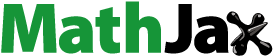
Abstract
Systemic acquired acclimation (SAA) is an important light acclimatory mechanism that depends on the global adjustments of non-photochemical quenching and chloroplast retrograde signaling. As the exact regulation of these processes is not known, we measured time-resolved fluorescence of chlorophyll a in Arabidopsis thaliana leaves exposed to excess light, in leaves undergoing SAA, and in leaves after excess light episode. We compare the behavior induced in wild-type plants with null mutant of non-photochemical quenching (npq4–1). The wild type rosettes exhibit a small reduction of fluorescence decay times in leaves directly exposed to excess light and in leaves undergoing SAA in ambient low light. However in npq4–1 exposition to excess light results in much faster fluorescence decay, which is insensitive to excitation power. At the same time npq4–1 leaves undergoing SAA displayed intermediate fluorescence decay. The npq4–1 plants also lost the ability to optimize florescence decay, and thus chlorophyll a dynamics up to 2 h after excess light episode. The fluorescence decay dynamics in both WT and npq4–1 can be described by a set of 3 maximum decay times. Based on the results, we concluded that functional PsbS is required for optimization of absorbed photon fate and optimal light acclimatory responses such as SAA or after excess light stress.
Abbreviations
DCMU | = | 3,4-dichlorophenyl)-1,1-dimethyl urea |
LHC | = | chlorophyll a/b/xanthophyll-binding proteins |
F0 | = | chlorophyll fluorescence zero |
Fm | = | chlorophyll fluorescence maximum |
Fv | = | chlorophyll fluorescence variable |
FD | = | chlorophyll fluorescence decay |
EEE | = | Excess Excitation Energy |
EL | = | Excess Light |
qE | = | EEE thermal dissipation |
LED | = | Light Emitting Diode |
Fv/Fm | = | maximum quantum efficiency of PSII |
NPQ | = | Non-Photochemical Quenching |
qI | = | photoinhibition |
PSII | = | Photosystem II |
ROS | = | Reactive Oxygen Species |
qT | = | state transition |
SAA | = | Systemic Acquired Acclimation |
WT | = | Wild Type |
qZ | = | zeaxanthin formation |
Introduction
Plants possess natural capacity to absorb light energy in excess of what is sufficient for photochemistry, thus, in full sunlight, only a portion of light energy absorbed by chlorophylls is used for CO2 fixation.Citation1,2 The excess excitation energy (EEE) defines all the absorbed energy that exceeds the amount required for photochemistry, and as such must be dissipated through both fluorescence and heat. Failure to dissipate and quench EEE is highly damaging to plants, leading often to chlorosis, bleaching or bronzing of leaves due to imbalanced reactive oxygen species (ROS) metabolism and EEE dissipation as heat.Citation1-8 Thus, plants evolved systemic acquired acclimation (SAA) mechanisms.Citation7
Non-photochemical quenching (NPQ) is a protective mechanism which, when in balance with photochemical processes, ensures a maximal increase in absorbed energy with minimal damage due to e.g., ROS and heat. It consists of state transition (qT), photoinhibition (qI), EEE thermal dissipation (qE), and zeaxanthin formation (qZ).Citation6,Citation8-11 The most rapid is the third one (qE), which has not yet been fully studied and understood.Citation12 For a proper function of qE three main components are needed: transthylakoidal pH gradient, xanthophylls with a removed epoxy groups from the 3-hydroxo β-rings, and PsbS. PsbS is a nuclear-encoded 22 kDa protein, homologous to chlorophyll a/b/xanthophyll-binding proteins (LHC) superfamily and acts in chloroplast as lumen pH sensor.Citation13,14 Nevertheless, there is a major structural difference between PsbS and other typical members of this family: the PsbS has four transmembrane helices instead of three. The PsbS protein is most likely situated between the core of the PSII and intrinsic antenna proteins.Citation13 PsbS has two glutamate residues (Glu-122 and Glu-226) responsible for pH sensing and both of them are needed for regulation of NPQ.Citation15 Increase of transthylakoidal pH gradient triggers the protonation of 122 and 226 glutamate residuals in PsbS,Citation16 however it is not sufficient to fully activate PsbS and regulate the conformational and allosteric changes of LHC-coreCitation17 as well as changes in chlorophylls orientation.Citation18-20 Recently, however a NPQ mechanism independent of transthylakoidal pH gradient changes was described.Citation11 npq4–1 is a single, semi-dominant nuclear mutation in PsbS and lacks qE capacity.Citation21 Growth as well as seed production of npq4–1 comparing to wild type plants is unaffected in light limiting condition,Citation21 but impaired in the outdoor EEE-generating conditions.Citation22
SAA responses require proper dissipation of the EEE and a very efficient system that is able to globally optimize light absorption, energy quenching, and photochemistry in plants partially exposed to highly variable natural light conditions (full sun, clouds shadows or moving sun spots). SAA is associated with global regulation of NPQ and other chlorophyll a fluorescence parameters, redox changes in the photosynthetic electron transport carriers and subsequent induction of light acclimatory and immune defenses molecular and physiological responses.Citation7,Citation23-28 Recently, it was revealed that EEE-induced permanent photodamage is due to induction of the programmed cell death that is depending on changes in NPQ- and the plastoqinone pool redox status-dependent chloroplast retrograde signaling.Citation7,Citation23-28 In recent work we have demonstrated that photo-electrochemical systemic signaling triggered by local NPQ changes induced systemic NPQ changes.Citation28 These findings led us to consideration of possible global (systemic) regulatory mechanism of absorbed photon fate.
