Abstract
Bionanotechnology blends the areas of nanotechnology and biological sciences. This article surveys the area of nanoscale biosensor development and the application of state-of-the-art biophysical techniques to elucidate fundamental membrane and surfactant biochemistry undertaken at the University of Waterloo (Canada). The Waterloo Institute for Nanotechnology (WIN), coordinates various cutting-edge nanotechnology research areas at the University of Waterloo. One focus is the area of biosensor research and fundamental biophysics in bionanotechnology. This is Part 2 of a contribution from the WIN initiative.
Biosensors & medical diagnostics
The Waterloo Institute for Nanotechnology (WIN) at the University of Waterloo (Ontario, Canada) conducts research in the area of bionanotechnology, as seen in Part 1 Citation[1]. A number of research groups associated with WIN have interests in novel biosensors based on nanomaterial scaffolds. The research group of Juewen Liu focuses on DNA bionanotechnology. The characteristic double-helix structure of DNA and its biological function has long been studied by many researchers. Over the last 20 years, many new and exciting properties of DNA have been discovered, allowing DNA to play an increasingly important role in many other fields. For example, first, in addition to proteins and RNA, DNA has also been shown to have catalytic properties and as such can be termed a deoxyribozyme. Second, ssDNA with certain sequences can bind essentially to any molecule of choice and these DNA ligands are called aptamers. Third, the programmable structure of DNA has allowed it to be a building block for the preparation of novel nanostructures, such as DNA-functionalized inorganic nanoparticles, allowing these hybrid materials to be used for sensing, drug delivery and imaging applications. Research in the Liu laboratory explores the property and function of DNA in the aforementioned areas for biological, medicinal and nanotechnology applications. One particular research program is related to the detection of metabolites in human blood serum. While diagnosis of disease can be determined by examining DNA, RNA and proteins, various studies have shown that metabolites can also be used as disease biomarkers in blood serum. The advantages of metabolites are their greater stability and higher concentration compared with proteins and nucleic acids. Current methods for metabolite detection include instrumentation techniques such as MS and NMR. An alternative detection method has been to use aptamers to selectively bind metabolites and a number of fluorescent sensors have been developed. In the Liu laboratory, new methods to obtain high-affinity aptamers for the binding of selective metabolites in blood serum are being developed. The ATP aptamer, selected by Szostak and co-workers 15 years ago (), can bind two ATP molecules to yield a well-defined structure. For detection of metabolites in serum, challenges include the optical background of serum and a low sample volume. Therefore, by immobilizing fluorescent aptamer sensors on magnetic beads, the Liu laboratory has developed a flow cytometry-based sensor that is capable of detecting ATP in as low as 10 µl serum samples () Citation[2]. The ultimate goal is to develop a multiplexed assay for simultaneous detection of a group of metabolites related to a specific disease for more precise disease diagnosis. At the same time, the group is using DNA to perform programmable assembly of nanomaterials. One example is to prepare hybrid materials containing both soft (liposomes) and hard (gold) nanoparticles. Gold nanoparticles are useful for imaging applications, while liposomes can contain therapeutic agents. These materials possess the advantages of both particles, allowing applications for drug delivery, imaging and biosensing.
(B) Selection of aptamers for metabolite binding. An ATP aptamer and its target binding is shown. (A) Selected aptamers can be used for assembly of advanced nanomaterials such as liposome–gold nanoparticle hybrids. (C) Aptamers can also be made into highly sensitive biosensors.
F: Fluorophore; MMP: Magnetic microparticle; Q: Quencher.
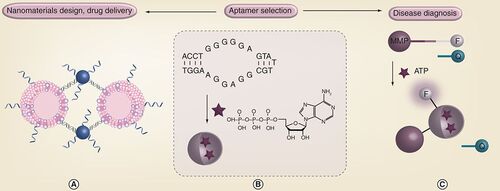
The Vivek Maheshwari group focuses on different approaches to nanosensor design utilizing intact cells . Electrical circuits are primarily driven by electrons as the charge carriers. The modern day elements of our society such as transistors, sensors and energy generation and delivery devices (directly or indirectly) are based on electrons and electric fields. In contrast, being based in an aqueous environment cells are limited in their ability to directly use electrons. Ions, small molecules and enzymes form the basis of cellular processes that provide the complementary functionality of sensors, energy generation and storage and information gathering and processing. Research into the integration of cells with electrical circuits has recently generated interest with applications in biomedical sensors and the study of whole-cell responses in a controlled environment. It also opens up the possibility of integrating cellular components such as ion pumps, cell receptors and protein-synthesis machinery with external circuits that could perhaps both control and use these components to develop a new paradigm of cellular-electronic devices. The recent interfacing of an external electronic circuit with the photosynthesis cycle to directly harvest the electrons highlights the future possibilities Citation[3].
