Abstract
Salicylic acid (SA) occupies a key role as a hormone central to both plant resistance to bacterial pathogens and tolerance of abiotic stresses. Plants at high elevation experience colder temperatures and elevated UV levels. While it has been predicted that SA concentrations will be higher in plants from high elevation populations, few studies have addressed this question. Here, we asked how concentrations of SA vary in natural populations of Arabidopsis thaliana collected across an elevational gradient on the Iberian Peninsula. In a series of common garden experiments, we found that constitutive SA concentrations were highest in genotypes from the low elevation populations. This result was in the opposite direction from our prediction and is an exception to the general finding that phenolic compounds increase with increasing elevation. These data suggest that high constitutive SA is not associated with resistance to cold temperatures in these plants. Furthermore, we also found that leaf constitutive camalexin concentrations, an important defense against some bacterial and fungal enemies, were highest in the low elevation populations, suggesting that pathogen pressures may be important. Further examination of this elevational cline will likely provide additional insights into the interplay between phenolic compounds and biotic and abiotic stress.
Introduction
At a broad geographic scale, plant assemblages can be predicted largely by differences in annual temperature and precipitation.Citation1 Plants within a climatic region converge evolutionarily on particular morphological, physiological, and phenological themes, which are particularly notable in association with the increased risk of cold stress with increasing latitude or elevation.Citation2,3 While hormones regulate much of the variety that we see in plant morphology and physiology, the extent of variation in the production of plant hormones in relation to latitudinal and elevational clines remains largely unknown.Citation4
High elevation environments present significant challenges from both abiotic and biotic stress. Notable abiotic stresses include the increased exposure to ultraviolet radiation (UV) and increased frequency of freezing temperatures.Citation5 It is not surprising therefore that the abundance and diversity of most taxonomic groups are lower in high elevation habitats.Citation5-8 While the diversity of pathogens is likely to be lower at high elevations,Citation7 their overall impact on hosts could exceed those at low elevation for several reasons. Because plant diversity is low in these habitats,Citation5 outbreaks of pathogens are transmitted more readily among hosts.Citation8,9 Because predators are less abundant in these habitats,Citation6 there is less potential for top-down control of outbreaking pests.Citation10 Finally, the virulence of some plant pathogens such as snow blight fungiCitation8,9 and ice nucleating bacteriaCitation11 is facilitated by cold conditions.
Plants at high elevations have been shown to exhibit elevated constitutive concentrations of phenolic compounds, particularly flavonoids and phenolic glycosides.Citation12-16 These compounds consist of aromatic rings that absorb UV radiation and in some cases have been shown to reduce the deleterious effects of oxidative damage to plant tissues.Citation17 Some of these compounds have been shown to increase in concentrations in plants following treatment with cold temperaturesCitation15,18 and have been correlated recently with high antibiotic activity against pathogenic bacteria.Citation16 However, the hormonal dynamics underpinning these elevational differences in phenolic chemistry have not been identified previously.
Perhaps the most widely represented phenolic in plants is the major plant hormone, salicylic acid (SA). SA has a central role in orchestrating the cascade of plant induced defenses against bacterial pathogens and some insect and fungal pests.Citation19 One of the important defense compounds against bacterial and fungal infection is camalexin, 3-thazole-2-yl-indole, which, like SA, is also derived from chorismate.Citation20 Interestingly, SA also has notable importance in thermal regulation, owing to its involvement in decoupling electron transport in mitochondria and the release of energy in the form of heat.Citation21 SA has particularly strong effects on thermal regulation in the arum family, Araceae,Citation21 but cold temperatures have been shown to induce accumulation of SA also in the model plant, Arabidopsis thaliana.Citation22,23 On the basis of these studies, a recent reviewCitation4 predicted that SA concentrations would likely be higher in A. thaliana at high elevations.
In the current study, we asked specifically whether SA concentrations increase with increasing elevation across a key region of endemism of A. thaliana on the Iberian PeninsulaCitation24 and how these concentrations relate to particular aspects of climatic variation. If leaf constitutive SA concentrations were found to be higher at high elevations, then that would be consistent with an important role of the abiotic or biotic stresses associated with the high elevation habitats. We then asked how a high temperature treatment would alter leaf concentrations of SA and finally whether leaf camalexin concentrations also varied across the elevational gradient.
