Abstract
Phytol is a diterpene alcohol of medicinal importance and it also has potential to be used as biofuel. We found over production of phytol in Nostoc punctiforme by expressing a 2-Methyl-3-buten-2-ol (MBO) synthase gene. MBO synthase catalyzes the conversion of dimethylallyl pyrophosphate (DMAPP) into MBO, a volatile hemiterpene alcohol, in Pinus sabiniana. The result of enhanced phytol production in N. punctiforme, instead of MBO, could be explained by one of the 2 models: either the presence of a native prenyltransferase enzyme with a broad substrate specificity, or appropriation of a MBO synthase metabolic intermediate by a native geranyl diphosphate (GDP) synthase. In this work, an expression vector with an indigenous petE promoter for gene expression in the cyanobacterium N. punctiforme was constructed and MBO synthase gene expression was successfully shown using reverse transcriptase (RT)-PCR and SDS-PAGE. Gas chromatography – mass spectrophotometry (GC-MS) was performed to confirm phytol production from the transgenic N. punctiforme strains. We conclude that the expression of MBO synthase in N. punctiforme leads to overproduction of an economically important compound, phytol. This study provides insights about metabolic channeling of isoprenoids in cyanobacteria and also illustrates the challenges of bioengineering non-native hosts to produce economically important compounds.
Keywords:
Abbreviations
BCS | = | bathocuproine disulphonate |
DMAPP | = | dimethylallyl pyrophosphate |
GC-MS | = | gas chromatography-mass spectrometry |
MBO | = | 2-Methyl-3-buten-2-ol |
MEP | = | Methyl-erythritol-4-phosphate |
MVA | = | mevalonate |
Introduction
Plants synthesize a variety of compounds that include short chain, low molecular weight isoprenoids.Citation1 Some pine species are known to express methylbutenol synthase. This enzyme is a soluble protein of molecular size of 70 kD that catalyzes the conversion of dimethylallyl pyrophosphate (DMAPP) into the 5-carbon volatile alcohol, 2-Methyl-3-buten-2-ol (MBO).Citation2 MBO is one of the smallest compounds in the isoprenoid family that is released by these pines into the atmosphere. Production of small isoprenoids may be constitutive or stress-induced in plants to protect them against different abiotic stressesCitation3-5 as well as biotic stresses.Citation6 Isoprene, a 5C volatile isoprenoid similar to MBO, has been demonstrated to interact and stabilize chloroplast membranes under high temperature conditions indicating a thermoprotective role.Citation7-9 Way et al. (2011) has also shown that emission of isoprene enhances tolerance to high-light stress.Citation10 The role of MBO production specifically in some pine species is still unclear but it is thought to play a similar role as isoprene since MBO emission from plants increases upon exposure to high light and temperature.Citation11
MBO is a promising compound that can be used for biosynthesis of value added products and biofuels,Citation12 but harvesting it from trees is not practical. Instead production in artificial hosts under controlled conditions where the volatile compound can be harvested would be ideal. In addition to MBO, larger isoprenoid compounds are also of economic importance and are used as active ingredients in different flavor, fragrance and medicinal products.Citation13,14 A number of different high-value isoprenoids are known, including artemisinin which is used as an antimalarial drugCitation15 and taxol, used as an anticancer compound.Citation16 Both of these pharmaceutically important compounds have been produced using metabolically engineered microbial platforms. Another important group of isoprenoids are the diterpene alcohols commonly known as phytol. Phytols have been demonstrated to have a number of pharmaceutically important properties including: antinociceptive and antioxidantCitation17; anti-inflamatory and antiallergicCitation18; antimicrobial activity against Mycobacterium tuberculosisCitation19,20 and Staphylococcus aureusCitation21; antischistosomal activity against Schistosoma mansoniCitation22 and antidibetic property in rats.Citation23 Moreover, phytol's physico-chemical properties are comparable with diesel biofuel.Citation24
The isoprenoid precursor molecule DMAPP can be produced through either the mevalonate (MVA) or methyl-erythritol-4-phosphate (MEP) biosynthetic pathway. The MVA pathway occurs in archea and eukaryotic cytosol while the MEP pathway occurs in prokaryotes such as cyanobacteria and plant chloroplasts.Citation25-27 In the MEP pathway DOXP synthase (DXS) catalyzes the condensation of pyruvate and glyceraldehyde 3-phosphate into deoxy-xylulose-5-phosphate (DOXP). In subsequent reactions DOXP reductase (DXR), 4-diphosphocytidyl-2-C-methyl-D-erythritol synthase (CMS), 4-diphosphocytidyl-2-C-methyl-D-erythritol kinase (CMK), 2-C-methyl-D-erythritol 2,4-cyclodiphosphate synthase (MDS), (E)-4-Hydroxy-3-methyl-but-2-enyl pyrophosphate (HMB-PP) synthase (HDS) and HMB-PP reductase (HDR) respectively converts DOXP into IPP. IPP isomerase can then freely inter-convert IPP to DMAPP.Citation28,29
Photosynthetic hosts have an advantage of producing MBO directly from sunlight utilizing existing environmental CO2 making this process renewable, environmental friendly, and without increasing greenhouse gases that would otherwise contribute to increase in global warming. In our previous work, MBO was produced in E. coli using codon optimized Pinus sabiniana MBO synthase gene in conjunction with an introduced MVA pathway.Citation30 Here, our aim was to produce photosynthetically derived MBO by expressing MBO synthase gene in a cyanobacterial host using the existing MEP pathway for synthesis of precursor metabolites. This was deemed feasible based upon observations that the unicellular cyanobacterium Synechocystis sp PCC 6803 could produce isoprene upon addition of an isoprene synthase gene,Citation28 indicating that cyanobacterial MEP pathway could produce enough of the common DMAPP precursor metabolite for isoprene, or in our experiments for MBO production.
