Abstract
RNA activation (RNAa) allows specific gene upregulation mediated by a small activating RNA (saRNA). Harnessing this process would help in developing novel therapeutics for undruggable diseases. Since its discovery in mid 2000s, improvements of saRNA design, synthetic chemistry and understanding of the biology have matured the way to apply RNAa. Indeed, MiNA therapeutics Ltd has conducted the first RNAa clinical trial for advanced hepatocellular carcinoma patients with promising outcomes. However, to fully realize the RNAa potential better saRNA delivery strategies are needed to target other diseases. Currently, saRNA can be delivered in vivo by lipid nanoparticles, dendrimers, lipid and polymer hybrids and aptamers. Further developing these delivery technologies and novel application of RNAa will prove to be invaluable for new treatment development.
RNA activation (RNAa) is a process mediated by RNAs to enhance gene expression. This pathway is highly regulated and evolutionarily conserved [Citation1–5]; therefore, there is enormous potential to apply this gene activation mechanism to target undruggable diseases. It was first discovered in mid 2000s, when Li et al. observed that small activating RNAs (saRNA), which were designed to have complementary sequences to the promoter regions of E-cadherin, p21WAF1/CIP1(p21) and VEGF, mediate sequence specific mRNA transcription activation of the respective targeted genes in mammalian cells [Citation6]. Janowski et al. also demonstrated that dsRNA can be designed specifically to target upregulation of the progesterone receptor [Citation7]. Subsequent to these observations of gene activation mediated by exogenous RNAs, it was found that endogenous small RNAs such as miRNA can induce gene upregulation. It was observed that mir-373 targets E-cadherin and CSDC2 promoters to increase gene expression [Citation8]. Another study also demonstrated that another class of endogenous small RNA, piwi-interacting RNA, can increase specific gene expression [Citation9]. Overall, these studies highlight the crucial role of endogenous small RNA in governing cell physiology, growth and development, and that saRNA can be designed to induce specific gene upregulation through a tightly regulated gene activation mechanism.
RNAa mechanism
The saRNA is a class of noncoding RNA composed with a 21-nucleotide dsRNA with 2-nucleotide overhangs at both ends. Indeed, saRNA has an identical structure and chemical components to a small interfering RNA (siRNA) despite their completely opposite biological functions. The key difference between saRNA and siRNA lies at the mechanism of action. Cytosolic saRNA is specifically loaded to an AGO2 protein () [Citation6,Citation10], unlike siRNA which can bind to AGO1-4 for activity. AGO 1, 3 and 4 can bind to RNA to mediate siRNA induced gene silencing although their actual roles are still remained to be fully discovered [Citation11]. In particular, AGO2, the only AGO variant processes catalytic activity, is important for both RNAi and RNAa [Citation12–14]. Knocking down of AGO 1, 3 and 4 do not affect gene activation mediated by saRNA while silencing of AGO2 abolishes gene upregulation [Citation6]. It has also been observed that saRNA binds only to AGO2 but not the other AGO variants [Citation10]. To trigger gene activation, the RNA-AGO2 complex is transported to the nucleus, likely by importin-8, for gene activation [Citation15], while in the case of siRNA-mediated gene silencing, the RNA-induced silencing complex can mediate gene knockdown in cytoplasm and nucleus [Citation16]. It is observed that nuclear AGO2-RNA complexes induce RNAa by targeting directly to gene promoters [Citation6,Citation8] or RNAs, which are either promoter associated transcripts or transcripts outside the promoter region such as long noncoding RNA [Citation6,Citation17–19]. The nuclear saRNA bound AGO2 complex recruits key proteins for transcription initiation such as RNA helicase A (RHA), RNA polymerase-associated protein CTR9 homolog (CTR9) and RNA polymerase II-associated factor 1 homolog (PAF1) at the targeted promoter site [Citation20,Citation21]. The RHA is involved in recruiting the transcription machinery to promoters and modifying the chromatin structure to initiate transcriptional activation [Citation22–24]. The CTR9 and PAF1 are components of the PAF1 complex which mediates transcription initiation and elongation [Citation21,Citation24–26]. The PAF1 complex is crucial in initiating histone modifications associated with active genes via the recruitment of key histone-modifying factors to the RNA polymerase II complex [Citation26–29]. All these studies highlight the association of the saRNA-AGO2 complex with other key proteins for transcription initiation although further studies are needed to elucidate the full details of the entire mechanism of RNAa mediated by saRNA. Since saRNA is very effective in inducing targeted gene upregulation, there is strong potential to apply this technology to target currently undruggable diseases.
(1) An endogenously occurring or exogenously introduced double-stranded saRNA is loaded into an AGO2 protein. (2) One of the saRNA duplex strands, the passenger strand, is cleaved and discarded in the cytoplasm and an active saRNA–AGO2 complex is formed. The complex enters the nucleus either by passive transport during mitosis or is actively transported across the nuclear envelope. (3) The active saRNA–AGO2 complex binds to cognate promoter sequences (complementary DNA or naturally occurring noncoding RNA transcripts) in association with the RNA helicase RHA and the RNA polymerase-associated protein CTR9. This results in the recruitment of histone remodeling enzymes, an open chromatin structure and RNA polymerase II-mediated transcriptional activation. (4) There is an increase in mRNA levels of the saRNA target and ultimately an increase in target protein production.
RHA: RNA helicase A; saRNA: Small activating RNA.
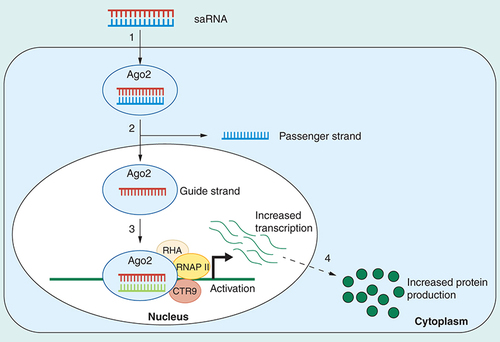
It should be noted that RNAa can also be mediated by other classes of RNAs such as siRNA, miRNA, enhancer RNA (eRNA) and long non-coding RNA with different mechanisms. Paradoxically, siRNA and miRNA can induce gene activation in some cases by acting on natural antisense transcripts (NATs). The NATs express endogenously and play an important role in governing expression. The sequence of NATs is often complementary to sense genes, their promoters or other regulatory regions. The NATs have been shown to regulate targeted gene expressions either through repression or activation, which is referred as discordant or concordant regulation respectively [Citation30]. Indeed, Johnsson et al. demonstrated that silencing of a NAT related to PTEN by siRNA increases PTEN transcription [Citation31]. Other studies also demonstrated miRNA and gapmer can be used to mediate gene activation by inhibiting NATs [Citation32,Citation33]. However, to harness gene activation via derepression, a NAT must be present for the gene target.
The eRNA and lncRNA are two other classes of RNA that can mediate gene activation. The eRNA and lncRNA are rather large RNAs: the size of an eRNA can be between 1 and 5 kb [Citation34–36], while lncRNAs are often longer than 200 nucleotides [Citation37]. Fully discussing the mechanistic actions of eRNA and lncRNA will be out of the scope of this review, but the key theme of these RNAs is that they are transcribed from regions far from the targeted promoters, and eRNA and lncRNA can mediate endogenous gene activation via epigenetic activators, mediators and cohesion protein complexes respectively. The current understandings of the gene regulation mechanisms of lncRNA and eRNA are concisely discussed in these reviews [Citation37–39].
saRNA as therapeutics: promise, challenge & progress
Decreased key protein expression is often the main cause of a number of diseases, the development of novel technologies to restore the expression of these proteins in the cells is of intense interests for better treatments. To overexpress a gene of interest, mRNA, Cripsr activation, antisense oligonucleotides and saRNA can be employed. Although mRNA can be synthesized chemically, it is difficult to produce mRNA longer than 150 nucleotides in length and the mRNA yield decreases exponentially as the length of mRNA increases [Citation40]. Longer mRNA can be produced by using a polymerase such as the T7 RNA polymerase. However, the T7 RNA polymerase can produce various kinds of aberrant byproducts, with a percentage of the byproducts that can be up to 70% [Citation40,Citation41]. The transcripts are also known to stimulate the immune system [Citation40]. Currently, it is still challenging to produce mRNA in a large industrial scale by the enzymatic method [Citation42]. These current hurdles limit mRNA as a therapeutic for overexpressing a gene target for disease treatment.