Materials and Methods
Arabidopsis material and growth conditions
Characteristics of the 15 source populations on the Iberian Peninsula (, Table S1) have been described extensively elsewhereCitation24 and seed stocks are publically available at the Arabidopsis Biological Resource Center of Ohio State University (ABRC CS#78884). The first climate principle component (Climate PC1) explains 71% of climate variation across the elevation gradient associated with the source populationsCitation24 (, Table S1).
Figure 1. Collection of 15 populations of A. thaliana across an elevational transect on the Iberian peninsula [as shown in]Citation24 showing (A) populations used in each of the 2 experiments and (B) the relationship between climate PC1 and elevation.
![Figure 1. Collection of 15 populations of A. thaliana across an elevational transect on the Iberian peninsula [as shown in]Citation24 showing (A) populations used in each of the 2 experiments and (B) the relationship between climate PC1 and elevation.](/cms/asset/a648ec92-0263-4cff-a5ca-f744078ffad6/kpsb_a_992741_f0001_oc.gif)
To assess leaf concentrations of SA across the elevational gradient, we first performed a common garden experiment in 2009 using seeds from 8 source populations across the elevation gradient and including 9 genotypes from each population. For four of the populations (ARB, POB, MUR, and VDM), we measured one replicate plant per genotype for SA for a total of 36 plants. For four of the populations (BAR, HOR, ALE, and VIE), we had more available seeds and for these populations we had 2 replicate plants per genotype for constitutive measurement of SA, for a total of 72 plants and a cumulative total of 108 plants (Table S2). We surface sterilized seeds and then planted them onto 36-cell flats filled with Promix-BX potting soil and placed them for one week in a 4°C cold room for cold stratification. We then transferred the flats to a growth room at the University of Pittsburgh with a constant temperature of 20°C and a 14 hr day length with light levels of 350 μmol m−2 sec−1 provided by a 1:1 mixture of sodium and metal halide lamps. At seven days past germination, we thinned to 3 seedlings per cell and at 10 d past germination, we thinned to one seedling per cell. We then subdivided the cells and assigned each plant to a specific treatment and then completely randomized and redistributed the cells across the growth room bench. Cells were moved at least once per week within the growth chamber to reduce positional effects.
To assess the direct effects of a heat treatment on SA concentrations, we also grew an additional 2 replicates per genotype for the same 4 populations (BAR, HOR, ALE, and VIE) for an additional 72 plants that were randomized along with the other replicates, but exposed to a 44°C treatment in the dark for 3 hrs beginning on Day 25. Control plants also placed in the dark for 3 hr, but were kept at the ambient temperature of 20°C. We then re-randomized the heat treatment and control plants together in the growth room and harvested all 180 plants on Day 30 of growth when all plants had rosettes and bolting had not yet been initiated.
To reassess SA concentrations across the elevational gradient and to measure leaf camalexin concentrations, we conducted an additional common garden experiment in 2013 using seeds from the larger set of 15 populations. For these measurements, we included one replicate of each of 4 genotypes from each of these 15 populations, for a total of 60 plants. Seeds were cold stratified at 4°C for 5 d and then grown at 22°C for 3 weeks (16 hr light/8 hr dark) in growth chambers. Rosettes were then exposed to a 4-week vernalization at 4°C to synchronize flowering time followed by 22°C for 3 weeks (16 hr light/8 hr dark) at which point they began flowering. Plants were randomly distributed in the growth chamber and were rotated every 2 d to minimize within-chamber effects. Leaf materials for the measurement of SA were collected at the start of flowering (Table S3).