N. punctiforme is an excellent model system for this work due to its sequenced genome,Citation31 well developed genetic system,Citation32-34 and full genome DNA microarray.Citation35 Recently N. punctiforme has been shown to produce neutral lipid droplets enriched with C17 alkanes, making this strain of interest for biotechnical applications.Citation36
In this study, an artificial plasmid containing an indigenous promoter for gene expression in N. punctiforme was built and expression of MBO synthase gene was clearly attained. Although MBO synthase was transcribed and translated, MBO was not produced and instead, production of phytols was enhanced. This result can be explained by one of 2 possible hypotheses involving either (1) a native prenyltransferase enzyme with a broad substrate specificity, or (2) appropriation of a MBO synthase metabolic intermediate by a native GDP synthase that is ultimately channeled to phytol biosynthesis. In addition to revealing information about enzymology and metabolic channeling in cyanobacteria, this work highlights the challenges in production of useful compounds through bioengineering of non-native host cells.
Results
Functional analysis of vector pSUN4KK2
The pSUN4KK2 vector for gene expression in N. punctiforme was constructed and N. punctiforme strains containing the pSUN4KK2 and pSUN119 were developed using electroporation and subsequent antibiotic selection. The pSUN119 is a promoterless vector and was used to develop control strain. The functionality of petE promoter of pSUN4KK2 was analyzed by studying GFP fluorescence from the strains with and without copper. Also, the strains containing pSUN119 was studied for GFP fluorescence to compare and confirm the functionality of the promoter. High levels of GFP florescence were observed in strains containing pSUN4KK2 in the presence of copper present in normal growth media () that was not present in the absence of copper (). Fluorescence in the absence of copper was due to autofluorescence, similar to levels observed in the promoterless control plasmid pSUN119 (). Due to trace contamination of water used in these experiments, micromolar amounts of bathocuproine disulphonate (BCS) were necessary to reduce basal levels of expression in pSUN4KK2. These levels of BCS were not found to limit growth.
Analysis of MBO synthase transgene expression
The Pinus sabiniana MBO synthase gene was cloned in the pSUN4KK2 vector to form pSUN4KK2-MBO in the correct orientation for transcription from the cyanobacterial petE promoter. N. punctiforme strain SBG101 containing the empty vector and strain SBG102 containing pSUN4KK2-MBO were constructed using electroporation and subsequent antibiotic selection. To test if transcription of MBO synthase occurred using an independent method, reverse-transcriptase (RT)-PCR was performed. RT-PCR results showed the presence of MBO synthase cDNA in both biological replications of transgenic strains SBG102 (). The size of the PCR product for replicate SBG102 samples exactly matched the size of the positive control but neither of the replicates for the empty vector control strains SBG101 contained MBO synthase cDNA. The negative controls N, using water as the template, and NN, using same amount of template from a reaction lacking reverse transcriptase confirms that the bands produced in the RT-PCR for SBG102 originally came from cDNA derived from mRNA and not from DNA contamination in the RNA samples. These results indicate that transgene MBO synthase is present and expressed in the transgenic strain of N. punctiforme SBG102 at the mRNA level.
Figure 2. Result of reverse transcriptase (RT) PCR showing expression of MBO synthase gene in transgenic N. punctiforme strain SBG102. Lanes K1 and K2 are biological replication of control strain SBG101 bearing pSUN4KK2 and KM1 and KM2 are biological replication of transgenic strain SBG102 bearing pSUN4KK2-MBO. Lane L is size ladder, N is template negative control for PCR, NN is negative control using product from reaction lacking reverse transcriptase, P is positive control using the template pSUN4KK2-MBO.
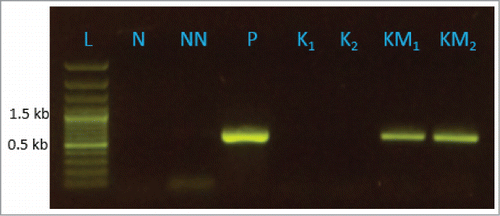
To study MBO synthase transgene expression at the protein level, SDS-PAGE was performed. Total soluble protein was used for SDS-PAGE. Analysis of stained gels indicated the presence of a ∼70 kD protein in the transgenic strain SBG102 sample that was not present in the control strain SBG101 sample (). This observation supports the contention that MBO synthase enzyme is produced in the host N. punctiforme strain SBG102.
Figure 3. SDS-PAGE protein analysis of crude protein extracts from control and transgenic strains of N. punctiforme. Lanes, 1 is total soluble protein from control strain SBG101; 2 is total soluble protein from transgenic strain SBG102; L is standard protein ladder in kilodaltons (kD). A faint band similar in size to MBO synthase (comparable to 70 kD and present in lane 2 but absent in lane 1) indicates expression of MBO synthase at protein level.
