels and gullies (; Lyons et al. Citation2005; Levy et al. Citation2011). These pulses alter soil geochemistry by redistributing major ions (Lyons et al. Citation2005; Harris et al. Citation2007) and transport soil sediments to streams and lakes (Foreman et al. Citation2004). Alterations to soil moisture and geochemistry constitute a change in habitat suitability that can alter soil invertebrate and microbial community abundance, composition and distribution (Powers et al. Citation1998; Nkem, Virginia et al. Citation2006; Barrett, Virginia, Wall & Adams 2008; Poage et al. Citation2008; Simmons et al. Citation2009; Ayres et al. Citation2010; Takacs-Vesbach et al. Citation2010). Given that soil invertebrate communities exhibit similar patterns to soil biogeochemical properties across the MDV (Barrett et al. Citation2004), the alterations to biological communities as a response to water pulses may have repercussions for the biogeochemical processes for which they are responsible (e.g., production, respiration and mineralization of nutrients). Though the recent trend has been for climate cooling (Doran et al. Citation2002) interspersed by warm summers characterized by discrete melt events (Barrett, Virginia, Wall & Adams 2008; Doran et al. Citation2008), future projections suggest that, as stratospheric ozone levels recover and the Southern Annular Mode diminishes, continental Antarctica will exhibit climate warming in step with modal global warming over the next 50–100 years (Chapman & Walsh Citation2007; Walsh Citation2009). Under this scenario or future, the MDV will experience a long-term press in increased temperatures (and therefore melt) with resulting changes in the structure and functioning of soil communities and ecosystems (Wall Citation2007). As the water pulses that result from the discrete warm years may presage the influence of this projected climate warming, it is important to understand the net influence of meltwater pulses and the mechanisms by which they influence soil biology and biogeochemical cycling.
Fig. 1 With climatic warming in a polar desert such as the McMurdo Dry Valleys of Antarctica, frozen reserves will melt to release pulses of water. A combination of water source (such as glacial, permafrost or snowmelt) and local topography (such as slope) will influence the directional movement of that water (arrows 1–3) and its influence on key soil ecosystem properties. The lower box depicts the predicted direction of changes caused by increased melt (arrows 1–3) on landscape and temporal heterogeneity, ion presence and concentration in the active layer, and biological (microbial and invertebrate) activity.
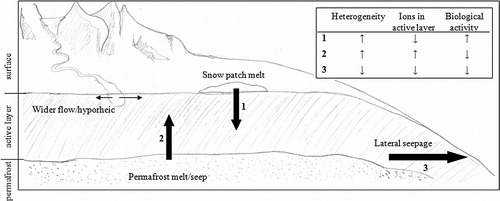
There is much uncertainty about the net effect of increased temperature and available water on soil biology and biogeochemistry in the MDV. For example, increased hydrologic activity has the potential to either increase spatial heterogeneity of soil biology and biogeochemistry (e.g., the scattered appearance of meltwater seeps and water tracks that alter soil ion content) or decrease soil heterogeneity (e.g., if leaching more evenly redistributes soil solutes and organisms). There is potential for biogeochemical cycling to become more connected across the landscape as newly-inundated environments coalesce to cause the landscape to become more uniform in moisture. Uniformly increased soil moisture may alter habitat suitability across the landscape and will therefore potentially result in altered distribution of taxa (Courtright et al. Citation2001). However, the response of the biota responsible for biogeochemical cycling to increased moisture is complex and not necessarily unidirectional. For example, increasing moisture tends to support more diverse invertebrate communities (Treonis et al. Citation1999; Ayres et al. Citation2007), but the most abundant and widely distributed of the higher invertebrate species (Nematoda: S. lindsayae) is dominant in drier soil. Microbes are also more diverse and abundant in drier soils (Takacs-Vesbach et al. Citation2010). Overall, the net responses of the soil ecosystem to water pulses will depend upon the water source and resulting movement of water in or across the landscape (). Here, we review the current knowledge of meltwater pulses and present new data from several examples of observed meltwater pulse events to discuss their influence on soil biology and biogeochemistry. We use these examples to discuss the potential impacts of future climate change on the MDV soil ecosystem.
The consequences of water pulses for soil biology and biogeochemistry
Responses due to infiltration (top down)
Increased air temperature in the MDV will lead to increased melt rates from glacial ice and accumulated snow packs. Concurrently, climate change projections suggest a windier MDV with more snow delivery from the interior of the continent, leading to additional sources of snow (Doran et al. Citation2008). Together, increased snow accumulation and melt rate will result in a greater volume of top-down water movement to the soil (i.e., infiltration; ) that may enhance the influence that snow packs have on soil biology and biogeochemistry (e.g., Ayres et al. Citation2010).