Measurement of leaf SA and camalexin concentrations
To measure leaf SA and camalexin concentrations we first harvested rosettes, froze them in liquid nitrogen, and stored them at −80°C prior to analysis. In the 2009 experiment, we extracted directly from the frozen tissue, and calculated SA concentrations on a wet mass basis, whereas in the 2013 experiment we lyophilized the tissue to dryness first and then calculated concentrations on a dry mass basis (µg/g leaf dry mass). To control for run to run variation, we added an internal control of 1 μg of O-Anisic Acid (Sigma # 169978) consisting of 100 μl from a stock solution of 10 μg of O-Anisic Acid in 1 ml of 100% methanol. To extract SA, we weighed leaf tissues, suspended them in 3 ml of 90% methanol, rocked the tubes in a shaker at room temperature for 24 hr, and transferred the supernatant to a new tube, as described previously.Citation25,26 We then resuspended the pellet in 3 ml of 100% methanol, vortexed, rocked the tubes again for 24 hr, and combined the 2 supernatant fractions. To capture both the free SA and the SA conjugated to sugar, we then split each sample into 2 equal volumes into 2 screwcap tubes and placed the tubes in a fume hood until dry, which required at least 48 hr. We used the first aliquot to measure free SA, and the second to measure total SA, which includes both the free and sugar-conjugated SA. To cleave the sugar from the SA glucoside, we added 40U of b-glucosidase enzyme (Sigma # 0395) in 400 μl of 100 mM sodium acetate buffer (pH 5.5) to the total SA aliquots. The paired free SA aliquot received 400 μl of buffer only. All tubes incubated overnight at 37°C. To deactivate the enzyme, we then added 400 μl of 10% trichloroacetic acid to all samples. To partition the SA from other compounds, we then extracted each tube twice with 1 ml of an organic solvent mixture (100:99:1 of ethyl acetate: cyclopentane: 2-propanol) and vortexed. We then collected the 2 organic phase fractions in a centrifuge tube, which we then placed in a fume hood until dry, which required at least 24 hrs. We resuspended the organic fraction in 600 μl of 55% methanol, vortexed, and rocked overnight. To filter out impurities, we passed the supernatant through 0.2 μm nylon spin-prep membrane filters (Fisher #07–200–389). To measure SA concentrations by high performance liquid chromatography (HPLC), we used an HP1100 system with a 4.6 × 150 mm Agilent Eclipse XDB C-18 column and fluorescence detector, with excitation at 301 nm and emission at 412 nm for SA, excitation at 301 nm and emission at 386 nm for camalexin, excitation at 301 nm and emission at 365 nm for O-anisic acid. The flow rate was 1 ml/min and the solvents were (A) 100% methanol and (B) 0.5% acetic acid in water. Each run consisted of 30% A and 70% B for the first 5 minutes, increasing to 40% A at 7.5 min and 60% A at 15 min, returning to 30% A at 18 min. To calculate concentrations of free SA, total SA, and camalexin, we divided the peak area of each compound by the product of the peak area of the O-Anisic acid internal standard and the sample mass in grams.
Results
To assess the hypothesis that SA concentrations increase with increasing elevation across a key region of endemism of A. thaliana on the Iberian Peninsula (), we conducted 2 large common garden experiments which allowed us to test the genotypes from the different source populations together under a common set of environmental conditions, and found that SA concentrations declined significantly with increasing elevation in both experiments, contrary to the predictions. In the larger experiment conducted in 2013 and including 15 source populations, we found that genotypes from the high elevation source populations produced an order of magnitude less free SA per dry mass relative to populations from lower elevations (R2 = 38.6%, P = 0.013, ). There was also a significant decline in total SA per dry mass with increasing elevation (R2 = 24.7%, P = 0.059, Fig. S1A). In the 2009 common garden experiment conducted on the smaller set of 8 populations, we found that genotypes from the low elevation source populations did not differ significantly in free SA (R2 = 30.9%, P = 0.15, Fig. S1C), but produced roughly 2-fold more total SA per wet mass relative to the populations from the higher elevations (R2 = 69.9%, P = 0.010, Fig. S1E).
Figure 2. Regressions of population means from the 2013 common garden experiment showing relationships between leaf free salicylic acid concentration and (A) elevation in meters and (B) climate PC1, where higher values represent colder temperatures and greater rainfall. Shown are population means (+/− 1SE) for 10-week-old plants representing 4 maternal families per population.