So far, all measurements of fluorescence decay and absorbed photon fate in WT and NPQ Arabidopsis mutants were performed in low light acclimated plants transiently exposed to high light conditions.Citation10,11,21,29 These ambient laboratory conditions do not reflect acclimation of photosystems to the naturally variable light conditions.Citation22,30,31 Recently we demonstrated that NPQ- and plastoquione pool redox status-dependent light acclimation and cell death signaling is differently regulated in the laboratory and in the field.Citation30 We expect, based on previous research,Citation22,30,31 that plants cultivated under naturally variable light conditions (greenhouse) develop different regulatory strategies as compared with plants grown in controlled low light environment that were studied in recent work focused on time-resolved spectroscopy of Arabidopsis.Citation10,11,29 Our results indicate that the fate of absorbed photons is regulated differently in directly EL exposed leaves of Arabidopsis plants cultivated in greenhouse in comparison to the previously reported results for WT and npq4–1. We also demonstrate that the fate of absorbed photons is regulated in leaves undergoing SAA or several hours after EL episode, and that PsbS protein plays a key role in these processes.
Materials and Methods
Plant material and growth conditions
All Arabidopsis thaliana (L.) Heynh. plants used in the experiments were of the ecotype Columbia (Col-0). The npq4–1 mutant containing a deletion of the entire NPQ4 gene (AT1G44575), derived from mutagenesis with fast-neutron bombardment,Citation21 was kindly provided by Professor K Niyogi. A. thaliana plants were growing in a greenhouse under natural long day and variable light conditions (summer 2011 and 2012) at an experimental field in Wolica.Citation30,31
Preparation procedures
Forty μM DCMU pretreatment was used to block photosynthetic electron transport on the secondary electron acceptor QB; infiltration was performed as described before.Citation7,28 SAA was induced as previously describedCitation7,28 with the following modification. Arabidopsis plants were partly (half of the rosette) exposed to 1 h of red LED light (with peak at 620 nm, 25 nm line width) of 3000 μmol photons m−2 s−1, then for chlorophyll fluorescence transients measurements were dark acclimated for minimum 40 min. This half of the plant we name “local.” The systemic part of a plant, i.e., the one not exposed to the red LED light, was always exposed to the ambient white low light (LL, 100–150 μmol photons m−2 s−1) (Photon System Instrument, Brno, Czech Republic).
Measurement of chlorophyll a fluorescence and fluorescence dynamics
Chlorophyll a fluorescence was measured as described before.Citation9,Citation30-32 The OJIP-test was performed as describedCitation33,34 using FluorCam and the associated software (Photon System Instruments, Brno, Czech Republic). Plants were dark-adapted for 40 min prior to measurement.
Fluorescence dynamics was studied with home-built fluorescence backscattering microscope and using time-correlated single photon counting technique (TCSPC). Optics was based on microscope objective LMPlan 50x (Olympus) with numerical aperture 0.5 and 6 mm working distance.Citation35 Sample was placed on XYZ precision translation stage (Thorlabs, Stockholm, Sweden). For excitation we used two picosecond lasers (Becker & Hickl, GmbH, Germany) BLD-488-SCM (485 nm) and BLD-640-SCM (640 nm) operating at a repetition rate of 20 MHz with the FWHM of laser impulse around 30 ps. Initial excitation power of the laser beams exciting the sample were around 12 μW for 485 nm and 8 μW for 640 nm. The setup consists of a SPC-150 TCSPC card (Becker & Hickl, GmbH, Germany) and a fast avalanche photodiode idQuantique id100–50 characterized with dark count of 50 cps with time resolution of about 30 ps. The emission was extracted using appropriate bandpass filters: HQ 600+ and HQ 665+ (Chroma Technology, USA) for 485 nm and 640 nm excitation, respectively. In addition, a narrow band pass filter HQ670/10 (Chroma Technology, USA) was used to spectrally select PSII chlorophyll a fluorescence. Five different points were chosen on every leaf and every point was measured using five different decreasing excitation powers controlled with a set of neutral density filters. Fluorescence dynamics were measured after 30 min of dark acclimation, after 60 min of illumination with actinic light following the dark acclimation, and then laser illumination. An actinic light intensity of 1500 up to 3000 μmol photons m−2 s−1 was used to illuminate the leaves.
Data analysis and statistics
Fluorescence lifetimes (τ) were determined by fitting three-exponential function to normalized fluorescence decays measured at five locations across the leaf of particular genotype and preparation (Equationequation 1(1)
(1) ):
(1)
(1)
For fitting we used OriginPro 7.5 software, and R2 was over 98% for all the fits. Using EquationEq. 1(1)
(1) we also determined relative intensities corresponding to each of the decay time (200 independent measurements that give 600 values of decay times).