The moisture provided by snow packs influences the vertical and lateral transport of salts in soil (Claridge et al. Citation1999). Greater mobilization of salts may result in short-term reductions in nutrient levels in the soil as ions are transported away by the meltwater. For example, measurements of electrical conductivity (an integrative measure of soil ion content) through the soil profile from both within an area covered by a recently-ablated snow patch and outside that snow patch in the dry soil reveal consistently lower conductivity inside the patch (). Lower conductivity inside the area affected by the snow patch suggests that the meltwater had largely transported soil ions away from the active layer beneath the patch. Preliminary data suggest that transported salts are frequently found in a margin of variable size around the snowpack (Gooseff & Barrett unpubl. data), and distant from snowpacks (Levy et al. Citation2011). Therefore, increased snowmelt from larger or more numerous snow patches could lead to increased heterogeneity across the landscape, such that soil conditions are homogenized within the subnivian areas but surrounded by a zone of high salt content that increases spatial variation. Subnivian soils host larger, more diverse soil invertebrate communities, illustrating that certain species prefer these habitat conditions below the snowpack (Gooseff et al. Citation2003). Should the volume of snowmelt increase, altered habitat suitability could promote heterotrophic production over a greater portion of the landscape. We anticipate that pulses of infiltration from snowmelt will therefore be accompanied by pulses of biological abundance and shifts in species composition, as well as biogeochemical processes for which they are responsible. In experiments simulating infiltration pulses, we have observed that short-term water applications do not result in immediate alterations to invertebrate communities (Simmons et al. Citation2009), but sustained alterations in water sources applied at the surface of soils can result in shifts in species composition (Ayres et al. Citation2010). Therefore, duration and frequency of the water pulses are expected to influence the net impact on biology and biogeochemistry. In response to infiltration pulses of a longer duration (such as large snow packs that consistently re-occur each year), we anticipate that the loss of soil ions due to water transport will be followed by a longer-term increase in microbial and invertebrate production that may result in enhanced soil C and nutrient availability via a gradual increase in organic matter, which is characteristically low in the MDV, causing water pulses to be accompanied by nutrient pulses. Given that in situ stoichiometry varies based on geology and landscape history (Barrett et al. Citation2007; Bate et al. Citation2008), the response to nutrient pulses would be expected to vary across the MDV.
Fig. 2 Electrical conductivity with increasing depth in the soil profile measured inside (filled circles) and outside (open circles) one area previously covered by a snow patch. Conductivity was measured at increasing depths in the soil profile to the depth of resistance using a 5:1 water: soil slurry for each sampling depth.
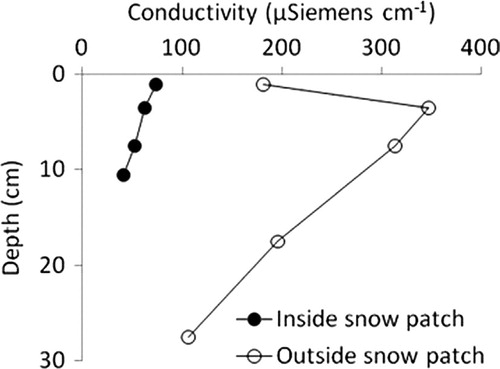
In addition to inter-annual influences, the duration of the water pulse will also likely affect within-season variations in soil biology and biogeochemistry. In a field manipulation simulating top-down water pulses, two sets of plots were established: one set (n=24) that received a one-time pulse of 12.7 L H2O m−2 (short-duration) to bring the upper 10 cm of soil to field capacity and one set (n=24) that received a weekly re-application of the same water pulse (long-duration). Soil carbon dioxide (CO2) flux was monitored from all plots to track the response of soil respiration (an integrative measure of soil biotic activity) to water pulse duration (). At day 0, prior to the pulse, soil moisture and CO2 flux were approximately equal in both sets of plots, and soil temperatures were relatively high resulting in CO2 flux levels typical for summer in the MDV (Parsons et al. Citation2004; Ball et al. Citation2009). Two and a half weeks after the initial pulse, temperature dropped significantly, and while the long-duration pulse was maintaining soil moisture at an elevated level, the short-duration plot had dried to low ambient levels. As a result of the higher moisture at lower temperatures, the long-duration plots exhibited a physically-determined negative CO2 flux (absorption of CO2 in soil water), rather than the low, positive rates (suggesting higher rates of respiration) observed in the short-duration plots experiencing dry, cold conditions (Temperature*Duration P=0.002 and Moisture P=0.004). The interaction of pulse duration with climate (e.g., temperature) suggests potential consequences for the carbon (C) cycle by altering the direction of influence of the dominant physical factors controlling soil CO2 flux. For example, longer residence time of soil water from such pulses may result in more negative fluxes of CO2 during cold periods of the day that may counterbalance any increased biotic release of CO2 via enhanced habitat suitability.