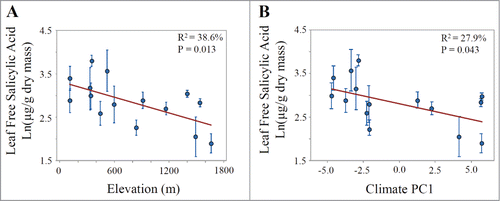
Free SA per dry mass was strongly negatively correlated with climate PC1 in the 2013 experiment (R2 = 27.9%, P = 0.043, ) where higher values of PC1 corresponded to colder and wetter habitats. The climate PC2 axis did not explain significant variation in leaf free SA and did not improve the fit of the model when included with climate PC1 (Table S4). Total SA was not correlated significantly with climate PC1 (R2 = 15.9%, P = 0.14, Fig. S1B) or climate PC2 (R2 = 0.12%, P = 0.90). In the 2009 experiment, free SA was not correlated with climate PC1 (R2 = 31.3%, P = 0.14, Fig. S1D), but total SA concentration was correlated significantly with climate PC1 (R2 = 67.1%, P = 0.013, Fig. S1F).
To assess whether a heat treatment itself could induce changes in SA concentrations, we also challenged plants from 4 populations (BAR, HOR, ALE, and VIE) along the elevation gradient with exposure to 44°C for 3 hr. Leaf free and total SA concentrations decreased by 10.8% and 11.2%, respectively in the heat treated plants relative to the control plants (F1,64 = 6.3, P = 0.015, and F1,64 = 5.1, P = 0.026, Fig. S2, respectively) and these responses to the heat treatment did not differ significantly among the 4 populations, as indicated by the non-significant population by treatment interaction term in the analysis of variance.
Figure 3. (A) Effect of a heat treatment of 44°C for 3 hr on leaf concentrations of free SA in plants from 4 source populations: BAR (429 m), HOR (431 m), ALE (1225 m), and VIE (1600 m). Each population was represented by 8 or 9 independent maternal lines, each represented by 2 replicates in each of the 2 treatments, for a total of 144 plants (Table S5). (B) Regression of population means of natural log transformed leaf camalexin concentrations from 11 populations in the 2013 common garden experiment as a function of the elevation of the source population in meters (Table S6). Four populations (PIN, RAB, BAR, and HOR) lacked constitutive leaf camalexin concentrations and were excluded from the regression. Significant P values are indicated in bold.
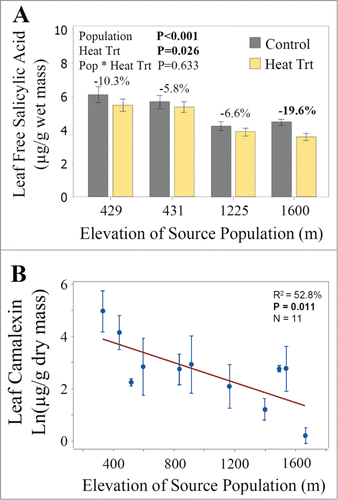
To determine whether levels of the anti-bacterial defenses also changed across the elevation gradient, we then assessed leaf camalexin concentrations in the 11 populations that had non-zero concentrations of this compound in the 2013 experiment. Interestingly, the 4 populations lacking camalexin production (PIN, RAB, BAR, and HOR) were all located at low elevations. For the 11 populations that had non-zero levels of camalexin production, we found that leaf constitutive concentrations of camalexin declined significantly with increasing elevation (R2 = 52.8%, P = 0.011, , Table S6).
Discussion
SA is perhaps the most widely distributed phenolic compound in plants and is central to defense against bacterial pathogens.Citation19 A recent reviewCitation4 predicted that SA concentrations would likely be higher in plants at high elevations, given evidence that cold temperatures directly stimulate SA production in laboratory plantsCitation22,23 and that concentrations of phenolics such as flavonoids and phenolic glycosides are typically very high in leaves of high elevation plants.Citation12-16 We report here that SA concentrations in Arabidopsis thaliana from wild populations on the Iberian Peninsula do not support the predicted pattern. Indeed, the cline of SA was in the opposite direction from what we expected, with concentrations higher on average in low elevation populations. These findings are robust in that they were observed in 2 separate common garden experiments, conducted using both frozen and lyophilized leaf tissues. To our knowledge, this is the first evidence of a geographical cline in SA concentrations in plants.