Crispr technology has been used for gene activation. There have been reports which demonstrated that by delivering a guide RNA and a modified Cas9 enzyme into cells, specific gene expression can be elevated [Citation43,Citation44]. While this technology can have a huge impact in the future, current understanding of Crispr and the potential downstream side effects still need to be improved before applying the technology for clinical use [Citation45]. One of the key problems of the Crispr system is that both the guide RNA and Cas9 need to be delivered to the targeted cells [Citation45], imposing an immense challenge to translate the technology with the current delivery systems.
The ASOs can be utilized for gene activation. Liang et al. have demonstrated that upregulation of gene expression by an ASO is possible [Citation46]. However, to increase the gene expression of a targeted gene, the ASO needs to be designed to inhibit mRNA translation from the upstream open reading frame at the 5′-UTR [Citation46]. This may limit the gene candidates that can be overexpressed by this ASO technology because not all the targeted mRNA would contain the upstream open reading frame. Modarresi et al. also demonstrated that using a gapmer to inhibit a NAT can result in targeted gene activation [Citation32]. This strategy can be attractive for gene activation, but the challenge will be to identify the NAT of the targeted gene for derepression.
The saRNA is an attractive agent to be used as a therapeutic not only due to its highly regulated gene activation mechanism, but also its chemistry and design. Being a small RNA, saRNA can be easily synthesized chemically in high yields. Bioinformatic tools have also been developed to guide the prediction of saRNA sequences to act on targeted promoters for activating transcription, allowing an subsequent identification of saRNA to activate specific gene experimentally [Citation6,Citation10,Citation47]. Several challenges are needed to be overcome to apply saRNA as a medicine such as potential activation of the immune response, off-target effects and poor RNA stability. Current development in chemistry, improved understanding of the saRNA biology and delivery strategies can help in realizing the full potential of saRNA in the clinic. In the following sections, strategies to improve saRNA stability, potency, off-target effect and tissue delivery, as well as ways to decrease unwanted immune response will be discussed.
Stability & potency
Small dsRNA can be unstable in serum with a half-life less than 4 h in 50% serum and 5 min under the 90% serum condition due to nuclease attack [Citation48,Citation49]. Efforts have been employed to increase the small dsRNA nuclease resistance by incorporating 2′-O-methyl and 2′-fluoro modifications in the RNA [Citation50]. Interestingly, such modifications in siRNA can also increase its potency, which could be due to an enhanced interaction between the guide strand and the targeted nucleic acids [Citation50]. However, in the case of saRNA, it was observed that when 2′-fluoro is applied to both sense and antisense strands, the gene activation potency decreases. On the other hand, restricting the modifications only to the guide strand does not affect gene activation efficacy [Citation51,Citation52]. This modification also improves the saRNA stability [Citation51].
The locked nucleic acid modification is known to increase RNA stability. This modification was applied to saRNA. locked nucleic acid within the passenger and/or guide strand(s) in general decreases activity, although the modification at both ends of the passenger strand resumes gene activation efficacy back to the native saRNA level [Citation52]. The potency of saRNA can also be enhanced by an addition of a 5′ inverted abasic modification on the sense strand. This modification improves saRNA activity likely through a more effective antisense strand loading in AGO2 [Citation10]. Overall, these studies indicated that chemical modifications on saRNA can increase the stability and in some cases potency as well. More systematic studies on different modification types at selected positions within the saRNA will therefore further the understanding of the chemical rules of modification for saRNA and will help produce more efficient saRNA for therapeutic use.
Immune response
Being a dsRNA, saRNA can be immunogenic. Efforts have been devoted to investigating chemical modifications to mute the immunogenicity. The 2′-fluoro modification on the saRNA specially on cytidines and uridines of the guide strand can significantly inhibit the immune stimulatory effects of saRNA [Citation51]. It was found that 2′-O-methyl modifications on the passenger strand and overhangs of the guide strand can abolish immune activation [Citation10]. The saRNA with such modifications has an increased safety profile for the clinic and is currently tested in a Phase I clinical trial [Citation10].
Off-target effect
Since saRNA has the same structure as siRNA, it is possible that saRNA can mediate off target effects. Strategies have been employed to allow the selection of saRNA only specific to the targeted sequence. The seed sequence of the saRNA can be used to predict any complementary binding of any transcript by a bioinformatic analysis. Once the possible off-target transcripts are identified, experiments can be conducted to investigate if the saRNA can cause gene silencing or activation of these transcripts [Citation10]. The saRNA with mutations in the seed sequence can also be used as controls for the off-target effect [Citation10]. To further validate the specific phenotype induced by saRNA, overexpression of the specific gene by other vector systems can be employed for a phenotypic comparison [Citation53]. Thus, these strategies would allow the selection of a target specific saRNA.
Delivery of saRNA
Although saRNA can be a tool to target currently undruggable diseases, saRNA needs to be delivered to the targeted cells to achieve its full potential as a therapeutic. It is difficult to deliver naked saRNA in vivo because dsRNA can easily be degraded by nucleases [Citation54]. Being a double stranded nucleic acid, saRNA has the nucleobases pairing inside, leaving the hydrated phosphates on the outside. These negatively charged phosphates do not favor the interaction between saRNA and the negatively charged cell membrane, and saRNA is easily subjected to renal clearance [Citation54]. To improve the pharmacokinetics and allow saRNA to be delivered to the targeted tissues, different strategies have been developed to increase delivery efficacy in vivo. Such strategies involve the use of lipids, dendrimers, lipid and polymer hybrids as well as aptamers for improved in vivo saRNA transfer (). These saRNA delivery strategies will be discussed in this section ().
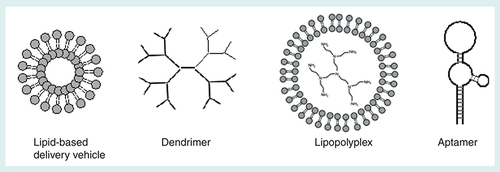
Table 1. In vivo models used to test strategies for mediating small activating RNA.
Lipid-based system
Cationic lipid was first shown in 1987 to be able to deliver plasmid DNA [Citation64]. Lipid-based systems subsequently have become one of the most widely used systems for nucleic acids delivery (A) [Citation65]. Lipid can encapsulate nucleic acids for cellular uptake via clathrin-mediated endocytosis, caveolae-mediated endocytosis, macropinocytosis, and clathrin and caveolae-independent endocytosis, depending on the size of the lipid–nucleic acid complexes as well as the targeted cells. Once being taken up into the cells, the lipid complexes are likely to be trapped in endosomes which would in turn fuse with lysosomes. Such event is characterized by a drop in the pH. The ability of a lipid based system to mediate an endosomal escape depends if the cationic lipids can effectively interact with anionic phospholipids from the endosomes, and the ability of the system to transit from the lamellar to the hexagonal phase to aid the endosomal escape [Citation66–69].
Lipid-based systems are effective in delivering siRNA/oligonucleotide cargos in vivo for transthyretin-mediated amyloidosis and cancer treatments [Citation70–73]. Previously, library screens of different types of lipidoids have yielded structure–function relationship understanding of the optimal lipidoid for siRNA delivery [Citation70,Citation74,Citation75]. These studies demonstrated that lipidoid can effectively deliver siRNA in the liver, indicating the potential of this class of molecules to be used in the clinic. Place et al. have investigated if lipidoid can deliver saRNA targeting the promoter of p21 for gene upregulation for tumor growth suppression [Citation51]. The study was conducted in a prostate cancer xenograft mouse model with an intratumor injection of the lipidoid-based formulation. Their results suggested that the lipidoid–saRNA complex were able to mediate strong tumor regression, with p21 protein expressed strongly in the tumor tissues. Kang et al. also applied lipidoid-saRNA to inhibit bladder cancer growth by increasing p21 levels via intravesical delivery in an orthotopic mouse model. Treatments with the lipidoid–saRNA in the mice led to a 40% tumor regression with increased p21 expression and apoptosis in the tumor tissues [Citation55]. Lipidoid has also been used to deliver saRNA upregulating NKX3-1, which is frequently downregulated in prostate cancer, in a xenograft prostate tumor mouse model via intratumor injections. Such treatment significantly decreased tumor burden and improved survival [Citation62]. These proof-of-concept studies indicated the potential of using the clinical relevant lipidoid system as a vehicle for saRNA therapy [Citation76]. However, so far lipidoid–saRNA complexes are confined to local delivery, more studies are still required to evaluate whether this system can be applied for saRNA delivery via different routes to tissues.