Fig. 3 Soil moisture, temperature and CO2 flux prior and 18 days after an artificial top-applied water pulse, where the water pulse was either applied once (short duration) or reapplied weekly (long-duration). Day 0, prior to the pulse, soil moisture and CO2 flux were approximately equal between both sets of plots (n=24 for each), and soil temperatures were relatively high, resulting in higher CO2 flux. By day 18, temperatures had dropped significantly, and while the long-duration pulse was maintaining soil moisture at an elevated level, the short-duration plot had dried back to natural levels. As a result of the higher moisture at lower temperatures, the long-duration plots exhibited negative CO2 flux, rather than the low, positive rates observed in the short-duration plots. Running an ANCOVA, with both temperature and moisture at each replicate plot as covariates, reveals a significant interaction of Temperature and Pulse duration (P=0.002) and a significant main effect of Moisture (P=0.004).
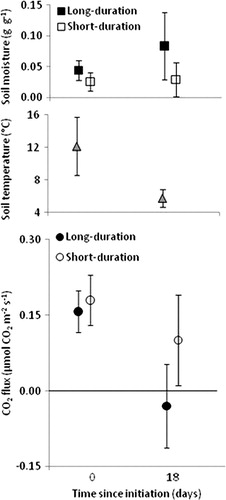
Responses to seeps
During warm events, water from the melt of subsurface ice, particularly the ice-cemented permafrost, is drawn through the active layer to the surface by capillary action (i.e., seepage), causing the appearance of variably-sized wetted patches scattered across the landscape (). Due to a lack or limited shallow groundwater movement prior to seep melt, salts can accumulate at the permafrost boundary until they are mobilized by the meltwater (Claridge et al. Citation1997). We have observed that the upward movement of water in seep patches is accompanied by an increase in water-soluble ion content in the active layer (). Principal components analysis (PCA) of the content of major cations and anions throughout the soil profile inside and outside of a seep patch demonstrate that the upper soil layers (0–2 cm, and sometimes to 5 cm) are indeed higher in ion content within the seep compared to soils outside (also supported by Levy et al. Citation2011). The deeper depths (5 cm to point of refusal) are homogeneously low in ion concentration both inside and outside the seep patch. The ions found in the upper layers inside the seep patch appear to have originated in or at the boundary with the permafrost itself, as there is no evidence of a build-up of ions at the lower depths in soils not affected by meltwater seepage (outside the patch), suggesting the active layer is not the source of the ions. In fact, ion content tends to be low at all depths outside the seep patches, suggesting that an increase in seep patch formation will lead to an increase in soil landscape heterogeneity in terms of both moisture and geochemistry.
Fig. 4 Principal components analysis using ion data measured in soil samples taken at increasing depth in the soil profile (depths 1–4, with 1 being the upper 2 cm of the soil and 4 being just above the depth of resistance) from two soil pits inside a seep patch and two outside a seep patch. Symbols represent individual soil samples, where circles denote samples from inside the seep patch and squares denote samples outside the seep patch. Numbers refer to sampling depth (1–4), where Depth 1 is a surface sample and Depth 4 is just above the depth of resistance. Letters (a and b) refer to the two replicate pits dug at each position. Arrows represent the ion species that drive the principal components axes.
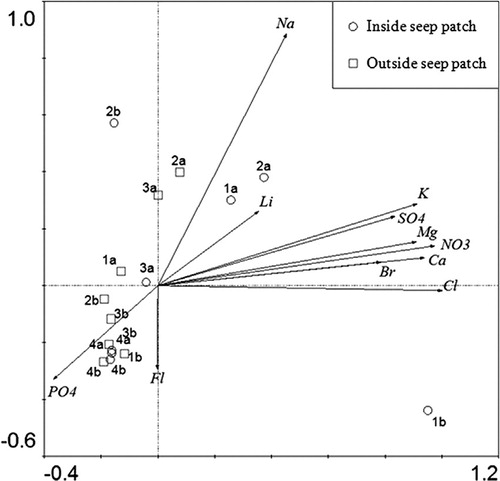
Though increased occurrence of seep patches will provide higher soil moisture that could potentially increase biotic activity across the MDV landscape, the increase in soil salt content will likely have an adverse affect on biotic activity in the upper layers of the soil (Nkem, Virginia et al. Citation2006; Poage et al. Citation2008). Depths 1 and 2 () represent the upper 5 cm of the soil, where the majority of invertebrate activity occurs (Powers et al. Citation1995). The osmotic stress imposed by the very high salt content means high-salinity soils exhibit very low or non-existent invertebrate abundances, despite the fact that many of these ions are mineral nutrients necessary for life (Nkem, Virginia et al. Citation2006; Poage et al. Citation2008). However, preliminary respiration measurements within and outside of seep patches reveals that seep patches are not always associated with a decrease in soil respiration (CitationBall &Virginia unpubl. ms.). Responses of the biological community (e.g., microbial vs. invertebrate) likely depend on a combination of factors, as not all species have the same threshold for salinity, moisture and organic matter resources (Courtright et al. Citation2001).