There are several possible explanations for the unexpected pattern of a decrease in SA with increasing elevation observed here. First, warm temperatures might directly stimulate plant production of SA. Many studies have shown consistently that warm temperatures actually suppress SA concentrations in A. thaliana and other plants [reviewed in].Citation4 To assess the possibility that these wild Spanish populations exhibited a different pattern, we directly exposed plants of 4 of the Spanish populations to an elevated temperature treatment of 44°C for 3 hr and found that the high temperature treatment reduced SA concentrations (), in agreement with the general pattern in the literature.Citation27 Thus, the high SA concentrations that we observe in low elevation plants cannot be explained directly by the warmer temperatures found at low elevations. However, the ability of plants to tolerate heat stress may require high levels of SA, as has been suggested in studies of acquired high temperature tolerance in laboratory experiments with seedlings of A. thaliana.Citation28 To our knowledge, no experimental work has examined the relationship of constitutive SA expression levels in wild plants to their survival under high temperature stress. Whether heat stress survival may explain the climate/elevation cline in SA observed here remains to be explored.
A second possible explanation for why SA concentrations would decrease with increasing elevation relates to source-sink dynamics. All phenolic compounds, ranging from simple compounds such as SA to complex polyphenolics, are derived from a common resource pool of chorismate.Citation29 If the complex polyphenolics have greater efficacy in protecting plants from UV stress, then plants at high elevations may shunt more of the chorismate toward this sink and away from the production of SA. There is currently insufficient data to address this hypothesis. Future assessment of the concentrations of chorismate and UV-B absorbing phenolics, such as flavonoids, across this elevational gradient would increase our ability to understand source-sink relationships in this species.
A third possible explanation for our pattern of higher SA levels in low elevation populations relates to the likelihood that overall enemy pressures are likely to be greater in low elevation environments. To assess additional evidence for this possibility, we also measured concentrations of camalexin, an important chemical defense against microbes,Citation20 and found that these concentrations were also highest in the low elevation populations (). Under this scenario, plants at the lower elevations have evolved higher constitutive levels of SA and camalexin as a consequence of strong selection by enemies. Bacterial diversity and abundance is likely to be higher at low elevations.Citation7 Furthermore, recent studies have found that pathogen loads on plants are higher at lower elevationsCitation30 and resistance to enemies is lower at high elevations.Citation31 It is possible that higher SA concentrations are needed in plants at low elevations to combat pathogens in those habitats, but at this point, manipulative experiments are needed to assess the relative impacts of these key environmental variables.
Concentrations of SA for A. thaliana previously reported in the literature have been typically around 1 µg/g wet mass of leaf tissue,Citation25 which is lower than the average value for our data of 4.7 µg/g wet mass for free SA from the 2009 experiment (95% confidence interval of 4.4 to 5.0 µg/g wet mass, Table S2). We have carefully checked and rechecked our calculations and methodology and see no explanation for this difference. We are confident that the elevational pattern is robust, despite this lack of congruence. These natural Spanish accessions may have constitutively higher SA concentrations than have been described previously in the literature; however, future studies should include the Col-0 and Ws-0 lines also for better calibration of our findings relative to those of other laboratories.
In other work, we have found that SA and camalexin concentrations do explain some variation in plant resistance to attack by a bacterial pathogen.Citation26 Thus, our finding of a geographic cline in SA and camalexin concentrations may contribute to understanding how plant resistance to bacterial infection is distributed in natural populations. Geographic clines in plant compounds, such as those examined here, are likely to provide information that will be important for understanding how plant populations will be affected by a warming climate.Citation32
Disclosure of Potential Conflicts of Interest
No potential conflicts of interest were disclosed.
SupplementalTables_Figures.doc
Download MS Word (1.8 MB)Acknowledgments
We thank 3 anonymous reviewers for their comments on the manuscript. We thank Shelley Valle for assistance in planting seeds and extracting leaf samples for analysis of SA and Marnin Wolfe for assistance with the heat treatment. The Arabidopsis accessions used in this study are maintained and provided by the Tonsor lab, University of Pittsburgh. Genetic lines used in this study are available from the Arabidopsis Biological Resource Center at the Ohio State University (CS# 78884).
Supplemental Material
Supplemental data for this article can be accessed on the publisher's website.
Funding
This work was supported by US National Science Foundation Grants IOS-0809171 and IOS-1120383 (S.J.T.) and 1050138 (M.B.T.).
References
- Whittaker RH. Communities and ecosystems, New York: Macmillan; 1970. 352 p.
- Schimper AFW. Plant-geography upon a physiological basis. Oxford: Clarendon Press; 1903. 839 p.