Another liposome platform system, called SMARTICLES, can also deliver nucleic acids [Citation73,Citation77,Citation78]. SMARTICLES is an amphoteric lipid system with a charge reversible character. It contains cationic lipid, which provides cationic charge at a low pH, and anionic lipid, which is pH-sensitive and amphiphilic [Citation77]. The pH sensitive SMARTICLES mediates effective intracellular delivery of nucleic acids, likely through an improved endosomal escape mechanism. The key features of SMARTICLES are that it is very stable in serum and effective in delivering nucleic acid cargos to the liver [Citation56], monocyte/macrophages and myeloid dendritic cells [Citation77].
Reebye et al. have critically evaluated the efficacy of SMARTICLES to deliver saRNA upregulating Cebpa in various liver disease models and demonstrated that SMARTICLES can effectively deliver saRNA in the liver to achieve therapeutic outcomes [Citation56]. Administrations of SMATICLES-saRNA in the diethylnitrosamine-induced cirrhotic HCC rat model intravenously three-times a week resulted in upregulation of Cebpa, improvement of liver function and a significant 80% decrease of tumor size [Citation56]. The SMARTICLES-saRNA was also tested to deliver nucleic acid cargos into a liver failure rat model exposed to the hepatotoxin CCl4. Biweekly injections of the SMARTICLES-saRNA particles increased hepatic expression of Cebpa and significantly reduced fibrosis. Crucially, the administration of the SMARTICLES-saRNA to the CCl4 treated rats resulted in a 78% increased survival benefit [Citation56]. Reebye et al. have also investigated the therapeutic efficacy of SMARTICLES for nonalcoholic fatty liver disease treatments. Mice from the methionine and choline-deficient diet induced nonalcoholic steatohepatitis model were injected biweekly with the SMARTICLES-saRNA upregulating Cebpa. It was observed that the treatment of SMARTICLES with the saRNA mediated improved liver functions, reduced hepatic triacylglycercide and macrophage infiltration within the liver [Citation56]. Taken together, these studies indicated that lipid-based systems can be effective for saRNA delivery. However, current systems only allow liver as well as intratumor targeting, further investigation on switching tropisms to other tissues for saRNA transfer will be crucial for the development of therapeutics for other diseases.
Dendrimer
Polymer-based systems have been widely used for nucleic acid delivery [Citation69]. Specifically to small dsRNA, branched polymers can provide a flexible structure to effectively encapsulate ‘rigid’ small RNA [Citation79,Citation80]. Dendrimers are symmetrical hyperbranched and star-shaped polymer systems (B). The key advantages of dendrimers are that they are versatile, derivatizable and generally monodisperse with a well-defined size. Various studies have shown that dendrimers are effective in delivering nucleic acids in vitro and in vivo [Citation81–86].
Polyamidoamine (PAMAM) is one of the most widely used dendrimers for nucleic acid delivery, especially for small RNAs. It was the first class of dendrimers to be synthesized [Citation87], and the synthetic and derivatization procedures are relatively simple [Citation83,Citation88]. This dendrimer contains primary amine groups on its spherical surface, and such amine groups can be protonated at the physiological pH, allowing the dendrimers to bind and condense negatively charged nucleic acids via electrostatic interactions to form stable and uniform nanoscale complexes [Citation89–91]. The formation of the nanocomplexes protects the RNA from nuclease degradation and promote cellular uptake through endocytosis. Depending on the size of the nanocomplexes as well as the cell types, one or more uptake mechanisms could be triggered [Citation92]. Generally, the uptake mechanism would involve clathrin-mediated, caveolin-mediated endocytosis, macropinocytosis and/or phagocytosis [Citation93–96].
Once within the cells, the nanocomplexes are likely to be trapped in endosomes, which will then mature to late endosomes and fuse with lysosomes. The pH during the maturation of the endosomes drops as a result of the proton pump vacuolar ATPase mediating an influx of hydrogen ions. The secondary and tertiary amines in PAMAM can counteract to the drop of pH by uptaking hydrogen ions. As a result, more protons will influx in the endosomes and this in turns enhanced the accumulation of Cl- within the endosomes. This increases the number of water molecules to move within the endosomes due to the osmotic pressure. In the end, the swollen endosomes will rupture and release the content into the cytosol, and this is known as the ‘proton sponge effect’ [Citation97]. Interestingly, PAMAM with defective branches can also mediate the ‘umbrella effect’ in which the degraded PAMAM unfolds into an extended conformation after protonation of the amine groups in endosomes. This causes the dendrimer to increase in volume and space and contributes to the endosomal escape to release the nucleic acids [Citation98].
Ryebee et al. have successfully used PAMAM to deliver saRNA upregulating Cebpa expression in a diethylnitrosamine-induced rats hepatocellular carcinoma (HCC) model. Intravenous injections of PAMAM-saRNA complexes in the rats resulted in upregulation of Cebpa, albumin and other critical hepatocyte markers such as Hnf1α and Hnf4α in the liver. The PAMAM-saRNA complexes also increased serum albumin and decreased serum bilirubin, aspartate aminotransferase (AST) and alanine aminotransferase (ALT) which suggested an improvement of liver function. The tumor burden was reduced significantly by 80% with a 40% reduction in a marker of pre-neoplastic transformation [Citation57]. Huan et al. also intravenously injected dendrimer-saRNA upregulating Cebpa in a mouse liver orthotopic xenograft tumor model, and they showed that saRNA upregulating Cebpa can inhibit intrahepatic and distant metastasis. Mice received the dendrimer-saRNA targeting Cebpa had increased serum albumin and decreased ALT, AST, indicating an improvement of liver function [Citation58]. All in all, these studies showed that PAMAM can be used to deliver saRNA in clinically relevant models, indicating that there is a strong potential to further develop the system for clinical use.
Lipopolyplex
Lipopolyplex is a system also widely used for the delivery of nucleic acids to cells [Citation69]. This system consists of three components: lipid, polymer and nucleic acid cargo (C). The key role of the lipid is to enhance membrane transfer especially for the endosomal escape while the polymer is crucial for an effective loading of the nucleic acid cargo [Citation69]. Depending on the size and structure of the nucleic acid cargo, different types of polymers should be used for loading the nucleic acid effectively [Citation69]. Apart from cargo loading, some polymers such as polyethylenimine and histidine rich peptides can aid the endosomal escape through the ‘proton sponge’ effect [Citation69,Citation99,Citation100].
Lipopolyplex is able to deliver saRNA in vivo. Wang et al. demonstrated that lipopolyplexes composed of polyethylenimine as the polymer with a lipid shell modulated with hyaluronan allowed targeted delivery of saRNA upregulating the tumor suppressor p21 in the colorectal cancer cells via an rectal administration in an orthotopic murine model. Particularly, the hyaluronan allowed effective binding to CD44 positive cancer cells [Citation59]. This study provides a proof of concept of using lipopolyplex for saRNA delivery. However, the study for saRNA transfer is limited for local delivery, whether the system can be applied for other delivery routes for tissue targeting remained to be seen.
Aptamer
An RNA aptamer is a class of ssRNA which can fold into 3D structures to specifically bind to targeted cell surface motifs (D). It represents as an attractive targeting tools due to their stability, low toxicity, low immunogenicity and improved safety when compared with antibodies [Citation101]. Once an aptamer binds to a cell surface motif, it is taken up typically via clathrin-mediated endocytosis or micropinocytosis. The uptaken aptamer thus is likely to be trapped in endosomes and eventually ended up in lysosomes, although sometimes the cargos would be ended up in endoplasmic reticulum, Golgi apparatus [Citation102].