Responses to lateral water movement
Where topography routes permafrost and/or snow meltwater down slope over the ice table, we see the lateral movement of water in the absence of well-defined channels, such as through sub-surface water flow paths (i.e., water tracks) or above-surface streams (Lyons et al. Citation2005; Levy et al. Citation2011; ). A dramatic example is that of an episodic meltwater channel – provisionally called Wormherder Creek – on the south side of the west lobe of Lake Bonney, which has re-activated only recently during discrete warm years as the result of snow pack and permafrost melt (Lyons et al. Citation2005). Such new or re-activated streams create fresh, new terrestrial – aquatic interfaces (i.e., wetlands), which are hotspots for biologic and biogeochemical exchange (Junk et al. Citation1989; Bardgett et al. Citation2001; Gooseff et al. Citation2002; Ayres et al. Citation2007; Barrett et al. Citation2009). We expect to observe accelerated movement of biota and nutrients across the landscape as a result. The seep water carries high loads of dissolved solutes, salts and limiting elements leached from the soil that are redistributed or deposited into Lake Bonney (Foreman et al. Citation2004; Lyons et al. Citation2005; Simmons et al. Citation2009). Sediments sampled from within the flow of the surface portion of the stream are significantly lower in conductivity than the surrounding un-affected soil at equivalent depths (). The leaching of mobile ions from the soil can contribute to the homogenization of a soil landscape that would otherwise vary more widely in ionic content (). The increased water and export of salts may improve habitat suitability for particular soil organisms; some taxa were stimulated by melting of permafrost and the re-activation of Wormherder Creek, while others declined (Barrett, Virginia, Wall & Adams 2008; Simmons et al. Citation2009). The MCM LTER is monitoring Wormherder Creek to reveal the long-term response of biology and biogeochemical cycling to such pulses.
Fig. 5 Soil conductivity in and around a re-activated stream fed by sub-surface ice melt along the entire stretch of the stream (from just upslope of the stream emergence to the lake shore). Lines denote conductivity along two transects in the dry soil bordering the stream on both the north (dashed) and south (solid) banks, representing the conductivity we would expect in the soil prior to the appearance of the stream. Diamonds represent point-samples taken from sediments within the re-activated stream, and demonstrate that most of the soil ions have been transported out of the soil. Distances were estimated from global positioning system coordinates for all sample points. All measurements within and outside the stream were made on surface soils (0–10 cm).
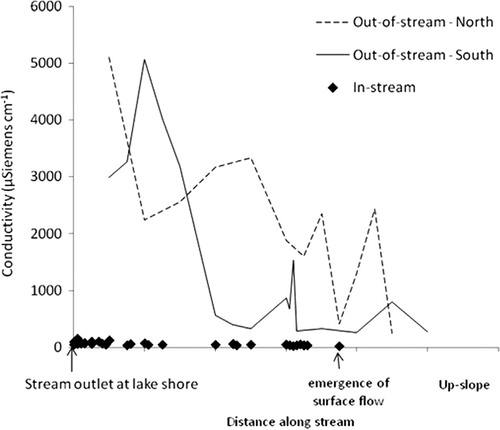
Similarly, subsurface water flow paths have been found to be a major pathway for movement of salts, with enhanced chemical weathering and leaching from the soil, and transport of those ions through the soil to the lakes in concentrations comparable to or sometimes in excess of glacial-fed streams (Levy et al. Citation2011). While the increased soil moisture will enhance habitat suitability for some species, the more saline portions of some water flow paths may be inhospitable to some species, resulting in an overall alteration of biotic communities.
The future of MDV soil biology and biogeochemistry
Observations and manipulative experiments clearly demonstrate that water pulse events influence MDV biology and biogeochemistry, particularly through alteration to geochemistry and therefore habitat suitability, distribution of biota and their functioning. Future projected changes in climate and moisture regimes will likely have a significant impact on the MDV soil ecosystem. Increased glacial melt will create consistently higher stream flow and lake levels, with increased surface area of associated hyporheic sediment-soil interfaces, that is, wetted margins (). Melting of snow and permafrost will increase the occurrence of surface and subsurface water flow, potentially creating new or re-activated relict wetlands. These newly-inundated areas have distinctively altered geochemistry (e.g., Lyons et al. Citation2005) and biological communities (e.g., Barrett, Virginia, Wall & Adams 2008; Simmons et al. Citation2009).