The cellular processes provide an impetus to the nanoparticles in the form of electrochemical modulation. The electronic interface, with a nanotip scanning electrode allows localized measurement of the cellular processes.
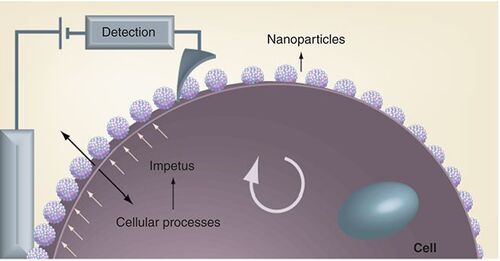
Cell integration with field-effect transistors (FETs) based on nanowires and nanotubes have been demonstrated as biochemical sensors Citation[4]. They have been able to detect electrochemical signals from neurons and cardiomyocyte cells Citation[5]. A parallel approach is to assemble an electronic device on the surface of the cell. Nanomaterials such as graphene, nanowires and self-assembled nanoparticles can be used for interfacing with the cell Citation[6]. These materials have quantum-scale characteristics to provide a sensitive interface for detection and measurement of cell properties. They also have the size to couple with the enzymes, ion pumps and cell receptors for integration with cellular machinery. Further developments in the area will hinge on research for new means of integration, robust operation and sustained functioning of the cellular electronic devices.
The Shirley Tang group utilizes the unique physical properties of nanomaterials, namely carbon nanotube (CNT), graphene and ZnO nanostructures, to obtain advanced sensory devices and biochips . In particular, the group is developing large-arrayed biosensors for multiplexed detection of biomolecules and pathogens that could lead to miniature bioanalytical devices for rapid diagnosis and field applications. One key challenge is the fabrication of highly reproducible nanosensors that could be readily integrated into complex electrical circuits and with microfluidic channels. To address this challenge, the Tang group looked at new synthetic methods to achieve site-selective growth of nanostructures and ordered architectures of nanomaterials on solid and flexible substrates. For example, a scalable method has been developed to prepare ultra-thin, homogeneous and transferable films of pristine CNTs. Such CNT films can be patterned lithographically and electrically contacted to make large arrays of highly uniform sensory devices on low-cost substrates such as glass and plastics, and potentially lead to wearable or flexible biosensors. Patterned growth of graphene sheets was obtained by a chemical vapor deposition method using predefined nickel film. With a facile and rapid microwave-assisted chemical method, flower-like ZnO nanostructures were directly synthesized and integrated into electrical circuits in a microfluidic compatible layout. With a platform of nanomaterial-based electrochemical- and optoelectrical-biosensors, broad and diversed applications are envisioned. Novel biofunctionalization techniques are being developed to couple nanomaterials with biological receptors (e.g., DNA oligonucleotide or antibody). Single-walled nanotube field-effect transistor (SWNT-FET) type biosensors have been developed for DNA and protein sensing. CNT thin film FET (CNT-TFT) and ZnO Schottky diode (ZnO-SD)-based biosensors are currently being explored for rapid identification of whole pathogens (e.g., bacteria or viruses).
(A) Optical image of a chip compared with a Canadian dime. (B) SEM image shows a set of devices on the chip with nanostructures bridging macroscopic metal electrodes. (C) and (D) Zoomed in SEM images of flower-like ZnO nanostructures and graphene, respectively.
SEM: Scanning electron microscopy.
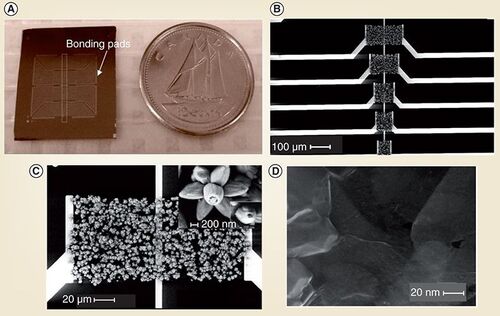
Scanning probe microscopy: biophysics & nanoscience
Research in Zoya Leonenko’s laboratory is focused on developing and using advanced scanning probe microscopy (SPM) techniques to study the biophysics of lipids and lipid–protein interactions; the role of structural changes and physical properties of lipid–protein assemblies in controlling biological processes and diseases. SPM is a large family of nanoscale imaging and manipulation techniques capable of probing local electrical, magnetic, chemical, mechanical, optical and thermal properties of matter at nanoscale levels, in addition to allowing the manipulation of these properties in a controlled manner. SPM is particularly useful in nanoscale biophysics research.