Yoon et al. have developed an aptamer system for targeted delivery of saRNA to pancreatic cancer cells in vivo. Through a systematic screening and enrichment of specific aptamers by the Systematic Evolution of Ligands by EXponential enrichment (SELEX), Yoon et al. identified aptamers that targeted pancreatic ductal adenocarcinoma. They subsequently linked the aptamers, which contained 2′fluropyrimidine to increase nuclease resistance, to saRNA upregulating of the tumor suppressor CEBPA [Citation60]. Injections of the aptamer–saRNA in mice bearing a PANC1 pancreatic tumor xenograft resulted in 30% more reduction of the tumor size compared with mice treated with Gemcitabine, an antipancreatic tumor drug used as the current standard of care [Citation60]. Crucially, administering aptamer-saRNA to the gemcitabine resistant AsPC-1 cell xenograft mouse model mediated more than 30% of tumor regression when compared with the gemcitabine and vehicle control with no toxicity [Citation60].
RNA aptamer was also used to deliver saRNA to target prostate cancer. A prostate-specific membrane antigen, a cell-surface receptor overexpressed in prostate cancer cells and tumor vascular endothelium [Citation103], was conjugated with saRNA upregulating dihydro-pyrimidinase-like 3 (DPYSL3), which was identified as a metastatic suppressor in prostate cancers. Administrations of this aptamer-saRNA in nude mice bearing orthotopic xenografts of prostate cancer through a 10-day consecutive treatment schedule mediated suppression of distal metastasis through an reduction of tumor cell mobility [Citation61]. These studies have demonstrated that saRNA coupled with aptamers can be effectively used for antitumor therapy, highlighting the potential of applying aptamers for saRNA delivery to target other diseases. Since aptamer-saRNA is a small structure with low immunogenicity and can be modified to be highly stable, it can be developed as a promising candidate for clinical therapy.
saRNA in clinical trial
Several oligonucleotide therapeutics have been developed for clinical use, including Vitravene (fomivirsen), Kynamro® (mipomirsen), Spinraza® (nusinersen) and Onpattro™ (patisiran), which are US FDA-approved ASO and siRNA drugs [Citation104–107]. Fomivirsen disrupts the replication of human cytomegalovirus (CMV) by targeting the mRNA of the major immediate early region proteins of the virus. However, the advent of high-activity anti-retroviral therapy led to a dramatic reduction in the number of CMV cases and therefore marketing of Fomivirsen was discontinued [Citation107]. On the other hand, another ASO, Kynamro, was approved to treat of homozygous familial hypercholesterolemia, a rare genetic disorder in which the body fails to remove the LDL cholesterol from the bloodstream [Citation107]. Spinraz was approved by US FDA in 2016 and allows treatment of spinal muscular atrophy through splicing correction to restore expression of a fully functional protein [Citation108]. Onpattro has been developed for the treatment of polyneuropathy of hereditary transthyretin (TTR)-mediated amyloidosis in adults. This drug mediates downregulation of TTR by an RNA interference-based mechanism [Citation109]. In 2016, the first saRNA-based drug, MTL-CEBPA which mediates upregulation of the transcription factor CEBPA, has been tested in a Phase I clinical trial for patients with advanced liver cancer [Citation10,Citation56,Citation110,Citation111].
Liver cancer is among the leading causes of cancer-related deaths worldwide [Citation112]. Total 80% of primary liver cancers are HCC [Citation113], and HCC is often diagnosed at advanced stages with a poor prognosis of an overall 5-year survival rate of 3–5% in a metastatic disease [Citation111]. The HCC does not respond to most common chemotherapeutics and as a consequence is difficult to treat [Citation114]. Although the primary treatment option for HCC is surgical resection, most patients are ineligible for this procedure due to a poor liver function and/or large unresectable tumors [Citation114]. The current standard-of-care treatment for advanced HCC is by systemic administrations of the multikinase inhibitor sorafenib; however, this only leads to a modest improvement in life expectancy by 3 months [Citation111]. Thus, there is still an unmet medical need for novel treatment to achieve survival rates beyond 1 year [Citation113].
The CEBPA is a basic leucine zipper transcription factor which is crucial for normal hepatocyte functions [Citation115]. The CEBPA regulates the balance between cell growth and differentiation in a range of tissues and modulates expression of various genes involved in energy homeostasis, metabolic processes and hepatocyte maturation [Citation116]. Interestingly, reduced CEBPA mRNA and protein levels are observed in HCC and hepatocytes derived from CEBPA knockout mice exhibit rapid growth, accumulation of chromosomal abnormalities and have the ability to form nodules in nude mice [Citation111,Citation117,Citation118]. A reverse correlation of CEBPA expression levels and tumor size and clinical stage has been described in HCC and a significant CEBPA downregulation often correlates with poor prognosis [Citation118]. Up to this point, no functional CEBPA mutations have been reported in HCC, which implies that alternative mechanisms such as promoter methylation or other epigenetic alterations are likely to contribute to the abrogation of CEBPA functions [Citation115]. Together with the current preclinical studies indicating hepatic upregulation of CEBPA can decrease tumor burden in vivo [Citation56,Citation57], restoring CEBPA expression in HCC patients could be key to improve patients’ treatments and survival rates.
The MTL-CEBPA consists of an saRNA targeting CEBPA upregulation with chemical modifications to increase stability and avoid activation of innate immunity [Citation10]. The payload is formulated into SMARTICLES for improved saRNA delivery [Citation10,Citation56]. Preliminary results of the Phase I clinical trial have shown that MTL-CEBPA was well tolerated in patients with all doses and no maximum tolerated dose was identified. Side effects of the treatment were mild to moderate in severity. There was also no evidence of drug accumulation. Patients with the MTL-CEBPA treatment had a significant increase of CEBPA expression in white blood cells. While many patients achieved stable disease, particularly a patient had over 70% tumor regression. Interestingly, three patients received MTL-CEBPA during the clinical trial as well as an off-study treatment of tyrosine kinase inhibitors demonstrated a striking outcome. Two of the three patients showed complete tumor responses together with a marked decrease in the α-fetoprotein tumor marker, while one patient experienced a partial tumor response. Overall, this promising initial data demonstrated the proof of concept of using saRNA to increase targeted gene expression and consequently improve patients’ outcome in the clinic.
Apart from HCC, decreased levels of CEBPA have been connected to other solid cancers such as lung, skin, cervical and head and neck squamous cell carcinoma and diseases such diabetes mellitus, endometriosis, asthma, chronic obstructive pulmonary disease and respiratory distress syndrome [Citation104–116]. Despite the initial promising results of MTL-CEBPA in liver cancer, at the moment delivery of the drug is mainly restricted to the liver and white blood cells. The development of novel targeted delivery strategies targeting CEBPA saRNA to additional tissues affected by CEBPA downregulation could open novel therapeutic avenues for other diseases.
Conclusion & future perspective
The recent US FDA approval of Onpattro to treat hereditary transthyretin amyloidosis has excited the field of small RNA therapeutics [Citation105,Citation106]. This shows that small dsRNA molecules can indeed be utilized to treat diseases previously undruggable in the clinic. The first RNAa clinical trial run by MiNA therapeutics Ltd has also showed promises for using saRNA for the treatment of HCC patients. Due to the advantages of gene upregulating using saRNA, it is anticipated that more saRNA drugs will be developed to the market and transform patients’ health, treatment and medicine in general. Further understanding of the saRNA chemistry and the gene upregulation mechanism will help in improving the selection of saRNA with minimal off-target effect and immunogenicity, with enhanced potency and stability. However, the key to unlock the potential relies on better strategies to deliver small dsRNA effectively to targeted cells.
Apart from using saRNA as a single agent, it is foreseen that saRNA could be further applied to different treatment paradigms. One possibility is to combine saRNA with other drugs for improved treatments. Indeed, it was observed that saRNA targeting p21 can enhance the sensitivity of A549 non-small-cell lung carcinoma to cisplatin [Citation132]. More studies focus on the benefit of combinational treatments could be proven to be invaluable. Since saRNA is a very potent tool for gene activation, it has been applied in regenerative medicine. Voutila et al. have demonstrated that saRNA can be an attractive method to reprogram human mesenchymal stem cells compared with the standard lentiviral based method since saRNA does not have concerns arisen from the lentiviral method such as insertion mutagenesis, induction of interferon response as well as activation of TGFβ signaling, which in turn could decrease reprogramming efficacy [Citation47]. There is also a report on the applying saRNA to upregulate the islet-β cell master transcription factor to enhance the adult hematopoietic CD34+ stem cell maturation to insulin-secreting cells when responds to different glucose levels [Citation133]. These studies demonstrate the versatility of saRNA and it is expected that RNAa will play a more central role in medicine in the future.