In addition to localized effects discussed in the sections above, water pulses should increase connectivity in biological distribution and biogeochemical cycles; given that water is a major vector of connectivity among ecosystem components in the MDV (Adams et al. Citation2007; Barrett et al. Citation2007). Increased movement of water should not only increase the amount of habitat for those species normally occurring in moist soils, but decrease the abundance of organisms better adapted to the prevailing arid, saline soils such as the present dominant invertebrate: the nematode, S. lindsayae (Wall Citation2007). Water dispersal of organisms will be enhanced transporting organisms among newly-connected landscape components, and thereby influencing biogeochemical cycling (Nkem, Wall et al. Citation2006; McKnight et al. Citation2007; Barrett, Virginia, Wall & Adams 2008). Additionally, more soils will become connected, and likely for longer periods of time, with other ecosystem components, as the new hydrologic regime will be transporting materials from soils through streams and ultimately to the lakes (e.g., Foreman et al. Citation2004).
Notably, responses of soil ecosystems to water pulses are not unilateral or unidirectional. The net effects will depend on the source and movement of water, which is a product of local geography and landscape development (e.g., topography). We may see greater “de-salinization” of the soil as salts are transported out of the active layer and into the lakes, which would result in a homogenization of the soil landscape, as in the case of lateral water movement through water flow paths (). Alternatively, we may see heterogeneous increases in soil salinity as the result of bottom-up water movement in the case of permafrost seep patches ().
There is much left to be learned to better understand what the overall consequences will be for the MDV. Through a combination of monitoring, experimentation and modelling, the MCM LTER is investigating the variety of water pulses to understand their impacts on terrestrial Antarctic ecosystem diversity and function and polar deserts more generally. Such research will provide important insight into whether, and if so how, climate warming will amplify connectivity and heterogeneity of biological communities and nutrient cycling across the landscape.
Acknowledgements
We thank the editors for the opportunity to be a part of this special issue resulting from the International Polar Year Oslo Science Conference 2010. We thank the Research Council of Norway and the Dickey Center for International Understanding and its Institute of Arctic Studies at Dartmouth College for travel funding that made it possible to attend the conference. The focus of this paper evolved from discussion among members of MCM LTER, in particular WBL and ANF, and was funded by National Science Foundation grant no. ANT-0423595.
References
- Adams B.J. Wall D.H. Gozel U. Dillman A.R. Chaston J.M. Hogg I.D. The southernmost worm, Scottnema lindsayae (Nematoda): diversity, dispersal and ecological stability. Polar Biology. 2007; 30: 809–815.
- Ayres E. Nkem J.N. Wall D.H. Adams B.J. Barrett J.E. Simmons B.L. Virginia R.A. Fountain A.G. Experimentally increased snow accumulation alters soil moisture and animal community structure in a polar desert. Polar Biology. 2010; 33: 897–907.
- Ayres E. Wall D. Adams B. Barrett J. Virginia R. Unique similarity of faunal communities across aquatic–terrestrial interfaces in a polar desert ecosystem. Ecosystems. 2007; 10: 523–535.
- Ball B.A. & Virginia R.A. unpubl. ms. Permafrost melt seep patches increase heterogeneity of soil geochemistry and therefore habitat suitability.
- Ball B.A. Virginia R.A. Barrett J.E. Parsons A.N. Wall D.H. Interactions between physical and biotic factors influence CO2 flux in Antarctic dry valley soils. Soil Biology and Biochemistry. 2009; 41: 1510–1517.
- Bardgett R.D. Anderson J.M. Behan-Pelletier V. Brussaard L. Coleman D.C. Ettema C. Moldenke A. Schimel J.P. Wall D.H. The influence of soil biodiversity on hydrological pathways and the transfer of materials between terrestrial and aquatic ecosystems. Ecosystems. 2001; 4: 421–429.
- Barrett J.E. Gooseff M.N. Takacs-Vesbach C. Spatial variation in soil active-layer geochemistry across hydrologic margins in polar desert ecosystems. Hydrology and Earth System Sciences. 2009; 13: 2349–2358.
- Barrett J.E. Virginia R.A. Hopkins D.W. Aislabie J. Bargagli R. Bockheim J.G. Campbell I.B. Lyons W.B. Moorhead D.L. Nkem J.N. Sletten R.S. Steltzer H. Wall D.H. Wallenstein M.D. Terrestrial ecosystem processes of Victoria Land, Antarctica. Soil Biology and Biochemistry. 2006; 38: 3019–3034.
- Barrett J.E, Virginia R.A, Lyons W.B, McKnight D.M, Priscu J.C, Doran P.T, Fountain A.G, Wall D.H. & Moorhead D.L. 2007. Biogeochemical stoichiometry of Antarctic Dry Valley ecosystems. Journal of Geophysical Research—Biogeosciences. 112, G01010, 10.3402/polar.v30i0.14555.
- Barrett J.E. Virginia R.A. Wall D.H. Adams B.J. Decline in a dominant invertebrate species contributes to altered carbon cycling in a low-diversity soil ecosystem. Global Change Biology. 2008; 14: 1734–1744.