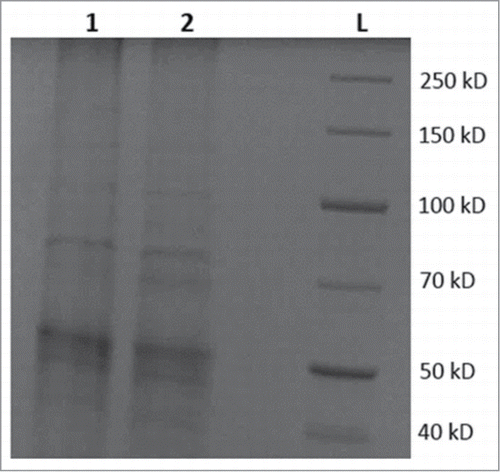
MBO production in control vs. transgenic N. punctiforme
Headspace air samples were used to analyze the production of volatile MBO from control SBG101 and transgenic SBG102 cultures through a GC-FID and GC-MS. None of the samples from control as well as transgenic strains showed any detectable peak comparable to MBO standard (data not shown). We further repeated this experiment with addition of NaCO3 in media to supplement the cyanobacteria with CO2 as well as with larger volumes of culture to ensure an adequate amount of host cells were present. In all cases, the headspace air did not contain MBO after incubation in quantities that were detectable by gas chromatography.
GC-MS analysis of total lipid extracted from both controls vs. transgenic N. punctiforme
Assuming the enzyme was active, it was hypothesized that MBO might be polymerized into higher molecular weight non-volatile isoprenoids that were incorporated into cell material. Due to their chemical nature, isoprenoids have hydrophobic characteristics, therefore analysis of total lipids were chosen for analysis. GC-MS analysis of lipid samples from strains SBG101 and SBG102 indicated differential expression of 3 peaks at retention times (RT) of 10.500, 10.667 and 10.792 min. These were found to be more highly expressed in lipid extracts from SBG102 than in SBG101 (). These peaks were respectively found to be phytol acetate and 2 phytol sterioisomers (here we termed them all together as phytols) with 91, 93 and 93% similarity match to NIST/EPA/NIH Mass Spectral Library (Data version: NIST 11, Software version 2.0). There are 29 different sterioisomers of phytol available through the NCBI PubChem. Phytols produced in our samples matched to phytol [InChI = 1S/C20H40O/c1-17(2)9-6-10-18(3)11-7-12-19(4)13-8-14-20(5)15-16-21/h15,17-19,21H,6-14,16H2,1-5H3]. Our purchased standard matched to phytol [InChI = 1S/C20H40O/c1-17(2)9-6-10-18(3)11-7-12-19(4)13-8-14-20(5)15-16-21/h15,17-19,21H,6-14,16H2,1-5H3/b20-15+/t18-,19-/m0/s1]. We were unable to find the exact commercially available standards for phytols identified from N. punctiforme in this study, however, fragmentation pattern of mass spectra produced for these peaks match to authentic NIST/EPA/NIH Mass Spectral Library with more than 90% similarity (Supplementary data file). These results indicate MBO was incorporated into a 20-carbon branched chain alcohol molecule identical to an isomer of phytol that was also present in an acetylated form.
Figure 4. Chromatograph showing differential production of phytols in transgenic strains SBG102 (pink) verses control strains SBG101 (black) where peak 1, 2 and 3 represent phytol acetate and 2 phytols (likely sterioisomers), respectively. Numbers on the bottom represent retention time (RT) in minutes.
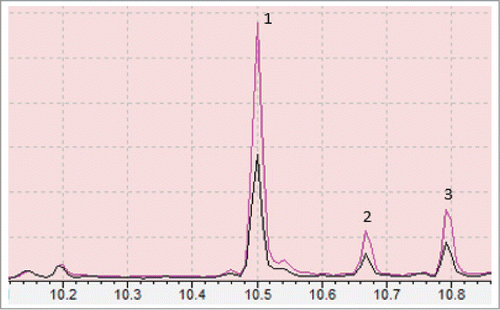
Localization and quantification of phytol production in N. punctiforme
To find out the location of phytol accumulation, cells were fractionated into lipid droplets, cytoplasm and membranes using ultra-centrifugation. Analysis of total lipids extracted from each fraction by GC-MS indicated that the peaks for phytols with retention times of 10.500, 10.667 and 10.792 min appeared only from the fraction containing soluble cytoplasmic components but not in the fraction containing lipid droplets or membranes/cell debris for all 3 biological replicates of transgenic and control strains. Phytols were quantified in transgenic compare to the control by comparing the peak area with those of a phytol isomer standard. The phytol standard produced 2 peaks at a RT of 12.125 and 12.275 min. An internal eicosane control (peak at RT of 4.325) was used to determine an extraction efficiency of 63.7% for the Bligh Dyer solvent system. Using this correction factor, the amount of phytols produced by these cells indigenously was calculated to be 2.473 μg, 0.384 μg, and 0.646 μg/mg Chla for the SBG102 transgenic strain and 1.122 μg, 0.168 μg and 0.303 μg/mg Chla for the SBG101 control strain for phytol acetate (RT = 10.500), and the 2 sterioisomers of phytol (RT = 10.677 and 10.792). Thus, the production of these phytols in the transgenic strain was more than twice than that of the control strain ().