RNA activation
RNA activation (RNAa) can be mediated by small activating RNAs (saRNAs) via a highly regulated, evolutionarily conserved mechanism, harnessing RNAa can prove to be invaluable for the development of novel treatment for undruggable diseases.
To activate gene expression, the guide strand of the saRNA is loaded to AGO2, and the complex needs to be transported to the nucleus.
The nuclear guide strand–AGO2 complex targets directly to gene promoters or associated transcripts, and recruits key components including RNA polymerase II to initiate gene activation.
saRNA design & modification
Key challenges to apply saRNA in vivo include off-target effect, immune activation, saRNA potency, stability and delivery.
Advancement of chemical modifications on saRNA allows improvement of stability, potency and immune response profile, while the off-target effect can be minimized with current bioinformatic tools coupled with experiment testing.
saRNA delivery strategies
The saRNA can be delivered in vivo using lipid nanoparticles, dendrimers, lipopolyplexes and aptamers.
Despite the effectiveness of these systems, in vivo saRNA delivery are confined mainly to liver targeting as well as local tissue injections. Therefore, better systems are needed to allow targeting of other tissues.
RNAa clinical trial
The first RNAa Phase I clinical trial indicates that the lipid nanoparticle encapsulated saRNA drug, MTL-CEBPA, is well tolerated with patients achieving stable disease or tumor regression.
Future perspective for RNAa
Together with the recent approval of siRNA for clinical use and the promising outcome of the RNAa clinical trial, it is expected that RNAa will play a more central role in medicine.
To fully harness this potential, efforts are needed to develop better saRNA delivery systems and strategies.
Acknowledgments
Authors would like to thank Dr CP Tan, Dr A Debacker, Dr D Vasconcelos and Dr L Sinigaglia for useful discussions.
Financial & competing interests disclosure
A Kwok and N Raulf are employees of MiNA therapeutics Ltd. N Habib is a cofounder, Head of Research and shareholder of MiNA therapeutics Ltd. N Habib is a Professor and Head of the Department of HPB Surgery at Imperial College London. The authors have no other relevant affiliations or financial involvement with any organization or entity with a financial interest in or financial conflict with the subject matter or materials discussed in the manuscript apart from those disclosed.
No writing assistance was utilized in the production of this manuscript.
References
- Uesaka M Agata K Oishi T Nakashima K Imamura T . Evolutionary acquisition of promoter-associated non-coding RNA (pancRNA) repertoires diversifies species-dependent gene activation mechanisms in mammals . BMC Genomics18 ( 1 ), 285 ( 2017 ).
- Pennacchio LA Ahituv N Moses AM et al. In vivo enhancer analysis of human conserved non-coding sequences . Nature444 ( 7118 ), 499 – 502 ( 2006 ).
- Visel A Prabhakar S Akiyama JA et al. Ultraconservation identifies a small subset of extremely constrained developmental enhancers . Nat. Genet.40 ( 2 ), 158 – 160 ( 2008 ).
- Huang V Qin Y Wang J et al. RNAa is conserved in mammalian cells . PLoS ONE5 ( 1 ), e8848 ( 2010 ).
- Guo D Barry L Lin SSH Huang V Li LC . RNAa in action: from the exception to the norm . RNA Biol.11 ( 10 ), 1221 – 1225 ( 2014 ).
- Li L-C Okino ST Zhao H et al. Small dsRNAs induce transcriptional activation in human cells . Proc. Natl Acad. Sci. USA103 ( 46 ), 17337 – 17342 ( 2006 ).
- Janowski BA Younger ST Hardy DB Ram R Huffman KE Corey DR . Activating gene expression in mammalian cells with promoter-targeted duplex RNAs . Nat. Chem. Biol.3 ( 3 ), 166 – 173 ( 2007 ).
- Place RF Li L-C Pookot D Noonan EJ Dahiya R . MicroRNA-373 induces expression of genes with complementary promoter sequences . Proc. Natl Acad. Sci. USA105 ( 5 ), 1608 – 1613 ( 2008 ).
- Yin H Lin H . An epigenetic activation role of Piwi and a Piwi-associated piRNA in Drosophila melanogaster . Nature450 ( 7167 ), 304 – 308 ( 2007 ).
- Voutila J Reebye V Roberts TC et al. Development and mechanism of small activating RNA targeting CEBPA, a novel therapeutic in clinical trials for liver cancer . Mol. Ther.25 ( 12 ), 2705 – 2714 ( 2017 ).
- Kalantari R Chiang CM Corey DR . Regulation of mammalian transcription and splicing by nuclear RNAi . Nucleic Acids Res.44 ( 2 ), 524 – 537 ( 2016 ).
- Meister G Landthaler M Patkaniowska A Dorsett Y Teng G Tuschl T . Human Argonaute2 mediates RNA cleavage targeted by miRNAs and siRNAs . Mol. Cell.15 ( 2 ), 185 – 197 ( 2004 ).
- Rand TA Ginalski K Grishin N V Wang X . Biochemical identification of Argonaute 2 as the sole protein required for RNA-induced silencing complex activity . Proc. Natl Acad. Sci. USA101 ( 40 ), 14385 – 14389 ( 2004 ).
- Liu J Carmell MA Rivas F V et al. Argonaute2 is the catalytic engine of mammalian RNAi . Science305 ( 5689 ), 1437 – 1441 ( 2004 ).
- Weinmann L Höck J Ivacevic T et al. Importin 8 is a gene silencing factor that targets argonaute proteins to distinct mRNAs . Cell136 ( 3 ), 496 – 507 ( 2009 ).
- Castel SE Martienssen RA . RNA interference in the nucleus: roles for small RNAs in transcription, epigenetics and beyond . Nat. Rev. Genet.14 ( 2 ), 100 – 112 ( 2013 ).
- Core LJ Waterfall JJ Lis JT . Nascent RNA sequencing reveals widespread pausing and divergent initiation at human promoters . Science322 ( 5909 ), 1845 – 1848 ( 2008 ).
- Seila AC Calabrese JM Levine SS et al. Divergent transcription from active promoters . Science322 ( 5909 ), 1849 – 1851 ( 2008 ).
- Schwartz JC Younger ST Nguyen NB et al. Antisense transcripts are targets for activating small RNAs . Nat. Struct. Mol. Biol.15 ( 8 ), 842 – 848 ( 2008 ).
- Portnoy V Lin SHS Li KH et al. SaRNA-guided Ago2 targets the RITA complex to promoters to stimulate transcription . Cell Res.26 ( 3 ), 320 – 335 ( 2016 ).
- Jaehning JA . The Paf1 complex: Platform or player in RNA polymerase II transcription . Biochim. Biophys. Acta1799 ( 5–6 ), 379 – 388 ( 2010 ).
- Mueller CL Jaehning JA . Ctr9, Rtf1, and Leo1 are components of the Paf1/RNA polymerase II complex . Mol. Cell. Biol.22 ( 7 ), 1971 – 1980 ( 2002 ).
- Tang W You W Shi F et al. RNA helicase A acts as a bridging factor linking nuclear β-actin with RNA polymerase II . Biochem. J.420 ( 3 ), 421 – 428 ( 2009 ).
- Zhang S Grosse F . Molecular characterization of nuclear DNA helicase II (RNA helicase A) . Methods Mol. Biol.587 , 291 – 302 ( 2010 ).
- Rondón AG Gallardo M García-Rubio M Aguilera A . Molecular evidence indicating that the yeast PAF complex is required for transcription elongation . EMBO Rep.5 ( 1 ), 47 – 53 ( 2004 ).
- Marton HA Desiderio S . The Paf1 complex promotes displacement of histones upon rapid induction of transcription by RNA polymerase II . BMC Mol. Biol.9 ( 1 ), 4 ( 2008 ).
- Kim J Guermah M Roeder RG . The human PAF1 complex acts in chromatin transcription elongation both independently and cooperatively with SII/TFIIS . Cell140 ( 4 ), 491 – 503 ( 2010 ).