Results
Chlorophyll fluorescence transients
Before we measured fluorescence transients we measured several standard OJIP parameters of chlorophyll a fluorescence (). Comparison of WT and npq4–1 plants with respect to the different fluorescence parameters revealed considerable differences in their photosynthetic performance. It was demonstrated in many studies that stress-induced photoinhibition resulted in Fv/Fm decrease.Citation36-38 Indeed, Arabidopsis WT and npq4–1 plants after EL episode displayed significant reduction of maximum quantum efficiency of PSII (Fv/Fm) in comparison to controls (), which suggests that they exhibited more effective quinone A (QA) reduction. This reduction was significantly higher in npq4–1 than in WT plants. Moreover, after 2 h EL the WT plants recovered almost completely from the stress conditions, while npq4–1 still exhibited values far from equilibrium. We also observed behavior similar to Fv/Fm in both WT and npq4–1 for Fv/F0 and PIABS ( and ). Fv/F0 parameter is proportional to the activity of the water-splitting complex on the PSII donor side and damage of D1 protein, and PIABS defines the efficiency of energy transfer for photochemistry. As in the case of Fv/Fm, PIABS in npq4–1 was not able to recover as WT did (). ΦDo defines total dissipation of untrapped EEE regardless of an active PSII reaction center number and indicates for impaired recovery of total dissipation efficiency in npq-4–1. Taken together these parameters confirm severe photodamage and impaired recovery of optimal PSII functions in npq4–1 under variable light conditions and lead us to the question of PsbS role in proper regulation of fluorescence dynamics during various light acclimatory responses, such as SAA and after EL.
Figure 1. The effect of red excess light (EL) illumination on fluorescence transient (OJIP) parameters in WT and npq4–1 mutant: (A) Fv/Fm, (B) Fv/Fo, (C) PIABS, and (D) ΦDo. On the x-axis, “ctrl” is control (plants before treatment); “0” is plants just after EL treatment; “15,” “30,” “60,” and “120” are plants after 15, 30, 60, and 120 min EL treatment, respectively (n = 3–4).
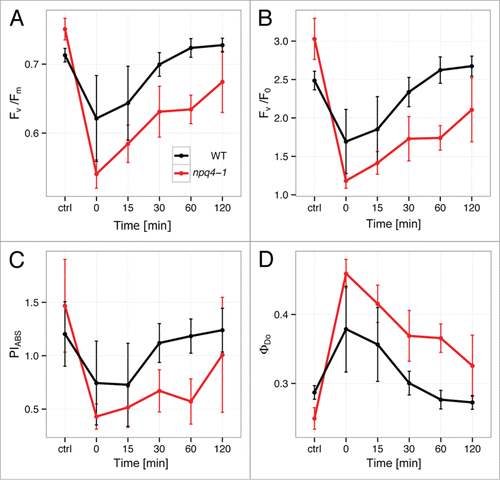
Dynamics of chlorophyll fluorescence
The first experiment concerns fluorescence dynamics of PS II chlorophylls in plants partially exposed to EL achieved using a LED red light illumination. Directly EL exposed part of a plant is named “local,” the second part of the plant undergoing SAA was exposed to the ambient low-light conditions, this part is named “systemic.”
In we present typical fluorescence transients measured for the WT and npq4–1 genotypes for the leaves excited with the lowest excitation power of 120 nW and wavelength of 485 nm. The excitation at 485 nm corresponds to maximum of the absorption of Arabidopsis leaves (Fig. S1). The excitation power is extremely low and at such intensities laser illumination, albeit achieved by using a microscope objective, we observed no changes in fluorescence dynamics over time for a given location. This observation validates the experimental approach described in this work. The data obtained for control leaves is compared with leaves treated with DCMU, exposed to EL episode, and undergoing SAA in both WT and npq4–1. Even qualitatively, the fluorescence transients exhibit strong changes depending upon treatment, genotype, and/or preparation of the sample. DCMU treatment of the WT Arabidopsis leaves results in longer FD similar to that observed in npq4–1 (). Upon EL episode the FD in local and systemic WT leaves is slightly reduced in comparison to the control, but in systemic leaves this reduction is weaker. In npq4–1 control and DCMU treated leaves we observed similar chlorophyll fluorescence transients, comparable to DCMU treated WT leaves (). In local EL exposed leaves FD was much faster in comparison to npq4–1 control. This was also observed for npq4–1 systemic leaves. However, while the decay of fluorescence in npq4–1 local leaves was shorter than that observed in local WT leaves, the npq4–1 systemic leaves (undergoing SAA in LL) featured longer decay than observed in WT systemic leaves ().
Figure 2. Normalized representative fluorescence decays obtained for WT and npq4–1 leaves for the lowest excitation power (120 nW at the wavelength of 485 nm). We display the data measured for the reference (control), for leaves treated with 40 μM DCMU, for local leaves directly exposed to red excess light (620 nm, 3000 μmol photons m−2 s−1 for 1 h followed by 45 min of darkness), and for systemic leaves exposed to low white cold light (150 μmol photons m−2 s−1 for 1 h followed by 45 min of darkness). Solid points represent the measurement, while red curves are fits using a three-exponential decay model, as described in the text.
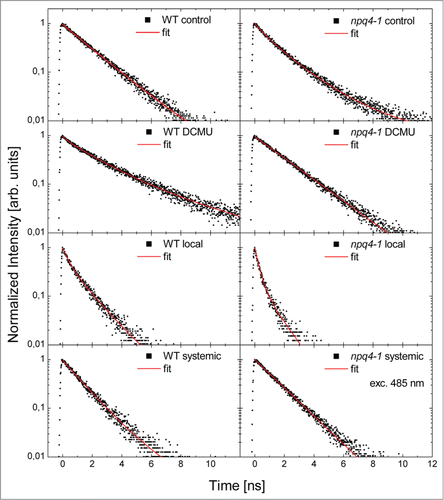
In , in addition to experimentally measured data (solid points), we also include the result of fitting the transients with combination of exponential decays (solid red lines). In our approach we aimed at using as few decay time constants as necessary for fitting all the measured transients, irrespective of the genotype or preparation. We find that when we use three exponential decays, this requirement is fulfilled and all the transients are approximated with R2 better than 98%. Consequently, the fitted function has the following form:(2)
(2) where ai is amplitude of i decay component and τi is lifetime of i decay component.