Discussion
In this work, a new artificial expression vector pSUN4KK2 was constructed and the functionality of the petE promoter contained in the vector was confirmed. The pSUN4KK2 vector was used to express Pinus sabiniana MBO synthase gene in the cyanobacterial host N. punctiforme. We successfully showed the expression of MBO synthase under the control of the petE promoter. The petE promoter is indigenous to N. punctiforme and transcribes the soluble copper-containing photosynthetic electron carrier plastocyanin. In the related cyanobacterium Nostoc sp. strain PCC 7120, the petE promoter requires copper for expression,Citation37 and a similar response was found for the N. punctiforme promoter used in pSUN4KK2 based on expression of the promoterless green fluorescent protein reporter located in pSUN4KK2 (). In copper-containing media such as used in our experiments (), the petE promoter exhibits strong basal expression in ammonia-grown cultures of ∼19% of the averaged expression of the 20 most highly transcribed genes based upon microarray analysis.Citation35 Perhaps due to this strong transcription, a protein is clearly observed at 70 kD in the transgenic strain which exactly matches the expected size of MBO synthaseCitation2, indicating that expression from this promoter on a multi-copy plasmid is capable of producing large quantities of protein. Only crude soluble proteins were used in SDS-PAGE since MBO synthase is a soluble protein.Citation2
Although we confirmed MBO synthase gene transcription () and translation () by performing a RT-PCR and SDS-PAGE, respectively, MBO production could not be confirmed at a detectable level. However, we found production of phytols in higher amount in the transgenic strain expressing the MBO synthase gene (). Over production of phytols with MBO transgene expression in N. punctiforme can be explained by 2 plausible pathways () that are supported by previously reported information.
Isopreonoids consist of a large family of organic compounds and broadly classified based upon its carbon numbers into hemiterpenes (C5), monoterpenes (C10), sesquiterpenes (C15), diterpenes (C20), triterpenes (C30), tetraterpenes (C40), longer-chain poly-isoprenoids and their derivatives that are produced from IPP and DMAPP precursors. DMAPP is the substrate for hemiterpene synthases like isoprene synthase and MBO synthase. Prenyltransferases also use DMAPP as an initial allylic unit and catalyze the sequential condensation reaction with IPP for production of elongated prenyl pyrophosphate precursors geranyl diphosphate (GDP; a 10-carbon C10 compound), farnesyl diphosphate (FDP; C15) and geranylgeranyl diphosphate (GGDP; C20).Citation38,39 These prenyl diphosphates are used in synthesis of different isoprenoids through respective bioenzymatic reactions in the isoprenoid pathway. Class I bacterial prenyltransferase contains a short-chain (C15-C20) prenyltransferases.Citation40,41 Ohto et al. 1999, reported 3 different Class I prenyltransferase from a cyanobacterium Synechococcus elongatus and those prenyltransferases were able to accept DMAPP as the initial allylic substrate.Citation42 A KEGG database search for the terpene backbone biosynthetic pathway in N. punctiforme has shown the presence of enzymes for the MEP pathway as well as for steps 1, 2, 4 and 6 in model-I () (http://www.genome.jp/kegg-bin/show_pathway?npu00900). A pblast with protein sequence of enzymes for steps 5 and 7 in model-I () into N. punctiforme have shown the presence of different potential genes.
Figure 6. A plausible biosynthetic pathway leading to formation of phytol in N. punctiforme. In the above diagram enzymes I, 1, 2, 4, 5, 6 and 7 shown in green are potential native enzymes: IPP isomerase (enzyme I) geranyl diphosphate (GDP) synthase (enzyme 1); farnesyl diphosphate (FDP) synthase (enzyme 2); geranylgeranyl diphosphate (GGDP) synthase (enzyme 4); pyrophosphatases (enzyme 5); geranylgeranyl reductase (GGR) (enzyme 6) and geranylgeraniol (GGOH) reductase (enzyme 7). Enzyme 3 is the foreign MBO synthase. GDP synthase, FDP synthase and GGDP synthase shown in gray as 1*, 2* and 4* respectively, are bacterial class I prenyltransferases with proposed broad range substrates specificity. Solid black arrow represents already known or proposed pathways while gray arrows show plausible reactions proposed in this model. Structures are adopted from NCBI pubchem compound library (http://pubchem.ncbi.nlm.nih.gov).
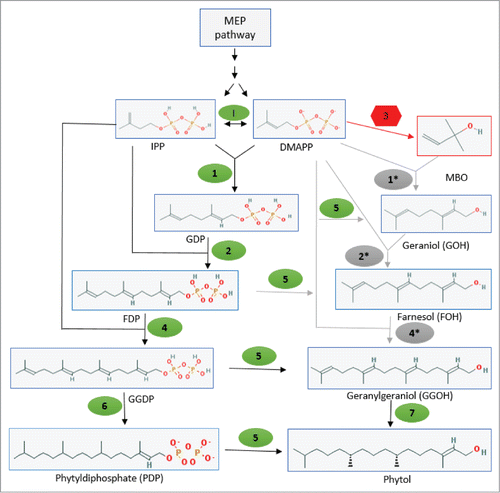
In model-I, we proposed that intracellularly produced MBO in N. punctiforme is utilized immediately by a prenyltransferase (C10-C20) with a broad range of substrate specificity to over produce geraniol (GOH), farnesol (FOH) and then to geranylgeraniol (GGOH) required substrates for subsequent phytol over-production (). Schuller et al. (2012) shown that a cyclic dipeptide N-prenyltransferase (CdpNPT) can use a diverse range of substrates, and Koeduka et al. (2011) has expressed a Streptomyces prenyltransferase HypSc (SCO7190) possessing broad-range substrate specificity in tomato and shown production of prenylated flavonoids, 3′-dimethylallyl naringenin (C20H20O5).Citation43,44 These studies indicate that prenyltransferases can utilize a broad range of substrates including those lacking a diphosphate group, supporting our hypothesis that a broad range prenyltransferase exists in N. punctiforme.