- Chu Y Yue X Younger ST Janowski BA Corey DR . Involvement of argonaute proteins in gene silencing and activation by RNAs complementary to a noncoding transcript at the progesterone receptor promoter . Nucleic Acids Res.38 ( 21 ), 7736 – 7748 ( 2010 ).
- Chu Y Simic R Warner MH Arndt KM Prelich G . Regulation of histone modification and cryptic transcription by the Bur1 and Paf1 complexes . EMBO J.26 ( 22 ), 4646 – 4656 ( 2007 ).
- Faghihi MA Wahlestedt C . Regulatory roles of natural antisense transcripts . Nat. Rev. Mol. Cell Biol.10 ( 9 ), 637 – 643 ( 2009 ).
- Johnsson P Ackley A Vidarsdottir L et al. A pseudogene long-noncoding-RNA network regulates PTEN transcription and translation in human cells . Nat. Struct. Mol. Biol.20 ( 4 ), 440 – 446 ( 2013 ).
- Modarresi F Faghihi MA Lopez-Toledano MA et al. Inhibition of natural antisense transcripts in vivo results in gene-specific transcriptional upregulation . Nat. Biotechnol.30 ( 5 ), 453 – 459 ( 2012 ).
- Wanowska E Kubiak MR Rosikiewicz W Makałowska I Szcześniak MW . Natural antisense transcripts in diseases: From modes of action to targeted therapies . Wiley Interdiscip. Rev. RNA9 ( 2 ), e1461 ( 2018 ).
- Melo CA Drost J Wijchers PJ et al. ERNAs are required for p53-dependent enhancer activity and gene transcription . Mol. Cell.49 ( 3 ), 524 – 535 ( 2013 ).
- Wang D Garcia-Bassets I Benner C et al. Reprogramming transcription by distinct classes of enhancers functionally defined by eRNA . Nature474 ( 7351 ), 390 – 397 ( 2011 ).
- Hah N Murakami S Nagari A Danko CG Kraus WL . Enhancer transcripts mark active estrogen receptor binding sites . Genome Res.23 ( 8 ), 1210 – 23 ( 2013 ).
- Ulitsky I Bartel DP . X LincRNAs: genomics, evolution, and mechanisms . Cell154 ( 1 ), 26 – 46 ( 2013 ).
- Jiao AL Slack FJ . RNA-mediated gene activation . Epigenetics9 ( 1 ), 27 – 36 ( 2014 ).
- Li W Notani D Rosenfeld MG . Enhancers as non-coding RNA transcription units: recent insights and future perspectives . Nat. Rev. Genet.17 ( 4 ), 207 – 223 ( 2016 ).
- Mu X Greenwald E Ahmad S Hur S . An origin of the immunogenicity of in vitro transcribed RNA . Nucleic Acids Res.46 ( 10 ), 5239 – 5249 ( 2018 ).
- Triana-Alonso FJ Dabrowski M Wadzack J Nierhaus KH . Self-coded 3′-extension of run-off transcripts produces aberrant products during in vitro transcription with T7 RNA polymerase . J. Biol. Chem.270 ( 11 ), 6298 – 6307 ( 1995 ).
- Kwon H Kim M Seo Y et al. Emergence of synthetic mRNA: in vitro synthesis of mRNA and its applications in regenerative medicine . Biomaterial156 , 172 – 193 ( 2018 ).
- Maeder ML Linder SJ Cascio VM Fu Y Ho QH Joung JK . CRISPR RNA-guided activation of endogenous human genes . Nat. Methods10 ( 10 ), 977 – 979 ( 2013 ).
- Perez-Pinera P Kocak DD Vockley CM et al. RNA-guided gene activation by CRISPR-Cas9-based transcription factors . Nat. Methods10 ( 10 ), 973 – 976 ( 2013 ).
- Xiong X Chen M Lim WA Zhao D Qi LS . CRISP R/Cas9 for human genome engineering and disease research . Annu. Rev. Genomics Hum. Genet.17 ( 1 ), 131 – 154 ( 2016 ).
- Liang XH Shen W Sun H Migawa MT Vickers TA Crooke ST . Translation efficiency of mRNAs is increased by antisense oligonucleotides targeting upstream open reading frames . Nat. Biotechnol.34 ( 8 ), 875 – 880 ( 2016 ).
- Voutila J Sætrom P Mintz P et al. Gene expression profile changes after short-activating RNA-mediated induction of endogenous pluripotency factors in human mesenchymal stem cells . Mol. Ther. Nucleic Acids1 ( 8 ), e35 ( 2012 ).
- Jafari M Xu W Pan R Sweeting CM Karunaratne DN Chen P . Serum stability and physicochemical characterization of a novel amphipathic peptide C6M1 for siRNA delivery . PLoS ONE9 ( 5 ), e97797 ( 2014 ).
- Morrissey DV Blanchard K Shaw L et al. Activity of stabilized short interfering RNA in a mouse model of hepatitis B virus replication . Hepatology41 ( 6 ), 1349 – 1356 ( 2005 ).
- Allerson CR Sioufi N Jarres R et al. Fully 2'-modified oligonucleotide duplexes with improved in vitro potency and stability compared to unmodified small interfering RNA . J. Med. Chem.48 ( 4 ), 901 – 904 ( 2005 ).
- Place RF Wang J Noonan EJ et al. Formulation of small activating RNA into lipidoid nanoparticles inhibits xenograft prostate tumor growth by inducing p21 expression . Mol. Ther. Nucleic Acids1 ( 3 ), e15 ( 2012 ).
- Watts JK Yu D Charisse K et al. Effect of chemical modifications on modulation of gene expression by duplex antigene RNAs that are complementary to non-coding transcripts at gene promoters . Nucleic Acids Res.38 ( 15 ), 5242 – 5259 ( 2010 ).
- Wang J Place RF Huang V et al. Prognostic value and function of KLF4 in prostate cancer: RNAa and vector-mediated overexpression identify KLF4 as an inhibitor of tumor cell growth and migration . Cancer Res.70 ( 24 ), 10182 – 10191 ( 2010 ).
- Juliano R Alam MR Dixit V Kang H . Mechanisms and strategies for effective delivery of antisense and siRNA oligonucleotides . Nucleic Acids Res.36 ( 12 ), 4158 – 4171 ( 2008 ).
- Kang MR Yang G Place RF et al. Intravesical delivery of small activating RNA formulated into lipid nanoparticles inhibits orthotopic bladder tumor growth . Cancer Res.72 ( 19 ), 5069 – 5079 ( 2012 ).
- Reebye V Huang KW Lin V et al. Gene activation of CEBPA using saRNA: preclinical studies of the first in human saRNA drug candidate for liver cancer . Oncogene37 ( 24 ), 3216 – 3228 ( 2018 ).
- Reebye V Sætrom P Mintz PJ et al. Novel RNA oligonucleotide improves liver function and inhibits liver carcinogenesis in vivo . Hepatology59 ( 1 ), 216 – 227 ( 2014 ).
- Huan H Wen X Chen X et al. C/EBPα short-activating RNA suppresses metastasis of hepatocellular carcinoma through inhibiting EGFR/β-catenin signaling mediated EMT . PLoS ONE11 ( 4 ), e0153117 ( 2016 ).
- Wang LL Feng CL Zheng WS et al. Tumor-selective lipopolyplex encapsulated small active RNA hampers colorectal cancer growth in vitro and in orthotopic murine . Biomaterials141 , 13 – 28 ( 2017 ).
- Yoon S Huang KW Reebye V et al. Targeted delivery of C/EBPα -saRNA by pancreatic ductal adenocarcinoma-specific rna aptamers inhibits tumor growth in vivo . Mol. Ther.24 ( 6 ), 1106 – 1116 ( 2016 ).
- Li C Jiang W Hu Q et al. Enhancing DPYSL3 gene expression via a promoter-targeted small activating RNA approach suppresses cancer cell motility and metastasis . Oncotarget7 ( 16 ), 22893 – 910 ( 2016 ).
- Ren S Kang MR Wang J et al. Targeted induction of endogenous NKX3-1 by small activating RNA inhibits prostate tumor growth . Prostate73 ( 14 ), 1591 – 601 ( 2013 ).