We point out that in many cases it was sufficient to use only two decay constants to obtain a good quality approximation of the experimental data. In such cases two of the three fitting decay times were equal. The fitting procedure, in addition to determining fluorescence decay constants, allowed us to obtain amplitudes associated with each of the decay component. The values of all the decay times extracted from all 200 measurements (two genotypes, four preparations, five excitation powers, and five points for each preparation) are summarized on a histogram in . We can conclude that the FD times are grouped into three subsets: between 0 and 1 ns, between 1 and 2.5 ns, and around 7 ns. The two subsets of the shorter decay times dominate the distribution, as the third subset consisting of the longest decay times accounts for approximately 5% of all the values. We note that the shortest decay time dominates (around 70% contribution) the fluorescence transients measured in npq4–1 local leaves exposed to EL. The first two values are comparable with the ones measured using another experimental approach.Citation10,11,29 Taking into account previously published results,Citation29 there is also a possibility that yet another much faster decay channel is present in the system, however, measuring decays below 50 ps is not achievable in our experimental setup. The approach we used for analyzing the fluorescence transients is also much simpler that the global analysis method e.g,Citation29 however limiting our detection to the PS II fluorescence only.
Figure 3. Histogram of decay times obtained by fitting a three-exponential decay model to fluorescence transients measured for WT and npq4–1. The histogram contains decay times extracted from fluorescence transients measured for five points across a leaf for all four preparations (control, DCMU, local, systemic), each treated with five excitation powers (12 μW, 3.8 μW, 1.2 μW, 380, nW, and 120 nW at the wavelength of 485 nm).
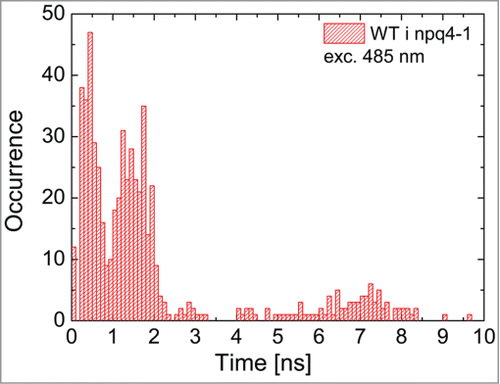
The correlation plots comparing the FD of three times and amplitudes associated with respective decay (Fig. S2) also display substantial differences between the two studied genotypes. First of all, the changes between control and DCMU-treated leaves are minute in the case of WT, with the exception of stronger contribution of the longest decay times. For local leaves of the rosettes we observe that for the WT there is still significant contribution of the longest decay times, completely absent in the case of the npq4–1. In addition, the shortest times extracted from the PSII fluorescence transients feature close to none dependence upon the excitation power for both WT and npq4–1 plants. Last but not least, the systemic leaves in a good approximation exhibit fluorescence decay time behavior that appears in between control and local leaves.
Post-stress chlorophyll fluorescence transient
The following experiment addresses a question of the role of NPQ (especially qE) and PsbS, in light acclimatory responses and returns to the equilibrium after EL. Chlorophyll fluorescence transients were measured at fixed time intervals after EL episode (0, 15, 30, 60, 120 min after EL) (). We show here the transients measured for the lowest excitation power (around 80 nW at 640 nm), an example of complete sets of five laser powers used are included in Figure S3.
Figure 4. Normalized representative fluorescence decays measured for WT and npq4–1 leaves exposed to red excess light for 1 h (EL, 620 nm, 3000 μmol photons m−2 s−1) followed by low white light exposure (150 μmol photons m−2 s−1) for different time intervals 0, 15, 30 min, 1 and 2 h after the EL episode. Then leaves were dark adapted for minimum 45 min. The transient measured at t = 0, t = 15 min, t = 30 min, t = 1 h, and t = 2 h after EL are shown and are compared with control measurement. Solid points represent the measurement, while red curves are fits using a three-exponential decay model, as described in the text.
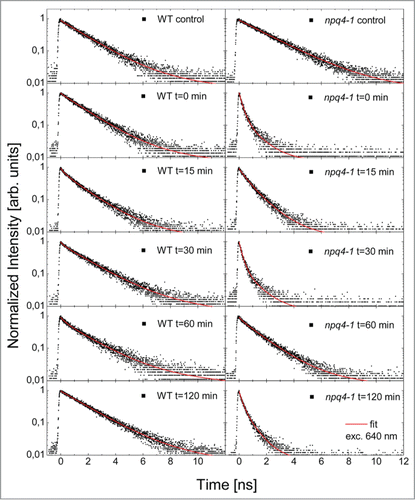
While solid points in correspond to measured data, lines represent fits using previously used three-exponential model (EquationEq. 1(1)
(1) ). In addition to the transients we display also the results of fitting in the form of amplitude-decay time correlation plots (). In the case of the WT the fluorescence transients feature essentially no change or small changes either directly after the EL or at several time intervals during the 2-h-long recovery period (). However, we observed dependence of the fluorescence transient upon the excitation power for every time interval: upon increase of the excitation power the decay was in all cases faster (). It indicates that in WT exposed to the EL the regulatory mechanism responsible for light adaptation preforms efficiently its function and participates in dissipation of the EEE. In a clear contrast, in the case of the npq4–1 we observed dramatic shortening of the FD, similarly as showed previously (). Just after EL (t = 0 min) the decay is extremely fast and features no dependence upon the excitation power (). This result is identical to previously discussed, indicating that the response of the npq4–1 plant is in principle independent upon the excitation wavelength. After 15 min from EL the decay gets slightly longer and there is some sensitivity of the decay to the excitation power. It can be clearly seen in the broadening of the distribution of the decay times located in the first, meaning the shortest, subset. Quite surprisingly, after another 15 min, the decays are similar to the ones measured at t = 0 min. Afterwards they get longer again after 1 h, but after 2 h from the EL the decays are very fast again. We found very little variation in the observed behavior between different points on the leaves for a given time interval.