Although broad spectrum prenyltransferases have been reported, we do not find one that uses a linear substrate similar to MBO, GOH or FOH. Therefore, over production of phytol in the transgenic strain may occur through an alternative model (). MBO synthase catalyzes the conversion of DMAPP to MBO through a transient carbocation molecule.Citation45 Similarly, as GDP synthase catalyzes condensation of DMAPP and IPP, it first dephosphorylates DMAPP into the same transient carbocation.Citation46 It is plausible that recombinant MBO synthase produced here in N. punctiforme may have lost activity resulting in the final hydrolysis but retain activity resulting in intermediate formation, perhaps due to miss folding or any number of other factors. As such, it might be able to accept its DMAPP substrate, dephosphorylate it into carbocation intermediate but lose it before its hydrolysis into MBO. Leaked carbocation intermediate in this way might be used instantly by GDP synthase to produce GDP which can be utilized in the following steps to produce phytols as shown in model-I. Either model could explain why production of volatile MBO was not observed.
Figure 7. Alternative model leading to overproduction of phytol through supply of a metabolic intermediate created by MBO synthase to GDP synthase in N. punctiforme. MBO synthase (enzyme 3); GDP synthase (enzyme 1); transient carbocation common to both reactions is shown in brackets. Top and bottom panel is adapted fromCitation45 and,Citation46 respectively. OPP denotes pyrophosphate (PPi) attachment.
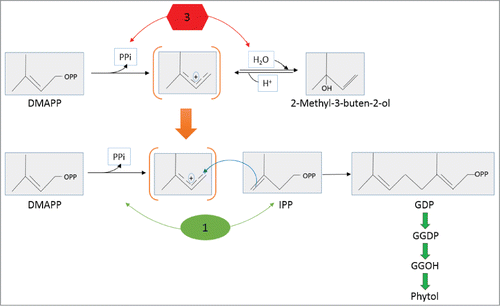
Cyanobacteria have been shown to utilize the MEP pathway for production of phytol.Citation27 The first reaction of the MEP pathway catalyzed by DOXP synthase is controlled through feedback inhibition by both IPP and DMAPP.Citation47,48 If a similar control mechanism exists in cyanobacteria, this would explain the limited production of phytols in control strain SBG101. In the transgenic strain however, recombinant MBO synthase could continuously convert DMAPP substrate to either MBO or a carbocation intermediate and in this way lower the concentration of DMAPP below threshold requirement for feedback inhibition. As a consequence, DMAPP would be expected to be produced at a higher rate in the transgenic strain which in turn would lead to more MBO for incorporation into phytols using either of the proposed models.
Phytol production from GGDP or GGOH in these models, is in accordance with a previous study which showed that reduction of carbon labeled GGDP into carbon labeled phytol when incubated with purified spinach chloroplasts.Citation49 From the above study we can confer the presence of both reductase and phosphatase activity in the chloroplast. Since, cyanobacteria are evolutionarily related to chloroplasts, we assume similar properties exist in our host and over production of GDP or GOH, FDP or FOH and GGDP or GGOH could lead to enhanced production of phytols as observed in our results.
We conclude that synthesis of phytol compounds can be enhanced in cyanobacteria by intracellular supply of the foreign metabolite MBO that is integrated into existing metabolism. Further work will be required to find the mechanism of incorporation and understand the metabolic complexities limiting expression of compounds of potential interest to medicine and energy.
Materials and Methods
Strains and growth conditions
N. punctiforme ATCC 29133 was used as the expression host. Cultures were grown in 50 ml of liquid Alan and Arnon medium (AA/4) containing 5 mM MOPS buffer (pH 7.8), 2.5 mM NH4Cl, 2.5 mM NaNO3 and 2.5 mM KNO3 in 125 ml EM flasks or on solid media as previously describedCitation50 using 10 μg ml−1 neomycin for plasmid selection. E. coli DH5α was grown in Luria-Bertani (LB) broth and agar plates using 30 μg ml−1 kanamycin for plasmid selection cloning and vector construction. Culture densities were based on chlorophyll concentrations after extraction in 90% methanol as described previously.Citation51 Normal AA/4 media (as described above) was used for culture grown in presence of copper. Filter sterilized copper free media was prepared using a micronutrient stock lacking copper with nanopure water. To chelate any residual copper, filter sterilized bathocuproine disulphonate (BCS) was added to a final concentration of 35 or 50 μM.
Vector construction and transformation
To create pSUN4KK2, 184 bp of the upstream intergenic region of Npun_R2789 containing the petE promoter was PCR amplified from N. punctiforme genomic DNA using upstream primer ggcaCTGCAGatagggcattgggcattgggaagt and downstream primer atggCTGCAGcctaacaaacaattttttttcctt. The transcriptional start site was located 24 bp from the downstream PstI site based on RACE analysis as previously described.Citation52 The PstI cut PCR fragment was cloned into the PstI site of pSUN119Citation53 in the correct orientation to initiate transcription toward the downstream multiple cloning site (MCS). The MCS was extended to include 5 additional restriction sites by cloning 25 additional bp into the KpnI site. Double stranded DNA for this extension was created by annealing 2 complementary oligonucleotides containing flanking KpnI sites to add the intervening sequence PpetE – GGGCCCTCTAGACCGGTGCGGCCGC – MCS. Sequencing confirmed the petE promoter was correctly situated upstream from the MCS containing restriction sites for ClaI, SmaI, XmaI, ApaI, XbaI, AgeI, NotI, and SacII.