- Clausen J & Hiesey WM. Experimental studies on the nature of species IV. Genetic structure of ecological races. Washington, DC: Carnegie Institute of Washington; 1958. 312 p.
- Traw MB, Bergelson J. Plant immune system incompatibility and the distribution of enemies in natural hybrid zones. Curr Opin Plant Biol 2010; 13:466-71; PMID:20494612; http://dx.doi.org/10.1016/j.pbi.2010.04.009
- Körner C. Alpine plant life: functional plant ecology of high mountain ecosystems. 2nd edition. Berlin: Springer; 2003. 349 p.
- Reymond A, Purcell J, Cherix D, Guisan A, Pellissier L. Functional diversity decreases with temperature in high elevation ant fauna. Ecol Entomol 2013; 38:364-73; http://dx.doi.org/10.1111/een.12027
- Bryant JA, Lamanna C, Morlon H, Kerkhoff AJ, Enquist BJ, Green JL. Microbes on mountainsides: contrasting elevational patterns of bacterial and plant diversity. Proc Nat Acad Sci 2008; 105:11505-11; PMID:18695215; http://dx.doi.org/10.1073/pnas.0801920105
- Burdon JJ, Wennström A, Ericson L, Müller WJ, Morton R. Density-dependent mortality in Pinus sylvestris caused by the snow blight pathogen Phacidium infestans. Oecologia 1992; 90:74-9; http://dx.doi.org/10.1007/BF00317811
- Barbeito I, Brücker RL, Rixen C, Bebi P. Snow fungi-induced mortality of Pinus cembra at the alpine treeline: evidence from plantations. Arctic Antarctic Alpine Res 2013; 45:455-70; http://dx.doi.org/10.1657/1938-4246-45.4.455
- Haukioja E. Plant defenses and population fluctuations of forest defoliators: mechanism-based scenarios. Ann Zool Fennini 2005; 42:313-25.
- Morris CE, Sands DC, Vinatzer BA, Glaux C, Guilbaud C, Buffiere A, Yan S, Dominguez H, Thompson BM. The life history of the plant pathogen Pseudomonas syringae is linked to the water cycle. ISME J 2008; 2:321-34; PMID:18185595; http://dx.doi.org/10.1038/ismej.2007.113
- Zidorn C, Schubert B, Stuppner H. Altitudinal differences in the contents of phenolics in flowering heads of three members of the tribe Lactuceae (Asteraceae) occurring as introduced species in New Zealand. Biochem System Ecol 2005; 33:855-72; http://dx.doi.org/10.1016/j.bse.2004.12.027
- Rieger G, Mu¨ller M, Guttenberger H, Bucar F. Influence of altitudinal variation on the content of phenolic compounds in wild populations of Calluna vulgaris, Sambucus nigra, and Vaccinium myrtillus. J Agric Food Chem 2008; 56:9080-6; PMID:18788745; http://dx.doi.org/10.1021/jf801104e
- Spitaler R, Winkler A, Lins I, Yanar S, Stuppner H, Zidorn C. Altitudinal variation of phenolic contents in flowering heads of Arnica montana cv. ARBO: a 3-year comparison. J Chem Ecol 2008; 34: 369-75; PMID:18259818; http://dx.doi.org/10.1007/s10886-007-9407-x
- Albert A, Sareedenchai V, Heller W, Seidlitz HK, Zidorn C. Temperature is the key to altitudinal variation of phenolics in Arnica montana L. cv. ARBO. Oecologia 2009; 160:1-8; PMID:19194724; http://dx.doi.org/10.1007/s00442-009-1277-1
- Martz F, Peltola R, Fontanay S, Duval RE, Julkunen-Tiitto R, Stark S. Effect of latitude and altitude on the terpenoid and soluble phenolic composition of juniper (Juniperus communis) needles and evaluation of their antibacterial activity in the boreal zone. J Agric Food Chem 2009; 57:9575-84; PMID:19772344; http://dx.doi.org/10.1021/jf902423k
- Li J, Ou-Lee T, Raba R, Amundson RG, Last RL. Arabidopsis flavonoid mutants are hypersensitive to UV-B irradiation. Plant Cell 1993; 5:171-9; PMID:12271060; http://dx.doi.org/10.1105/tpc.5.2.171