- Wang T Li M Yuan H et al. saRNA guided iNOS up-regulation improves erectile function of diabetic rats . J. Urol.190 ( 2 ), 790 – 8 ( 2013 ).
- Felgner PL Gadek TR Holm M et al. Lipofection: a highly efficient, lipid-mediated DNA-transfection procedure . Proc. Natl. Acad. Sci. USA84 ( 21 ), 7413 – 7417 ( 1987 ).
- Li W Szoka FC . Lipid-based nanoparticles for nucleic acid delivery . Pharm. Res.24 ( 3 ), 438 – 449 ( 2007 ).
- Xu Y Szoka FC . Mechanism of DNA release from cationic liposome/DNA complexes used in cell transfection . Biochemistry35 ( 18 ), 5616 – 5623 ( 1996 ).
- Hafez IM Maurer N Cullis PR . On the mechanism whereby cationic lipids promote intracellular delivery of polynucleic acids . Gene Ther.8 ( 15 ), 1188 – 1196 ( 2001 ).
- Zelphati O Szoka FC . Mechanism of oligonucleotide release from cationic liposomes . Proc. Natl. Acad. Sci. USA93 ( 21 ), 11493 – 11498 ( 1996 ).
- Kwok A . The challenges and current advances in delivering RNAi as therapeutics . In : DNA and RNA Nanobiotechnologies in Medicine: Diagnosis and Treatment of Diseases.ErdmannVABarciszewskiJ ( Eds ). Springer , Berlin, Heidelberg, Germany , 189 – 224 ( 2013 ).
- Akinc A Goldberg M Qin J et al. Development of lipidoid–sirna formulations for systemic delivery to the liver . Mol. Ther.17 ( 5 ), 872 – 879 ( 2009 ).
- Aleku M Schulz P Keil O et al. Atu027, a liposomal small interfering RNA formulation targeting protein kinase N3, inhibits cancer progression . Cancer Res.68 ( 23 ), 9788 – 9798 ( 2008 ).
- Nourbakhsh M Jaafari MR Lage H et al. Nanolipoparticles-mediated MDR1 siRNA delivery reduces doxorubicin resistance in breast cancer cells and silences MDR1 expression in xenograft model of human breast cancer . Iran. J. Basic Med. Sci.18 ( 4 ), 385 – 392 ( 2015 ).
- Rodrigueza W V Woolliscroft MJ Ebrahim AS et al. Development and antitumor activity of a BCL-2 targeted single-stranded DNA oligonucleotide . Cancer Chemother. Pharmacol.74 ( 1 ), 151 – 166 ( 2014 ).
- Whitehead KA Dorkin JR Vegas AJ et al. Degradable lipid nanoparticles with predictable in vivo siRNA delivery activity . Nat. Commun.5 ( 1 ), 4277 ( 2014 ).
- Akinc A Zumbuehl A Goldberg M et al. A combinatorial library of lipid-like materials for delivery of RNAi therapeutics . Nat. Biotechnol.26 ( 5 ), 561 – 569 ( 2008 ).
- Ozcan G Ozpolat B Coleman RL Sood AK Lopez-Berestein G . Preclinical and clinical development of siRNA-based therapeutics . Adv. Drug Deliv. Rev.87 , 108 – 119 ( 2015 ).
- Andreakos E Rauchhaus U Stavropoulos A et al. Amphoteric liposomes enable systemic antigen-presenting cell-directed delivery of CD40 antisense and are therapeutically effective in experimental arthritis . Arthritis Rheum.60 ( 4 ), 994 – 1005 ( 2009 ).
- Arranz A Reinsch C Papadakis KA et al. Treatment of experimental murine colitis with CD40 antisense oligonucleotides delivered in amphoteric liposomes . J. Control. Rel.165 ( 3 ), 163 – 172 ( 2013 ).
- Kwok A Hart SL . Comparative structural and functional studies of nanoparticle formulations for DNA and siRNA delivery . Nanomedicine7 ( 2 ), 210 – 219 ( 2011 ).
- Kwok A McCarthy D Hart SL Tagalakis AD . Systematic comparisons of formulations of linear oligolysine peptides with siRNA and plasmid DNA . Chem. Biol. Drug Des.87 ( 5 ), 747 – 763 ( 2016 ).
- Malkoch M Malmström E Nyström AM . Dendrimers: properties and applications . In : Polymer Science: A Comprehensive Reference, 10 Volume Set.MoellerMMatyjaszewskiK ( Eds ). Amsterdam, The Netherlands , 113 – 176 ( 2012 ).
- Biswas S Torchilin VP . Dendrimers for siRNA Delivery . Pharmaceuticals (Basel)6 ( 2 ), 161 – 83 ( 2013 ).
- Mintzer MA Grinstaff MW . Biomedical applications of dendrimers: a tutorial . Chem. Soc. Rev.40 ( 1 ), 173 – 190 ( 2011 ).
- Kwok A Eggimann GA Reymond JL Darbre T Hollfelder F . Peptide dendrimer/lipid hybrid systems are efficient DNA transfection reagents: Structure-activity relationships highlight the role of charge distribution across dendrimer generations . ACS Nano7 ( 5 ), 4668 – 4682 ( 2013 ).
- Kwok A Eggimann GA Heitz M Reymond JL Hollfelder F Darbre T . Efficient transfection of siRNA by peptide dendrimer–lipid conjugates . ChemBioChem17 ( 23 ), 2223 – 2229 ( 2016 ).
- Heitz M Kwok A Eggimann G Hollfelder F Darbre T . Reymond J-L. peptide dendrimer–lipid conjugates as DNA and siRNA transfection reagents: role of charge distribution across generations . Chim. Int. J. Chem.71 ( 4 ), 220 – 225 ( 2017 ).
- Tomalia DA Baker H Dewald J et al. A new class of polymers: starburst-dendritic macromolecules . Polym. J.17 ( 1 ), 117 – 132 ( 1985 ).
- Bauer BJ Amis EJ . Characterization of dendritically branched polymers by small angle neutron scattering (SANS), small angle X-ray scattering (SAXS) and transmission electron microscopy (TEM) . In : Dendrimers and Other Dendritic PolymersFréchetJMJTomaliaDA ( Eds ). John Wiley & Sons, Ltd , Chichester, UK , 255 – 284 ( 2001 ).
- D'Emanuele A Attwood D . Dendrimer-drug interactions . Adv. Drug Deliv. Rev.57 ( 15 ), 2147 – 2162 ( 2005 ).
- Shcharbin D Pedziwiatr E Bryszewska M . How to study dendriplexes I: characterization . J. Control. Rel.135 ( 3 ), 186 – 197 ( 2009 ).
- Shcharbin D Pedziwiatr E Blasiak J Bryszewska M . How to study dendriplexes II: transfection and cytotoxicity . J. Control. Rel.141 ( 2 ), 110 – 127 ( 2010 ).
- Rejman J Bragonzi A Conese M . Role of clathrin-and caveolae-mediated endocytosis in gene transfer mediated by lipo-and polyplexes . Mol. Ther.12 ( 3 ), 468 – 474 ( 2005 ).
- Shen W Van Dongen MA Han Y et al. The role of caveolin-1 and syndecan-4 in the internalization of PEGylated PAMAM dendrimer polyplexes into myoblast and hepatic cells . Eur. J. Pharm. Biopharm.88 ( 3 ), 658 – 663 ( 2014 ).
- Sahay G Alakhova DY Kabanov A V . Endocytosis of nanomedicines . J. Control. Rel.145 ( 3 ), 182 – 195 ( 2010 ).
- Sakurai Y Hatakeyama H Sato Y et al. Endosomal escape and the knockdown efficiency of liposomal-siRNA by the fusogenic peptide shGALA . Biomaterials32 ( 24 ), 5733 – 5742 ( 2011 ).
- Hufnagel H Hakim P Lima A Hollfelder F . Fluid phase endocytosis contributes to transfection of DNA by PEI-25 . Mol. Ther.17 ( 8 ), 1411 – 1417 ( 2009 ).
- Haensler J Szoka FC . Polyamidoamine cascade polymers mediate efficient transfection of cells in culture . Bioconjug. Chem.4 ( 5 ), 372 – 379 ( 1993 ).
- Tang MX Redemann CT Szoka FC . In vitro gene delivery by degraded polyamidoamine dendrimers . Bioconjug. Chem.7 ( 6 ), 703 – 714 ( 1996 ).