P. sabiniana MBO synthase gene optimized for E. coli expression contained in a plasmidCitation2,30 was excised using XbaI and NotI and cloned into a similarly digested pSUN4KK2 vector to form pSUN4KK2-MBO. The pSUN4KK2-MBO and pSUN4KK2 plasmids were electroporated into N. punctiforme as described beforeCitation50 to form strains SBG102 and SBG101, respectively.
Reverse transcription PCR (RT-PCR)
Total RNA was extracted from duplicate 50 ml cultures of N. punctiforme SBG101 and SBG102 strains grown to a chlorophyll concentration of 6.5 μg Chla ml−1. Quality and concentration of RNA was assayed using a NanoDrop 2000c Spectrophotometer (Thermo Scientific, USA). One microgram of RNA from all samples were used for cDNA synthesis using iScript Reverse Transcriptase for RT-qPCR (BIO-RAD, USA). Reverse transcription was conducted in PCR tubes at 25°C for 5 min, 42°C for 30 min, and 85°C for 5 min. A negative control (NC) contained the same amount of 1 μg total RNA from strain SBG102 and iScript master mix (BIO-RAD, USA) but lacking reverse transcriptase. PCR was performed using equal volumes of control and experimental cDNAs as template, 1 μl of 10 μM of primers MBOS-f (5′-GCTTGTAGCGCGATTCAAACGGAA) and MBOS-r (5′-AGACGCCTTTCATGTACTCTGGCA) for MBO synthase detection and master mix (Fermentas, USA). A positive control using pSUN4KK2-MBO plasmid and 2 negative controls, one with dH2O as the template (N) and another (NN) using product of NC from above reverse transcriptase steps were included. To assure RNA was not contaminated with genomic DNA, RNA samples were used as templates in PCR reactions with the primers above. PCR was performed at an initial denaturation at 95°C for 5 min, 30 cycles with denaturation at 95°C for 30 s, annealing at 55.2°C for 30 s and extension at 72°C for 1 min, with a final extension at 72°C for 5 min.
SDS-PAGE analysis
To study the expression of MBO synthase gene at protein level, cells from 1 ml culture of strains SBG101 and SBG102 grown to approximately 6.5 μg Chla ml−1 chlorophyll were harvested by centrifugation and the pellet suspended in total volume of 300 μl with TBS (Tris-Base saline). Cells were lysed by probe sonication (Ultrasonic Processor) using a microtip at 14% amplitude for 5 cycles of 30 s on ice. After centrifugation at 13,000 × g for 10 min at 4°C, supernatants from both samples were removed and 15 μl of each sample used for SDS-PAGE analysis.
Analysis of MBO production
To assay MBO production from N. punctiforme, both control strain SBG101 and transgenic strain SBG102 were grown in closed vials and head space air was tested through gas chromatograph (GC) and gas chromatograph – mass spectrophotometer (GC-MS). Triplicates of 3 ml and 6 ml of liquid Alan and Arnon medium in sealable tubes were inoculated with log phase SBG101 and SBG102 cultures respectively. The cultures were then air-tight with rubber stoppers, crimp sealed and incubated for 7–10 days at 25°C with shaking under continuous photoperiod. Above experiment was also repeated with addition of 25 mM NaCO3 in the media to overcome CO2 limitation in a closed system. Following incubation, headspace air were sampled and analyzed both through a GC–FID using a Hewlett-Packard,HP6890 Gas Chromatograph as previously describedCitation30 and a QP2010S GC-MS (SHIMADZU, USA) equipped with a HP-5 column (30 m, 0.32 mm, 0.25 μM) held at 65°C for 1 minutes, increased to 300°C at 15°C min−1, with a 300°C final temperature maintained for 2 minutes, with a split ratio of 1:75 and helium carrier gas at a flow rate of 1.2 ml min−1. MBO standard was obtained from Sigma-Aldrich.
GC-MS analysis of total lipid extracted from control vs transgenic N. punctiforme
Cultures grown to late log/ early stationary phase were used for lipid extraction. Total lipids were extracted from samples containing 175 μg Chla concentrated to a final volume of 1 ml using Bligh and Dyer method.Citation54 Care was taken in handling of samples to minimize any biasness in the result. Equal volume of extracted lipids were dried under a stream of N2 gas and then dissolved in equal volume of hexane (Sigma-Aldrich, USA) before injected into a QP2010S GC-MS (SHIMADZU, USA) using the settings described above.
Localization and quantification of phytol production in N. punctiforme
Pellets containing 360 μg Chla harvested from early stationary phase by centrifugation, were suspended in 25 mM Tris-HCl (pH 7.5), 250 mM sucrose and lysed using 2 passes through a French pressure cell at 16000 psi. The lysate was placed in an ultracentrifuge tube and 3 ml of deionized water was gently added atop the lysate. Ultracentrifugation was performed for 150 min at 26000 rpm at 23°C. Following centrifugation, a top layer containing lipid droplets, middle layer containing cytoplasmic contents of the cells, and pellet containing cell membranes and cell debris were collected in separate tubes. Total lipids were extracted and analyzed through GC-MS as above. An internal standard (eicosane; C20 straight chain hydrocarbon which was not found to produce indigenously in N. punctiforme) was added to samples prior to extraction to estimate the lipid extraction efficiency. Phytol production was quantified by comparing peak areas with that of a phytol standard (Sigma-Aldrich, USA).