- Barrett J.E. Virginia R.A. Wall D.H. Doran P.T. Fountain A.G. Welch K.A. Lyons W.B. Persistent effects of a discrete warming event on a polar desert ecosystem. Global Change Biology. 2008; 14: 2249–2261.
- Barrett J.E. Virginia R.A. Wall D.H. Parsons A.N. Powers L.E. Burkins M.B. Variation in biogeochemistry and soil biodiversity across spatial scales in a polar desert ecosystem. Ecology. 2004; 85: 3105–3118.
- Bate D.B. Barrett J.E. Poage M.A. Virginia R.A. Soil phosphorus cycling in an Antarctic polar desert. Geoderma. 2008; 144: 21–31.
- Bernstein L., Bosch P., Canziani O., Chen Z., Christ R., Davidson O., Hare W., Huq S., Karoly D., Kattsov V., Kundzewicz Z., Liu J., Lohmann U., Manning M., Matsuno T., Menne B., Metz B., Mirza M., Nicholls N., Nurse N., Pachauri R., Palutikof J., Parry M., Qin D., Ravindranath N., Reisinger A., R.Jiawen, Riahi K., Rosenzweig C., Rusticucci M., Schneider S., Sokona Y., Solomon S., Stott P., Stouffer R., Sugiyama T., Swart R., Tirpak D., Vogel C., Yohe G. & Reisinger A. 2007. Climate change 2007: synthesis report. Contribution of Working Groups I, II and III to the Fourth Assessment Report of the Intergovernmental Panel on Climate Change. Geneva: Intergovernmental Panel on Climate Change.
- Bockheim J.G. Landform and soil development in the McMurdo Dry Valleys, Antarctica: a regional synthesis. Arctic Antarctic and Alpine Research. 2002; 34: 308–317.
- Bockheim J.G. Campbell I.B. McLeod M. Permafrost distribution and active-layer depths in the McMurdo Dry Valleys, Antarctica. Permafrost and Periglacial Processes. 2007; 18: 217–227.
- Burkins M.B. Virginia R.A. Chamberlain C.P. Wall D.H. Origin and distribution of soil organic matter in Taylor Valley, Antarctica. Ecology. 2000; 81: 2377–2391.
- Burkins M.B. Virginia R.A. Wall D.H. Organic carbon cycling in Taylor Valley, Antarctica: quantifying soil reservoirs and soil respiration. Global Change Biology. 2001; 7: 113–125.
- Campbell I.B. Claridge G.G.C. The influence of moisture on the development of soils in the cold deserts of Antarctica. Geoderma. 1982; 28: 221–238.
- Campbell I.B. Claridge G.G.C. Antarctica: soils, weathering processes and environment. Elsevier. New York, 1987
- Campbell I.B. Claridge G.G.C. Balks M.R. Campbell D.I. Moisture content in soils of the McMurdo Sound and Dry Valley region of Antarctica. Ecosystem processes in Antarctic ice-free landscapes. Lyons W.B. et al.. A.A. Balkema. Rotterdam, 1997; 61–76.
- Chapin F.S. Sturm M. Serreze M.C. McFadden J.P. Key J.R. Lloyd A.H. McGuire A.D. Rupp T.S. Lynch A.H. Schimel J.P. Beringer J. Chapman W.L. Epstein H.E. Euskirchen E.S. Hinzman L.D. Jia G. Ping C.L. Tape K.D. Thompson C.D.C. Walker D.A. Welker J.M. Role of land-surface changes in Arctic summer warming. Science. 2005; 310: 657–660.
- Chapman W.L. Walsh J.E. A synthesis of Antarctic temperatures. Journal of Climate. 2007; 20: 4096–4117.
- Claridge G.G.C. Campbell I.B. Balks M.R. Ionic migration in soils of the Dry Valley region. Ecosystem processes in Antarctic ice-free Landscapes. Lyons W.B. et al.. A.A. Balkema. Rotterdam, 1997; 137–143.
- Claridge G.G.C. Campbell I.B. Balks M.R. Movement of salts in Antarctic soils: experiments using lithium chloride. Permafrost and Periglacial Processes. 1999; 10: 223–233.
- Convey P. Block W. Peat H.J. Soil arthropods as indicators of water stress in Antarctic terrestrial habitats?. Global Change Biology. 2003; 9: 1718–1730.
- Courtright E.M. Wall D.H. Virginia R.A. Determining habitat suitability for soil invertebrates in an extreme environment: the McMurdo Dry Valleys, Antarctica. Antarctic Science. 2001; 13: 9–17.
- Doran P.T. McKay C.P. Fountain A.G. Nylen T. McKnight D.M. Jaros C. Barrett J.E. Hydrologic response to extreme warm and cold summers in the McMurdo Dry Valleys, East Antarctica. Antarctic Science. 2008; 20: 499–509.