Figure 5. Correlation plots of intensity of a decay component vs. decay time obtained for WT and npq4–1 leaves directly exposed to red excess light (EL, 620 nm, 3000 μmol photons m−2 s−1) followed by white low light exposure (150 μmol photons m−2 s−1) for different time intervals 0, 15, 30 min, 1 and 2 h after EL. Then leaves were dark adapted for minimum 45 min. Black, red, and green symbols correspond to the fastest (τ1), medium (τ2), and the slowest (τ3) decay components, respectively as described in the text. The data obtained at t = 0, t = 15 min, t = 30 min, t = 1 h, and t = 2 h after EL are shown and are compared with control measurement.
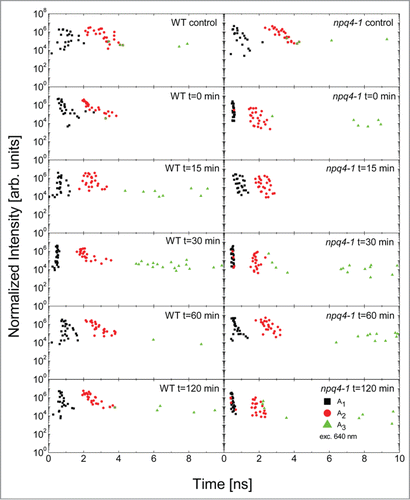
The transients measured in this experiment were also fitted using the three-exponential decay model and the obtained fluorescence decay times can also be grouped into three subsets, as discussed above (). The correspondence between the results displayed in confirms the universality of the approach described in this work, indicating that the fluorescence transients of PSII in Arabidopsis can be approximated with up to three decay constants yielding a very good fitting accuracy.
Discussion
Plants need to regulate absorbed photon fate in order to optimize photosynthesis and EEE quenching in highly variable light conditions. In a naive picture absorbed light energy distribution between photochemistry, chlorophyll fluorescence, and heat are in direct competition with each other.Citation9 The exact mechanism that enables plants to regulate and balance the rates of these processes under various light conditions has been moderately clarified.Citation6,10,11,22,29 SAA is an important light acclimatory mechanism that allows plants to globally optimize photosynthesis, molecular responses, and photosynthetic electron transport properties during partial exposure to EL.Citation7,28 NPQ components play important roles in optimization of absorbed photon fateCitation10,11,29 and in SAA.Citation7,Citation22-24,Citation28 Recently it was demonstrated that plants evolved sophisticated NPQ mechanisms that secure optimal plant fitness and productivity in challenging light environment.Citation6,10,11,22,29 The results of time-resolved fluorescence of PSII in Arabidopsis point out the key role of the PsbS protein in achieving appropriate regulation of energy dissipation in changing light conditions. In previous work we demonstrated that NPQ- and plastoquinone redox status-dependent local and systemic retrograde signaling for regulation of ASCORBATE PEROXIDASE 1 and 2, a robust molecular markers of EL and SAA responses, are deregulated during DCMU treatment and in the npq4–1 these genes are induced already in the non-stress LL-conditions.Citation7,28 Therefore in the present work we address the question of FD times regulation during EL, SAA, and after EL stress, since this regulation is directly influencing redox status of the plastoquinine pool, subsequent chloroplast retrograde signaling and light acclimatory responses.
Relatively small sensitivity of the fluorescence transient in Arabidopsis rosettes to EL episode and huge changes observed in npq4–1 or after DCMU treatment that reduce NPQ almost to zero, indicate the presence of highly efficient regulatory system of absorbed photon fate in higher plants that depends on PsbS activity.
The short decay () observed in npq4–1 local (EL exposed) leaves indicates that absorbed energy is immediately radiated back with high efficiency, as chlorophyll fluorescence. These results might explain why npq4–1 exhibit impaired growth in naturally fluctuating light in the field,Citation22 but not in controlled LL or high light laboratory conditions. Our observations also prove that NPQ and PsbS is required not only in directly EL-challenged leaves, but also in leaves undergoing SAA (LL-exposed) (). Importantly, the decay times measured for the systemic leaves lay in-between the local and control leaves (). This indicates that systemic changes of EEE dissipation can be observed not only in physiological time ranges, measured in the range from ms to min (),Citation7,28 but that these changes are determined by the processes that take place already during initial steps of absorbed photon energy distribution in PSII (). The intermediate decay observed for the systemic leaves indicates also that the part of the rosette not directly exposed to the EL episode, prepares for such a possibility by fine tuning of the fluorescence dynamics to potential EL threat. This reasoning is confirmed by FD measurements performed on npq4–1 systemic leaves ().