Disclosure of Potential Conflicts of Interest
No potential conflicts of interest were disclosed.
Acknowledgments
We sincerely acknowledge Dr. Thomas D. Sharkey, Michigan State University for providing us MBO synthase gene contained in a plasmid and Dr. Dennis Gray, Saginaw Valley State University for helpful information about the plasmid.
Funding
We acknowledge a partial student stipend provided to DG through Pamela M. Klein, M.D. Scholarship in Biology at CSUN. This work was also supported by National Science Foundation Grant MCB-1413583 to MLS.
References
- Guenther A, Karl T, Harley P, Wiedinmyer C, Palmer PI, Geron C. Estimates of global terrestrial isoprene emissions using MEGAN (Model of Emissions of Gases and Aerosols from Nature). Atmos Chem Phys 2006; 6:3181-210.
- Gray DW, Breneman SR, Topper LA, Sharkey TD. Biochemical characterization and homology modeling of methylbutenol synthase and implications for understanding hemiterpene synthase evolution in plants. J Biol Chem 2011; 286:20582-90; PMID:21504898; http://dx.doi.org/10.1074/jbc.M111.237438
- Vickers CE, Possell M, Cojocariu CI, Velikova VB, Laothawornkitkul J, Ryan A, Mullineaux PM, Nicholas Hewitt C. Isoprene synthesis protects transgenic tobacco plants from oxidative stress. Plant Cell Environ 2009; 32:520-31; PMID:19183288
- Loreto F, Schnitzler JP. Abiotic stresses and induced BVOCs. Trends Plant Sci 2010; 15:154-66; PMID:20133178; http://dx.doi.org/10.1016/j.tplants.2009.12.006.
- Sharkey TD, Singsaas EL. Why plants emit isoprene. 1995; 374:769
- Fineschi S, Loreto F. Leaf volatile isoprenoids: an important defensive armament in forest tree species. iForest–Biogeosci Forestry 2012; 5:13-7; http://dx.doi.org/10.3832/ifor0607-009
- Siwko ME, Marrink SJ, de Vries AH, Kozubek A, Schoot Uiterkamp AJ, Mark AE. Does isoprene protect plant membranes from thermal shock? A molecular dynamics study. Biochim Biophys Acta 2007; 1768:198-206; PMID:17125733; http://dx.doi.org/10.1016/j.bbamem.2006.09.023
- Singsaas EL, Lerdau M, Winter K, Sharkey TD. Isoprene increases thermotolerance of isoprene-emitting species. Plant Physiol 1997; 115:1413-20; PMID:12223874
- Singsaas EL, Sharkey TD. The effects of high temperature on isoprene synthesis in oak leaves. 2000; 23:751-7
- Way DA, Schnitzler JP, Monson RK, Jackson RB. Enhanced isoprene-related tolerance of heat- and light-stressed photosynthesis at low, but not high, CO2 concentrations. Oecologia 2011; 166:273-82; PMID:21380850; http://dx.doi.org/10.1007/s00442-011-1947-7
- Gray DW, Lerdau MT, Goldstein AH. Influences of temperature history, water stress, and zneedle age on methylbutenol emissions. Ecology 2003; 84:765-76; http://dx.doi.org/10.1890/0012-9658(2003)084%5b0765:IOTHWS%5d2.0.CO;2
- Sharkey TD. The future of isoprene research. BullGeorg Natl Acad Sci 2009; 5:106-13
- Zwenger S, Basu C. Plant terpenoids: applications and future potentials. Biotechnol Mol Biol Rev 2008; 3:1-7.
- Kirby J, Keasling JD. Biosynthesis of plant isoprenoids: perspectives for microbial engineering. Annu Rev Plant Biol 2009; 60:335-55; PMID:19575586; http://dx.doi.org/10.1146/annurev.arplant.043008.091955
- Martin VJ, Pitera DJ, Withers ST, Newman JD, Keasling JD. Engineering a mevalonate pathway in Escherichia coli for production of terpenoids. Nat Biotechnol 2003; 21:796-802; PMID:12778056; http://dx.doi.org/10.1038/nbt833
- Ajikumar PK, Xiao WH, Tyo KE, Wang Y, Simeon F, Leonard E, Mucha O, Phon TH, Pfeifer B, Stephanopoulos G. Isoprenoid pathway optimization for Taxol precursor overproduction in Escherichia coli. Science 2010; 330:70-4; PMID:20929806; http://dx.doi.org/10.1126/science.1191652
- Santos CCdMP, Salvadori MS, Mota VG, Costa LM, de Almeida AAC, de Oliveira GAL, et al. Antinociceptive and antioxidant activities of phytol in vivo and in vitro models. Neurosci J 2013; 2013:9; http://dx.doi.org/10.1155/2013/949452
- Ryu KR, Choi JY, Chung S, Kim DH. Anti-scratching behavioral effect of the essential oil and phytol isolated from Artemisia princeps Pamp. in mice. Planta Med 2011; 77:22-6; PMID:20645242; http://dx.doi.org/10.1055/s-0030-1250119
- Rajab MS, Cantrell CL, Franzblau SG, Fischer NH. Antimycobacterial activity of (E)-phytol and derivatives: a preliminary structure-activity study. Planta Med 1998; 64:2-4; PMID:9491760; http://dx.doi.org/10.1055/s-2006-957354