- Doran P.T. Priscu J.C. Lyons W.B. Walsh J.E. Fountain A.G. McKnight D.M. Moorhead D.L. Virginia R.A. Wall D.H. Clow G.D. Fritsen C.H. McKay C.P. Parsons A.N. Antarctic climate cooling and terrestrial ecosystem response. Nature. 2002; 415: 517–520.
- Ebnet A.F. Fountain A.G. Nylen T.H. McKnight D.M. Jaros C.L. A temperature-index model of stream flow at below-freezing temperatures in Taylor Valley, Antarctica. Annals of Glaciology. 2005; 40: 76–82.
- Foreman C.M. Wolf C.F. Priscu J.C. Impact of episodic warming events on the physical, chemical and biological relationships of lakes in the McMurdo Dry Valleys, Antarctica. Aquatic Geochemistry. 2004; 10: 239–268.
- Fountain A.G. Lyons W.B. Burkins M.B. Dana G.L. Doran P.T. Lewis K.J. McKnight D.M. Moorhead D.L. Parsons A.N. Priscu J.C. Wall D.H. Wharton R.A. Virginia R.A. Physical controls on the Taylor Valley ecosystem, Antarctica. Bioscience. 1999; 49: 961–971.
- Fountain A.G. Nylen T.H. Monaghan A. Basagic H.J. Bromwich D. Snow in the McMurdo Dry Valleys, Antarctica. International Journal of Climatology. 2010; 30: 633–642.
- Freckman D. Virginia R.A. Soil biodiversity and community structure in the McMurdo Dry Valleys, Antarctica. Ecosystem dynamics in a polar desert: the McMurdo Dry Valleys, Antarctica. Priscu J.C. American Geophysical Union. Washington D.C, 1998; 323–335.
- Friedmann E.I. Kappen L. Meyer M.A. Nienow J.A. Long-term productivity in the cryptoendolithic microbial community of the Ross Desert, Antarctica. Microbial Ecology. 1993; 25: 51–69.
- Gooseff M.N. Barrett J.E. Doran P.T. Fountain A.G. Lyons W.B. Parsons A.N. Porazinska D.L. Virginia R.A. Wall D.H. Snow-patch influence on soil biogeochemical processes and invertebrate distribution in the McMurdo Dry Valleys, Antarctica. Arctic Antarctic and Alpine Research. 2003; 35: 91–99.
- Gooseff M.N. McKnight D.M. Lyons W.B. Blum A.E. Weathering reactions and hyporheic exchange controls on stream water chemistry in a glacial meltwater stream in the McMurdo Dry Valleys. Water Resources Research. 2002; 38: 17.
- Harris K.J. Carey A.E. Lyons W.B. Welch K.A. Fountain A.G. Solute and isotope geochemistry of subsurface ice melt seeps in Taylor Valley, Antarctica. Geological Society of America Bulletin. 2007; 119: 548–555.
- Hogg I.D. Cary S.C. Convey P. Newsham K.K. O”'Donnell A.G. Adams B.J. Aislabie J. Frati F. Stevens M.I. Wall D.H. Biotic interactions in Antarctic terrestrial ecosystems: are they a factor?. Soil Biology & Biochemistry. 2006; 38: 3035–3040.
- Junk W.J, Bayley P.B. & Sparks R.E. 1989. The flood pulse concept in river–floodplain systems. InD.P.Dodge: Proceedings of the International Large River Symposium. Canadian Special Publication of Fisheries and Aquatic Science106. Pp. 110–127. Ottawa: Department of Fisheries and Oceans.
- Levy J.S. Fountain A.G. Gooseff M.N. Welch K.A. Lyons W.B. Water tracks and permafrost in Taylor Valley, Antarctica: extensive and shallow groundwater connectivity in a cold desert ecosystem. Geological Society of America Bulletin. 2011; 123: 2295–2311.
- Lyons W.B. Fountain A. Doran P. Priscu J.C. Neumann K. Welch K.A. Importance of landscape position and legacy: the evolution of the lakes in Taylor Valley, Antarctica. Freshwater Biology. 2000; 43: 355–367.
- Lyons W.B. Welch K.A. Carey A.E. Doran P.T. Wall D.H. Virginia R.A. Fountain A.G. Csathó B.M. Tremper C.M. Groundwater seeps in Taylor Valley Antarctica: an example of a subsurface melt event. Annals of Glaciology. 2005; 40: 200–206.
- McKnight D.M. Niyogi D.K. Alger A.S. Bomblies A. Conovitz P.A. Tate C.M. Dry valley streams in Antarctica: ecosystems waiting for water. Bioscience. 1999; 49: 985–995.
- McKnight D.M. Tate C.M. Andrews E.D. Niyogi D.K. Cozzetto K. Welch K. Lyons W.B. Capone D.G. Reactivation of a cryptobiotic stream ecosystem in the McMurdo Dry Valleys, Antarctica: a long-term geomorphological experiment. Geomorphology. 2007; 89: 186–204.