In the case of npq4–1 that already before EL challenge displays strongly reduced NPQ, the behavior of fluorescence dynamics for identical treatments is very different than for the WT. While the changes observed for DCMU-treated and systemic leaves are minute in respect to the control, and correlate with previous observations performed on plants grown under controlled LL conditions,Citation10,11,29 in our experimental system npq4–1 displays strong shortening of the FD ( and ). This observation is qualitatively in contrast to the results described in,Citation10,11,29 where the fluorescence exhibited longer decay upon high light illumination.Citation29 The difference may originate from substantially contrasting growth conditions used in both experiments: cultivating plants in highly variable greenhouse light conditions should influence the development of other acclimatory and regulatory mechanisms than that in controlled LL conditions. In fact, also the WT plants grown under variable conditions ( and ) exhibit shortened decay of fluorescence in local and systemic leaves as compared with the LL laboratory conditions.Citation29 Moreover, the Holzwarth groupCitation29 showed that a component of quenching (called Q2) is independent from PsbS and dependent on zeaxanthin. Since npq4–1 is not affected in zeaxanthin synthesis, this could be also the reason for the development of short lifetime in npq4–1. Regardless of the above differences, both the othersCitation10,11,29 and our experiments demonstrate how dynamic process is the light acclimation and how difficult is to experimentally address the question about the role of PsbS in light acclimatory responses.
The effect of EL illumination of local npq4–1 leaves is remarkably strong, as in this case the fluorescence transient features essentially no dependence upon increasing excitation power ( and ): the transients are clearly superimposed on top of each other. This stays in clear contrast to all other preparations, including WT local and systemic leaves, and npq4–1 systemic leaves where upon increasing the excitation power we observe further reduction of the FD (Fig. S3). This result demonstrates, that for the PsbS-deprived plants EL episode (in local and systemic leaves) deregulates EEE dissipation as fluorescence. Regardless of the strongly reduced level of light acclimatory mechanisms, the npq4–1 mutant still exhibits some accommodation behavior as the FD observed for the systemic leaves is longer than for the local leaves, but still shorter than for the control ( and ; Fig. S3).
The experiment performed at varied time intervals after EL ( and ), indicates that in the case of npq4–1 the energy dissipation is completely deregulated and even 2 h after the EL episode the mutant is far from reaching any sort of equilibrium. The “swinging behavior” of fluorescence transients means that optimization mechanisms of photon fate regulation after EL-LL transition are also impaired in npq4–1. In fact we found that about 6 h after the EL-LL transition the npq4–1 leaves feature transients similar to WT plants and the behavior remains unchanged at later time points (data not shown).
Conclusions
We conclude that PsbS plays a role in regulation of the absorbed photon energy fate not only in leaves directly exposed to EL, but also in leaves undergoing SAA and after EL stress. This observation has serious consequence because it indicates that there is an active networking between different PSII in different chloroplasts, cells, and leaves and indicate that higher plants evolved highly sophisticated system for coordinated global (local and systemic) adjustment of PSII LHC molecular functions such as EEE dissipation as fluorescence. In this system PsbS plays crucial and distinguished role. This role relies on global optimization of photosynthesis, EEE dissipation and the chloroplast redox retrograde signaling between different chloroplasts, cells, and plant leaves, in order to ensure optimal whole plant fitness and productivity in a highly variable light environment (e.g., moving clouds, shadows and flickering light in bushes). This finding has also an important consequences for understanding how plants globally regulate absorbed photon fate and subsequent molecular and physiological mechanisms. Moreover, for better understanding of these mechanisms and regulations of absorbed photon fate, measurements of chlorophyll fluorescence transients need to be performed and compared in plants grown under naturally variable light conditions.
Disclosure of Potential Conflicts of Interest
No potential conflicts of interest were disclosed.
KPSB_A_982018-FS3.pdf
Download PDF (278.7 KB)KPSB_A_982018-FS2.pdf
Download PDF (247.5 KB)KPSB_A_982018-FS1.pdf
Download PDF (13.2 KB)Funding
This work was supported by the Welcome/2008/1 and Welcome/2008/2 Programs operated within the framework of the Foundation for Polish Science, co-financed by the European Regional Development Fund given to S.K. and S.M., respectively.