- Sonawane ND Szoka FC Verkman AS . Chloride accumulation and swelling in endosomes enhances DNA transfer by polyamine-DNA polyplexes . J. Biol. Chem.278 ( 45 ), 44826 – 44831 ( 2003 ).
- Midoux P Pichon C Yaouanc JJ Jaffrès PA . Chemical vectors for gene delivery: a current review on polymers, peptides and lipids containing histidine or imidazole as nucleic acids carriers . Br. J. Pharmacol.157 ( 2 ), 166 – 178 ( 2009 ).
- Que-Gewirth NS Sullenger BA . Gene therapy progress and prospects: RNA aptamers . Gene Ther.14 ( 4 ), 283 – 291 ( 2007 ).
- Yoon S Rossi JJ . Aptamers: uptake mechanisms and intracellular applications . Adv. Drug Deliv. Rev.134 , 22 – 35 ( 2018 ).
- McNamara JO Andrechek ER Wang Y et al. Cell type-specific delivery of siRNAs with aptamer-siRNA chimeras . Nat. Biotechnol.24 ( 8 ), 1005 – 1015 ( 2006 ).
- Stein CA Castanotto D . FDA-approved oligonucleotide therapies in 2017 . Mol. Ther.25 ( 5 ), 1069 – 1075 ( 2017 ).
- Ledford H . Gene-silencing technology gets first drug approval after 20-year wait . Nature560 ( 7718 ), 291 – 292 ( 2018 ).
- Wood H . FDA approves patisiran to treat hereditary transthyretin amyloidosis . Nat. Rev. Neurol.14 ( 10 ), 570 ( 2018 ).
- Jiang K . Biotech comes to its “antisenses” after hard-won drug approval . Nat. Med.19 ( 3 ), 252 – 252 ( 2013 ).
- Ottesen EW . ISS-N1 makes the first FDA-approved drug for spinal muscular atrophy . Transl. Neurosci.8 ( 1 ), 1 – 6 ( 2017 ).
- Adams D Gonzalez-Duarte A O'Riordan WD et al. Patisiran, an RNAi therapeutic, for hereditary transthyretin amyloidosis . N. Engl. J. Med.379 ( 1 ), 11 – 21 ( 2018 ).
- ClinicalTrials.gov . First-in-Human safety and tolerability study of MTL-CEBPA in patients with advanced liver cancer . https://clinicaltrials.gov/ct2/show/NCT02716012 . 2016 – 2017 ( 2016 ).
- Setten RL Lightfoot HL Habib NA Rossi JJ . Development of MTL-CEBPA: small activating RNA Drug for hepatocellular carcinoma . Curr. Pharm. Biotechnol.19 ( 8 ), 611 – 621 ( 2018 ).
- Medavaram S Zhang Y . Emerging therapies in advanced hepatocellular carcinoma . Exp. Hematol. Oncol.7 ( 1 ), 17 ( 2018 ).
- Llovet JM Montal R Sia D Finn RS . Molecular therapies and precision medicine for hepatocellular carcinoma . Nat. Rev. Clin. Oncol.15 ( 10 ), 599 – 616 ( 2018 ).
- Lohitesh K Chowdhury R Mukherjee S . Resistance a major hindrance to chemotherapy in hepatocellular carcinoma: an insight . Cancer Cell Int.18 , 44 ( 2018 ).
- Lourenço AR Coffer PJ . A tumor suppressor role for C/EBPα in solid tumors: more than fat and blood . Oncogene36 ( 37 ), 5221 – 5230 ( 2017 ).
- Yang J Croniger CM Lekstrom-Himes J et al. Metabolic response of mice to a postnatal ablation of CCAAT/enhancer-binding protein α . J. Biol. Chem.280 ( 46 ), 38689 – 38699 ( 2005 ).
- Tomizawa M Wang Y-Q Ebara M et al. Decreased expression of the CCAAT/enhancer binding protein alpha gene involved in hepatocyte proliferation in human hepatocellular carcinomas . Int. J. Mol. Med.9 ( 6 ), 597 – 600 ( 2002 ).
- Tomizawa M Watanabe K Saisho H Nakagawara A Tagawa M . Down-regulated expression of the CCAAT/enhancer binding protein alpha and beta genes in human hepatocellular carcinoma: a possible prognostic marker . Anticancer Res.23 ( 1a ), 351 – 354 ( 2003 ).
- Tada Y Brena RM Hackanson B Morrison C Otterson GA Plass C . Epigenetic modulation of tumor suppressor CCAAT/enhancer binding protein alpha activity in lung cancer . J. Natl Cancer Inst.98 ( 6 ), 396 – 406 ( 2006 ).
- Halmos B Huettner CS Kocher O Ferenczi K Karp DD Tenen DG . Down-regulation and antiproliferative role of C/EBPalpha in lung cancer . Cancer Res.62 ( 2 ), 528 – 534 ( 2002 ).
- Didon L Roos AB Elmberger GP Gonzalez FJ Nord M . Lung-specific inactivation of CCAAT/enhancer binding protein α causes a pathological pattern characteristic of COPD . Eur. Respir. J.35 ( 1 ), 186 – 197 ( 2010 ).
- Sato A Xu Y Whitsett JA Ikegami M . CCAAT/enhancer binding protein-α regulates the protease/antiprotease balance required for bronchiolar epithelium regeneration . Am. J. Respir. Cell Mol. Biol.47 ( 4 ), 454 – 463 ( 2012 ).
- Sugahara K Iyama K-I Kimura T et al. Mice lacking CCAAT/enhancer-binding protein-a show hyperproliferation of alveolar type II cells and increased surfactant protein mRNAs . Cell Tissue Res.306 ( 1 ), 57 – 63 ( 2001 ).
- Anand S Ebner J Warren CB et al. C/EBP transcription factors in human squamous cell carcinoma: selective changes in expression of isoforms correlate with the neoplastic state . PLoS ONE9 ( 11 ), e112073 ( 2014 ).
- Pan Z Zheng W Zhang J et al. Downregulation of the expression of CCAAT/enhancer binding protein α gene in cervical squamous cell carcinoma . BMC Cancer14 ( 1 ), 417 ( 2014 ).
- Bennett KL Hackanson B Smith LT et al. Tumor suppressor activity of CCAAT/enhancer binding protein is epigenetically down-regulated in head and neck squamous cell carcinoma . Cancer Res.67 ( 10 ), 4657 – 4664 ( 2007 ).
- Kazkayasi I Burul-Bozkurt N Önder S Kelicen-Ugur P Pekiner C . Effects of experimental diabetes on C/EBP proteins in rat hippocampus, sciatic nerve and ganglia . Cell. Mol. Neurobiol.33 ( 4 ), 559 – 567 ( 2013 ).
- Kai K Nasu K Kawano Y et al. Death receptor 6 is epigenetically silenced by histone deacetylation in endometriosis and promotes the pathogenesis of endometriosis . Am. J. Reprod. Immunol.70 ( 6 ), 485 – 496 ( 2013 ).
- Borger P Black JL Roth M . Asthma and the CCAAT-enhancer binding proteins: a holistic view on airway inflammation and remodeling . J. Allergy Clin. Immunol.110 ( 6 ), 841 – 846 ( 2002 ).
- Borger P Tamm M Black JL Roth M . Asthma: is it due to an abnormal airway smooth muscle cell . Am. J. Respir. Crit. Care Med.174 ( 4 ), 367 – 372 ( 2006 ).
- Roth M Johnson PRA Borger P et al. Dysfunctional interaction of C/EBPα and the glucocorticoid receptor in asthmatic bronchial smooth-muscle cells . N. Engl. J. Med.351 ( 6 ), 560 – 574 ( 2004 ).
- Wei J Zhao J Long M et al. P21WAF1/CIP1 gene transcriptional activation exerts cell growth inhibition and enhances chemosensitivity to cisplatin in lung carcinoma cell . BMC Cancer10 , 632 ( 2010 ).
- Reebye V Sætrom P Mintz PJ et al. A short-activating RNA oligonucleotide targeting the islet β-cell transcriptional factor MafA in CD34+ cells . Mol. Ther. Nucleic Acids2 ( 6 ), e97 ( 2013 ).