- Montes-Hugo M. Doney S.C. Ducklow H.W. Fraser W. Martinson D. Stammerjohn S.E. Schofield O. Recent changes in phytoplankton communities associated with rapid regional climate change along the western Antarctic Peninsula. Science. 2009; 323: 1470–1473.
- Moorhead D.L. Doran P.T. Fountain A.G. Lyons W.B. McKnight D.M. Priscu J.C. Virginia R.A. Wall D.H. Ecological legacies: Impacts on ecosystems of the McMurdo Dry Valleys. Bioscience. 1999; 49: 1009–1019.
- Moorhead D.L. Priscu J.C. The McMurdo Dry Valley ecosystem: organization, controls, and linkages, in ecosystem processes in a polar desert: the McMurdo Dry Valleys, Antarctica. Ecosystem processes in a polar desert: the McMurdo Dry Valleys. Priscu J.C. American Geophysical Union. Washington D.C, 1998; 351–364.
- Moorhead D.L. Wall D.H. Virginia R.A. Parsons A.N. Distribution and life-cycle of Scottnema lindsayae (Nematoda) in Antarctic soils: a modeling analysis of temperature responses. Polar Biology. 2002; 25: 118–125.
- Nezat C.A. Lyons W.B. Welch K.A. Chemical weathering in streams of a polar desert (Taylor Valley, Antarctica). Geological Society of America Bulletin. 2001; 113: 1401–1408.
- Nkem J.N. Virginia R.A. Barrett J.E. Wall D.H. Li G. Salt tolerance and survival thresholds for two species of Antarctic soil nematodes. Polar Biology. 2006; 29: 643–651.
- Nkem J.N. Wall D.H. Virginia R.A. Barrett J.E. Broos E.J. Porazinska D.L. Adams B.J. Wind dispersal of soil invertebrates in the McMurdo Dry Valleys, Antarctica. Polar Biology. 2006; 29: 346–352.
- Parsons A.N. Barrett J.E. Wall D.H. Virginia R.A. Soil carbon dioxide flux in Antarctic dry valley ecosystems. Ecosystems. 2004; 7: 286–295.
- Poage M.A. Barrettt J.E. Virginia R.A. Wall D.H. The influence of soil geochemistry on nematode distribution, McMurdo Dry Valleys, Antarctica. Arctic Antarctic and Alpine Research. 2008; 40: 119–128.
- Powers L.E. Freckman D.W. Virginia R.A. Spatial distribution of nematodes in polar desert soils of Antarctica. Polar Biology. 1995; 15: 325–333.
- Powers L.E. Ho M.C. Freckman D.W. Virginia R.A. Distribution, community structure, and microhabitats of soil invertebrates along an elevational gradient in Taylor Valley, Antarctica. Arctic and Alpine Research. 1998; 30: 133–141.
- Priscu J.C. Phytoplankton nutrient deficiency in lakes of the McMurdo Dry Valleys, Antarctica. Freshwater Biology. 1995; 34: 215–227.
- Runkel R.L. McKnight D.M. Andrews E.D. Analysis of transient storage subject to unsteady flow: diel flow variation in an Antarctic stream. Journal of the North American Benthological Society. 1998; 17: 143–154.
- Simmons B.L. Wall D.H. Adams B.J. Ayres E. Barrett J.E. Virginia R.A. Long-term experimental warming reduces soil nematode populations in the McMurdo Dry Valleys, Antarctica. Soil Biology and Biochemistry. 2009; 41: 2052–2060.
- Steig E.J. Schneider D.P. Rutherford S.D. Mann M.E. Comiso J.C. Shindell D.T. Warming of the Antarctic ice-sheet surface since the 1957 International Geophysical Year. Nature. 2009; 457: 459–U454.
- Takacs-Vesbach C. Zeglin L. Gooseff M.A. Barrett J.E. Priscu J.C. Factors promoting microbial diversity in the McMurdo Dry Valleys. Life in Antarctic deserts and other cold dry environments: astrobiological analogues. Doran P. et al.. Cambridge University Press. Cambridge, 2010; 221–257.
- Treonis A.M. Wall D.H. Virginia R.A. Invertebrate biodiversity in Antarctic dry valley soils and sediments. Ecosystems. 1999; 2: 482–492.
- Virginia R.A. Wall D.H. How soils structure communities in the Antarctic dry valleys. Bioscience. 1999; 49: 973–983.
- Wall D.H. Global change tipping points: above- and below-ground biotic interactions in a low diversity ecosystem. Philosophical Transactions of the Royal Society B. 2007; 362: 2291–2306.
- Walsh J.E. A comparison of Arctic and Antarctic climate change, present and future. Antarctic Science. 2009; 21: 179–188.