References
- Asada K. THE WATER-WATER CYCLE IN CHLOROPLASTS: Scavenging of Active Oxygens and Dissipation of Excess Photons. Annu Rev Plant Physiol Plant Mol Biol 1999; 50:601-39; http://dx.doi.org/10.1146/annurev.arplant.50.1.601; PMID:15012221
- Ruban A, Lavaud J, Rousseau B, Guglielmi G, Horton P, Etienne AL. The super-excess energy dissipation in diatom algae: comparative analysis with higher plants. Photosynth Res 2004; 82:165-75; http://dx.doi.org/10.1007/s11120-004-1456-1; PMID:16151872
- Apel K, Hirt H. Reactive oxygen species: metabolism, oxidative stress, and signal transduction. Annu Rev Plant Biol 2004; 55:373-99; http://dx.doi.org/10.1146/annurev.arplant.55.031903.141701; PMID:15377225
- Laloi C, Stachowiak M, Pers-Kamczyc E, Warzych E, Murgia I, Apel K. Cross-talk between singlet oxygen- and hydrogen peroxide-dependent signaling of stress responses in Arabidopsis thaliana. Proc Natl Acad Sci U S A 2007; 104:672-7; http://dx.doi.org/10.1073/pnas.0609063103; PMID:17197417
- Van Breusegem F, Bailey-Serres J, Mittler R. Unraveling the tapestry of networks involving reactive oxygen species in plants. Plant Physiol 2008; 147:978-84; http://dx.doi.org/10.1104/pp.108.122325; PMID:18612075
- Li Z, Wakao S, Fischer BB, Niyogi KK. Sensing and responding to excess light. Annu Rev Plant Biol 2009; 60:239-60; http://dx.doi.org/10.1146/annurev.arplant.58.032806.103844; PMID:19575582
- Karpiński S, Reynolds H, Karpińska B, Wingsle G, Creissen G, Mullineaux P. Systemic signaling and acclimation in response to excess excitation energy in Arabidopsis. Science 1999; 284:654-7; http://dx.doi.org/10.1126/science.284.5414.654; PMID:10213690
- Niyogi KK. Safety valves for photosynthesis. Curr Opin Plant Biol 2000; 3:455-60; http://dx.doi.org/10.1016/S1369-5266(00)00113-8; PMID:11074375
- Baker NR. Chlorophyll fluorescence: a probe of photosynthesis in vivo. Annu Rev Plant Biol 2008; 59:89-113; http://dx.doi.org/10.1146/annurev.arplant.59.032607.092759; PMID:18444897
- Bonente G, Ballottari M, Truong TB, Morosinotto T, Ahn TK, Fleming GR, Niyogi KK, Bassi R. Analysis of LhcSR3, a protein essential for feedback de-excitation in the green alga Chlamydomonas reinhardtii. PLoS Biol 2011; 9:e1000577; http://dx.doi.org/10.1371/journal.pbio.1000577; PMID:21267060
- Brooks MD, Sylak-Glassman EJ, Fleming GR, Niyogi KK. A thioredoxin-like/β-propeller protein maintains the efficiency of light harvesting in Arabidopsis. Proc Natl Acad Sci U S A 2013; 110:E2733-40; http://dx.doi.org/10.1073/pnas.1305443110; PMID:23818601
- Amarnath K, Zaks J, Park SD, Niyogi KK, Fleming GR. Fluorescence lifetime snapshots reveal two rapidly reversible mechanisms of photoprotection in live cells of Chlamydomonas reinhardtii. Proc Natl Acad Sci U S A 2012; 109:8405-10; http://dx.doi.org/10.1073/pnas.1205303109; PMID:22586081
- Kim S, Pichersky E, Yocum CF. Topological studies of spinach 22 kDa protein of Photosystem II. Biochim Biophys Acta 1994; 1188:339-48; http://dx.doi.org/10.1016/0005-2728(94)90054-X; PMID:7803450
- Dominici P, Caffarri S, Armenante F, Ceoldo S, Crimi M, Bassi R. Biochemical properties of the PsbS subunit of photosystem II either purified from chloroplast or recombinant. J Biol Chem 2002; 277:22750-8; http://dx.doi.org/10.1074/jbc.M200604200; PMID:11934892
- Li X-P, Gilmore AM, Caffarri S, Bassi R, Golan T, Kramer D, Niyogi KK. Regulation of photosynthetic light harvesting involves intrathylakoid lumen pH sensing by the PsbS protein. J Biol Chem 2004; 279:22866-74; http://dx.doi.org/10.1074/jbc.M402461200; PMID:15033974
- Niyogi KK, Li X-P, Rosenberg V, Jung H-S. Is PsbS the site of non-photochemical quenching in photosynthesis? J Exp Bot 2005; 56:375-82; http://dx.doi.org/10.1093/jxb/eri056; PMID:15611143
- Caffarri S, Kouril R, Kereïche S, Boekema EJ, Croce R. Functional architecture of higher plant photosystem II supercomplexes. EMBO J 2009; 28:3052-63; http://dx.doi.org/10.1038/emboj.2009.232; PMID:19696744
- Ruban AV, Berera R, Ilioaia C, van Stokkum IH, Kennis JT, Pascal AA, van Amerongen H, Robert B, Horton P, van Grondelle R. Identification of a mechanism of photoprotective energy dissipation in higher plants. Nature 2007; 450:575-8; http://dx.doi.org/10.1038/nature06262; PMID:18033302
- Bergantino E, Segalla A, Brunetta A, Teardo E, Rigoni F, Giacometti GM, Szabò I. Light- and pH-dependent structural changes in the PsbS subunit of photosystem II. Proc Natl Acad Sci U S A 2003; 100:15265-70; http://dx.doi.org/10.1073/pnas.2533072100; PMID:14657329
- Ruban, AV, Horton, P. The Xanthophyll Cycle Modulates the Kinetics of Nonphotochemical Energy Dissipation in Isolated Light-Harvesting Complexes, Intact Chloroplasts, and Leaves of Spinach. Plant Physiol 1999, 119, 531-542
- Li X-P, Björkman O, Shih C, Grossman AR, Rosenquist M, Jansson S, Niyogi KK. A pigment-binding protein essential for regulation of photosynthetic light harvesting. Nature 2000; 403:391-5; http://dx.doi.org/10.1038/35000131; PMID:10667783