Most known SPM techniques include scanning tunneling microscopy (STM), atomic-force microscopy (AFM) and near-field scanning optical microscopy. STM was developed in the IBM laboratories by Binnig and Rorher in 1981 and went on to receive a Nobel Prize for this development in 1986. AFM was invented later to overcome the limitations of STM in biological applications Citation[7,101]. The principle of AFM imaging is to measure various forces, which cause the deflection of the cantilever with an attached scanning probe when the surface is scanned . These forces include van der Waals forces, electrostatic forces and other interactions.
(A) Principle of atomic force microscopy. (B) Atomic-force microscopy image of amyloid-β1–42 fibrils
(A) With permission from Citation[101] © JPK instruments.
(B) With permission from Choi Y and Leonenko Z (Leonenko’s group) [Unpublished Data].
![Figure 4. Principle of atomic force microscopy and an example atomic-force microscopy image. (A) Principle of atomic force microscopy. (B) Atomic-force microscopy image of amyloid-β1–42 fibrils(A) With permission from Citation[101] © JPK instruments.(B) With permission from Choi Y and Leonenko Z (Leonenko’s group) [Unpublished Data].](/cms/asset/0ba9f77a-0551-428e-a7fc-c132aef69bf5/ifmc_a_12362357_f0004.jpg)
Force measurements or atomic-force spectroscopy is a way of measuring interaction forces between the probe and the sample Citation[8]. These forces are used to characterize the properties of the sample. When the molecules of interest are attached to the AFM probe, specific interaction and binding energies can be investigated. AFM is also widely used in nanolithography, for nanomanipulation and in nano- and biosensor development. Multiple cantilever arrays, vibrating at specific frequencies, are used as extremely sensitive sensors to detect adsorption of a very small amount of substances to the cantilever surface. When modified with various specific self-assembled monolayers, they provide a wide variety of sensing approaches, from sensing the presence of toxic substances in the air to cancer detection.
Leonenko’s laboratory uses AFM, Kelvin probe force microscopy (KPFM), optical/fluorescence microscopy, single-molecule force spectroscopy and Langmuir-Blodgett monolayer techniques. KPFM is a type of SPM and is peformed by applying a voltage to a conducting AFM tip Citation[9]. The probe scans across the structure of interest and the electrostatic interaction is recorded at each point of the sample. It reveals a surface potential landscape at the same time as the surface topography is acquired.
The laboratory specifically studies the biophysical structure and function of lipid membrane and lipid rafts and their role in amyloid fibril formation in relation to Alzheimer’s disease. This study will pose an exciting new opportunity not only to understand the molecular mechanisms of biologically important processes, such as the interaction of proteins with lipid membrane, but also to target diseases such as Alzheimer‘s. This requires a detailed understanding of the nature of lipid rafts and mechanisms of their interaction with amyloid-forming proteins, which are associated with at least 20 diseases in addition to Alzheimer‘s, for which no cure is currently available Citation[10,11]. The AFM image in , shows amyloid β 1–42 fibrils formed in solution after 24 h of incubation. The group also showed that electrostatic interactions with chemical modified and lipid surfaces are important and affect the structure of amyloid fibrils.
Leonenko’s laboratory applies AFM and KPFM to study the structure and function of lung surfactant, which is a complex lipid–protein mixture covering the lung interface which reduced the effort in the breathing process. The group investigates how surfactant properties depend on surfactant composition, the presence of cholesterol and amyloid fibrils and how these features affect interaction of lung surfactant with nanoparticles Citation[12–14]. With Langmuir-Blodgett monolayer techniques the surfactant film is compressed at the air–water interface, and with AFM and KPFM, the research group showed that the surfactant film forms distinct multilayers. The structure of these multilayers shows significant variance in surface potential revealed by KPFM , which in turn affects the interaction of the lung surfactant with nanoparticles.
(A) Atomic-force microscopy images, (B) Kelvin force probe microscopy images and (C) Cross-section plot, animal lipid-extract surfactant film (bles) supported on mica.
Reprinted with permission from Citation[13] © Elsevier.
![Figure 5. Images obtained by atomic-force microscopy approaches (A) Atomic-force microscopy images, (B) Kelvin force probe microscopy images and (C) Cross-section plot, animal lipid-extract surfactant film (bles) supported on mica.Reprinted with permission from Citation[13] © Elsevier.](/cms/asset/e8da9ed7-b0e5-4b99-a59a-1798cdcc89e4/ifmc_a_12362357_f0005.jpg)
Future perspective
Improved methods to design and fabricate nanoscale biosensors will be developed over the next few years, allowing for increased sensitivity and selectivity to occur in these new devices. Improved understanding of how to effectively link detector molecules, such as proteins, aptamers and cells to nanomaterials, will allow for extremely selective biosensor development. Further research into proprietary nanoscale detection systems for high-throughput drug screening is also likely to impact future directions of drug discovery. The application of various cutting-edge biophysical techniques such as SPM will improve our knowledge of membrane biochemistry and its contribution to medical conditions, including various neuropathologies.