- Bilger W, Rolland M, Nybakken L. UV screening in higher plants induced by low temperature in the absence of UV-B radiation. Photochem Photobiol Sci 2007; 6:190-5; PMID:17277843; http://dx.doi.org/10.1039/b609820g
- Vlot AC, Dempsey DMA, Klessig DF. Salicylic acid, a multifaceted hormone to combat disease. Ann Rev Phytopathol 2009; 47:177-206; PMID:19400653; http://dx.doi.org/10.1146/annurev.phyto.050908.135202
- Ren D, Liu Y, Yang K, Han L, Mao G, Glazebrook J, Zhang S. A fungal-responsive MAPK cascade regulates phytoalexin biosynthesis in Arabidopsis. Proc Nat Acad Sci 2008; 105:5638-43; PMID:18378893; http://dx.doi.org/10.1073/pnas.0711301105
- Raskin I, Turner IM, Melander WR. Regulation of heat production in the inflorescences of an Arum lily by endogenous salicylic acid. Proc Natl Acad Sci 1989; 86:2214-8; http://dx.doi.org/10.1073/pnas.86.7.2214
- Kaplan F, Kopka J, Haskell DW, Zhao W, Schiller KC, Gatzke N, Sung DY, Guy CL. Exploring the temperature-stress metabolome of Arabidopsis. Plant Physiol 2004; 136:4159-68; PMID:15557093; http://dx.doi.org/10.1104/pp.104.052142
- Leyva A, Jarillo JA, Salinas J, Martinez-Zapater JM. Low temperature induces the accumulation of phenylalanine ammonia-lyase and chalcone synthase mRNAs of Arabidopsis thaliana in a light-dependent manner. Plant Physiol 1995; 108:39-46; PMID:12228452
- Wolfe MD, Tonsor SJ. Adaptation to spring heat and drought in northeastern Spanish Arabidopsis thaliana. New Phytologist 2014; 201, 323-34; PMID:24117851; http://dx.doi.org/10.1111/nph.12485
- Mukherjee M, Larrimore KE, Ahmed NJ, Bedick TS, Barghouti NT, Traw MB, Barth C. Ascorbic acid deficiency in Arabidopsis induces constitutive priming that is dependent on hydrogen peroxide, salicylic acid, and the NPR1 gene. Mol. Plant Microbe-Interact 2014; 23:340-51; http://dx.doi.org/10.1094/MPMI-23-3-0340
- Zhang N, Lariviere A, Tonsor SJ, Traw MB. Constitutive camalexin production and environmental stress response variation in Arabidopsis populations from the Iberian Peninsula. Plant Sci 2014; 225:77-85; PMID:25017162; http://dx.doi.org/10.1016/j.plantsci.2014.05.020
- Yang S, Hua J. A haplotype-specific resistance gene regulated by BONZAI1 mediates temperature-dependent growth control in Arabidopsis. Plant Cell 2004; 16:1060-71; PMID:15031411; http://dx.doi.org/10.1105/tpc.020479
- Larkindale J, Hall JD, Knight MR, Vierling E. Heat stress phenotypes of Arabidopsis mutants implicate multiple signaling pathways in the acquisition of thermotolerance. Plant Physiol 2005; 138:882-97; PMID:15923322; http://dx.doi.org/10.1104/pp.105.062257
- Chen Z, Zheng Z, Huang J, Lai Z, Fan B. Biosynthesis of salicylic acid in plants. Plant Signal Behav 2009; 4:493-6; PMID:19816125; http://dx.doi.org/10.4161/psb.4.6.8392
- Springer YP. Clinal resistance structure and pathogen local adaptation in a serpentine flax–flax rust interaction. Evolution 2007; 61, 1812-22; PMID:17683425; http://dx.doi.org/10.1111/j.1558-5646.2007.00156.x
- Pellissier L, Roger A, Bilat J, Rasmunn S. High elevation Plantago lanceolata plants are less resistant to herbivory than their low elevation conspecifics: is it just temperature? Ecography 2014; 37:950-9; http://dx.doi.org/10.1111/ecog.00833
- Boland G, Melzer M, Hopkin A, Higgins V, Nassuth A. Climate change and plant diseases in Ontario. Canadian J Plant Pathol 2004; 26:335-50; http://dx.doi.org/10.1080/07060660409507151