- Külheim C, Agren J, Jansson S. Rapid regulation of light harvesting and plant fitness in the field. Science 2002; 297:91-3; http://dx.doi.org/10.1126/science.1072359; PMID:12098696
- Mullineaux P, Ball L, Escobar C, Karpińska B, Creissen G, Karpiński S. Are diverse signalling pathways integrated in the regulation of arabidopsis antioxidant defence gene expression in response to excess excitation energy? Philos Trans R Soc Lond B Biol Sci 2000; 355:1531-40; http://dx.doi.org/10.1098/rstb.2000.0713; PMID:11128006
- Fryer MJ, Ball L, Oxborough K, Karpiński S, Mullineaux PM, Baker NR. Control of Ascorbate Peroxidase 2 expression by hydrogen peroxide and leaf water status during excess light stress reveals a functional organisation of Arabidopsis leaves. Plant J 2003; 33:691-705; http://dx.doi.org/10.1046/j.1365-313X.2003.01656.x; PMID:12609042
- Mateo A, Mühlenbock P, Rustérucci C, Chang CC-C, Miszalski Z, Karpińska B, Parker JE, Mullineaux PM, Karpiński S. LESION SIMULATING DISEASE 1 is required for acclimation to conditions that promote excess excitation energy. Plant Physiol 2004; 136:2818-30; http://dx.doi.org/10.1104/pp.104.043646; PMID:15347794
- Rossel JB, Wilson PB, Hussain D, Woo NS, Gordon MJ, Mewett OP, Howell KA, Whelan J, Kazan K, Pogson BJ. Systemic and intracellular responses to photooxidative stress in Arabidopsis. Plant Cell 2007; 19:4091-110; http://dx.doi.org/10.1105/tpc.106.045898; PMID:18156220
- Mühlenbock P, Szechyńska-Hebda M, Płaszczyca M, Baudo M, Mateo A, Mullineaux PM, Parker JE, Karpińska B, Karpiński S. Chloroplast signaling and LESION SIMULATING DISEASE1 regulate crosstalk between light acclimation and immunity in Arabidopsis. Plant Cell 2008; 20:2339-56; http://dx.doi.org/10.1105/tpc.108.059618; PMID:18790826
- Szechyńska-Hebda M, Kruk J, Górecka M, Karpińska B, Karpiński S. Evidence for light wavelength-specific photoelectrophysiological signaling and memory of excess light episodes in Arabidopsis. Plant Cell 2010; 22:2201-18; http://dx.doi.org/10.1105/tpc.109.069302; PMID:20639446
- Holzwarth AR, Miloslavina Y, Nilkens M, Jahns P. Identification of two quenching sites active in the regulation of photosynthetic light-harvesting studied by time-resolved fluorescence. Chem Phys Lett 2009; 483:262-7; http://dx.doi.org/10.1016/j.cplett.2009.10.085
- Wituszyńska W, Slesak I, Vanderauwera S, Szechynska-Hebda M, Kornas A, Van Der Kelen K, Mühlenbock P, Karpinska B, Mackowski S, Van Breusegem F, et al. Lesion simulating disease1, enhanced disease susceptibility1, and phytoalexin deficient4 conditionally regulate cellular signaling homeostasis, photosynthesis, water use efficiency, and seed yield in Arabidopsis. Plant Physiol 2013; 161:1795-805; http://dx.doi.org/10.1104/pp.112.208116; PMID:23400705
- Wituszyńska W, Gałązka K, Rusaczonek A, Vanderauwera S, Van Breusegem F, Karpiński S. Multivariable environmental conditions promote photosynthetic adaptation potential in Arabidopsis thaliana. J Plant Physiol 2013; 170:548-59; http://dx.doi.org/10.1016/j.jplph.2012.11.016; PMID:23287000
- Gawroński P, Górecka M, Bederska M, Rusaczonek A, Ślesak I, Kruk J, Karpiński S. Isochorismate synthase 1 is required for thylakoid organization, optimal plastoquinone redox status, and state transitions in Arabidopsis thaliana. J Exp Bot 2013; 64:3669-79; http://dx.doi.org/10.1093/jxb/ert203; PMID:23956412
- Krüger GHJ, Tsimilli-Michael M, Strasser RJ. Light stress provokes plastic and elastic modifications in structure and function of photosystem II in camellia leaves. Physiol Plant 1997; 101:265-77; http://dx.doi.org/10.1111/j.1399-3054.1997.tb00996.x
- Van Heerden PDR, Tsimilli-Michael M, Krüger GHJ, Strasser RJ. Dark chilling effects on soybean genotypes during vegetative development: parallel studies of CO2 assimilation, chlorophyll a fluorescence kinetics O-J-I-P and nitrogen fixation. Physiol Plant 2003; 117:476-91; http://dx.doi.org/10.1034/j.1399-3054.2003.00056.x; PMID:12675738
- Krajnik B, Schulte T, Piątkowski D, Czechowski N, Hofmann E, Maćkowski S. SIL-based confocal fluorescence microscope for investigating individual nanostructures. Cent. Eur. J. Phys. 2011; 9:293-9; http://dx.doi.org/10.2478/s11534-010-0098-5
- Demmig-Adams B, Adams WW. Photoprotection and Other Responses of Plants to High Light Stress. Annu Rev Plant Physiol Plant Mol Biol 1992; 43:599-626; http://dx.doi.org/10.1146/annurev.pp.43.060192.003123
- Yruela I, Pueyo JJ, Alonso PJ, Picorel R. Photoinhibition of photosystem II from higher plants. Effect of copper inhibition. J Biol Chem 1996; 271:27408-15; http://dx.doi.org/10.1074/jbc.271.44.27408; PMID:8910320
- Ballottari M, Dall’Osto L, Morosinotto T, Bassi R. Contrasting behavior of higher plant photosystem I and II antenna systems during acclimation. J Biol Chem 2007; 282:8947-58; http://dx.doi.org/10.1074/jbc.M606417200; PMID:17229724