Deoxyribozyme
The ribozyme is a catalytic RNA-based enzyme. Deoxyribozyme is a DNA-based catalytic nucleic acid.
Aptamer
Peptides or oligonucleotides that exhibit an affinity to target molecules.
Nanotechnology
Technology that attempts to control matter at the atomic and/or molecular scale, which usually deals with molecular structures that are in the size region of 1–100 nm.
Field-effect transistors
Transistors that rely on an electrical field to control the conductivity of a channel in a semiconductor material.
Executive summary
Bottom-up creation of novel biosensors utilizing intact cells and proteins are being pursued.
Application of DNA- and RNA-based aptamers can provide ‘tailor-made’ drug and metabolite recognition elements for novel biosensors.
Bionanotechnology-based biosensors may provide new bases for high-throughput drug screening approaches, improving upon current methodologies.
Novel applications of nanoscale devices will lead to further proprietary approaches to drug discovery as well as medical devices important in real-time evaluation of metabolic conditions in patients, likely connected to wireless monitoring devices.
Refinement and extension of several biophysical techniques will ameliorate our basic knowledge of membrane biochemistry.
Acknowledgements
Grateful acknowledgment is made to the research text and posts contributed for this article by Professors Leonenko, Liu, Maheshwari and Tang.
Financial & competing interests disclosure
The authors have no financial interests in presenting this work. The authors would like to thank NSERC (Canada) and the University of Waterloo for financial support. The authors have no other relevant affiliations or financial involvement with any organization or entity with a financial interest in or financial conflict with the subject matter or materials discussed in the manuscript apart from those disclosed.
No writing assistance was utilized in the production of this manuscript.
Additional information
Funding
Bibliography
- Honek JF , FrancqA, CartyAJ. Bionanotechnology: small can have a big impact in the medical sciences: a WIN-win situation. Part 1.Fut. Med. Chem.2(10), 1515–1522 (2010).
- Huang PJ , LiuJ. Flow cytometry-assisted detection of adenosine in serum with an immobilized aptamer sensor.Anal. Chem.82, 4020–4026 (2010).
- Ryu WH , BaiSJ, ParkJSet al. Direct extraction of photosynthetic electrons from single algal cells by nanoprobing system. Nano Lett. 10, 1137–1143 (2010).
- Lin TW , HsiehPJ, LinCLet al. Label free detection of protein–protein interactions using a calmodulin-modified nanowire transistor. Proc. Natl Acad. Sci. USA 107, 1047–1052 (2010).
- Patolsky F , TimkoBP, YuGet al. Detection, stimulation and inhibition of neuronal signals with high-density nanowire transistor arrays. Science 313, 1100–1104 (2006).
- Maheshwari V , FomenkoDE, SinghG, SarafRF. Ion mediated monolayer deposition of gold nanoparticles on microorganisms: discrimination by age.Langmuir26, 371–377 (2010).
- Morris VJ , KirbyAR, GunningAP. Atomic Force Microscopy for Biologists. Imperial College Press, London, UK (1999).
- Cappella B , DietlerG. Force-distance curves by atomic force microscopy.Surf. Sci. Rep.34(1), 5–104 (1999).
- Zerveck U , LoppacherC, OttoT, GrafstromS, EngLM. Accuracy and resolution limits of Kelvin probe force microscopy.Phys. Rev. B71(12), 1–9 (2005).
- Dobson CM . The structural basis of protein folding and its links with human disease.Phil. Trans. R. Soc. Lond. B356(1406), 133–145 (2001).
- Johansson J . Molecular determinants for amyloid fibril formation: lessons from lung surfactant protein C.Swiss Med. Wkly133, 275–28 (2003).
- Hane F , MooresB, AmreinM, LeonenkoZ. Effect of SP-C on surface potential distribution in pulmonary surfactant: atomic force microscopy and Kelvin-probe force microscopy study.Ultramicroscopy109, 968–973 (2009).
- Finot E , LeonenkoY, MooresB, EngLM, AmreinM, LeonenkoZ. Effect of cholesterol on electrostatics in lipid–protein films of a pulmonary surfactant.Langmuir26(3), 1929–1935 (2010).
- Hane F , DrolleE, LeonenkoZ. Effect of cholesterol and amyloid-β peptide on structure and function of mixed-lipid films and pulmonary surfactant BLES.J. Nanomedicine DOI:10.1016/j.nano.2010.05.001 (2010) (Epub ahead of print).
Website
- JPK Instruments. http//www.jpk.com