- Saikia D, Parihar S, Chanda D, Ojha S, Kumar JK, Chanotiya CS, Shanker K, Negi AS. Antitubercular potential of some semisynthetic analogues of phytol. Bioorg Med Chem Lett 2010; 20:508-12; PMID:20004575; http://dx.doi.org/10.1016/j.bmcl.2009.11.107
- Inoue Y, Hada T, Shiraishi A, Hirose K, Hamashima H, Kobayashi S. Biphasic effects of geranylgeraniol, teprenone, and phytol on the growth of Staphylococcus aureus. Antimicrob Agents Chemother 2005; 49:1770-4; PMID:15855494; http://dx.doi.org/10.1128/AAC.49.5.1770-1774.2005
- de Moraes J, de Oliveira RN, Costa JP, Junior AL, de Sousa DP, Freitas RM, Allegretti SM, Pinto PL. Phytol, a diterpene alcohol from chlorophyll, as a drug against neglected tropical disease Schistosomiasis mansoni. PLoS Negl Trop Dis 2014; 8:e2617; http://dx.doi.org/10.1371/journal.pntd.0002617
- Elmazar MM, El-Abhar HS, Schaalan MF, Farag NA. Phytol/Phytanic acid and insulin resistance: potential role of phytanic acid proven by docking simulation and modulation of biochemical alterations. PLoS One 2013; 8:e45638; PMID:23300941; http://dx.doi.org/10.1371/journal.pone.0045638
- Muradov N, Taha M, Miranda AF, Kadali K, Gujar A, Rochfort S, Stevenson T, Ball AS, Mouradov A. Dual application of duckweed and azolla plants for wastewater treatment and renewable fuels and petrochemicals production. Biotechnol Biofuels 2014; 7:30; PMID:24576349; http://dx.doi.org/10.1186/1754-6834-7-30
- Lichtenthaler HK. Non-mevalonate isoprenoid biosynthesis: enzymes, genes and inhibitors. Biochem Soc Trans 2000; 28:785-9; PMID:11171208; http://dx.doi.org/10.1042/BST0280785
- Rohmer M. The discovery of a mevalonate-independent pathway for isoprenoid biosynthesis in bacteria, algae and higher plants[dagger]. Nat Prod Rep 1999; 16:565-74; PMID:10584331; http://dx.doi.org/10.1039/a709175c
- Disch A, Schwender J, Müller C, Lichtenthaler HK, Rohmer M. Distribution of the mevalonate and glyceraldehyde phosphate/pyruvate pathways for isoprenoid biosynthesis in unicellular algae and the cyanobacterium Synechocystis PCC 6714. Biochem J 1998; 333 ( Pt 2):381-8; PMID:9657979
- Lindberg P, Park S, Melis A. Engineering a platform for photosynthetic isoprene production in cyanobacteria, using Synechocystis as the model organism. Metab Eng 2010; 12:70-9; PMID:19833224; http://dx.doi.org/10.1016/j.ymben.2009.10.001
- Barkley SJ, Desai SB, Poulter CD. Type II isopentenyl diphosphate isomerase from Synechocystis sp. strain PCC 6803. J Bacteriol 2004; 186:8156-8; PMID:15547291; http://dx.doi.org/10.1128/JB.186.23.8156-8158.2004
- Gupta D, Summers M, Basu C. Engineering an isoprenoid pathway in escherichia coli for production of 2-Methyl-3-buten-2-ol: a potential biofuel. Mol Biotechnol 2014; 56:516-23; PMID:24271564; http://dx.doi.org/10.1007/s12033-013-9721-1
- Meeks JC, Elhai J, Thiel T, Potts M, Larimer F, Lamerdin J, Predki P, Atlas R. An overview of the genome of Nostoc punctiforme, a multicellular, symbiotic cyanobacterium. Photosynth Res 2001; 70:85-106; PMID:16228364; http://dx.doi.org/10.1023/A:1013840025518
- Elhai J, Wolk CP. Conjugal transfer of DNA to cyanobacteria. Methods Enzymol 1988; 167:747-54; PMID:3148842; http://dx.doi.org/10.1016/0076-6879(88)67086-8
- Cohen MF, Wallis JG, Campbell EL, Meeks JC. Transposon mutagenesis of Nostoc sp. strain ATCC 29133, a filamentous cyanobacterium with multiple cellular differentiation alternatives. Microbiology 1994; 140 (Pt 12):3233-40; PMID:7881544; http://dx.doi.org/10.1099/13500872-140-12-3233
- Argueta C, Yuksek, K., Summers, M. Construction and use of GFP reporter vectors for analysis of cell-type-specific gene expression in Nostoc punctiforme. J Microbiol Methods 2004; 59(2):181-8; PMID:15369854; http://dx.doi.org/10.1016/j.mimet.2004.06.009
- Campbell EL, Summers ML, Christman H, Martin ME, Meeks JC. Global gene expression patterns of Nostoc punctiforme in steady-state dinitrogen-grown heterocyst-containing cultures and at single time points during the differentiation of akinetes and hormogonia. J Bacteriol 2007; 189:5247-56; PMID:17483217
- Peramuna A, Summers ML. Composition and occurrence of lipid droplets in the cyanobacterium Nostoc punctiforme. Arch Microbiol 2014; PMID:25135835
- Buikema WJ, Haselkorn R. Expression of the Anabaena hetR gene from a copper-regulated promoter leads to heterocyst differentiation under repressing conditions. Proceed Natl Acad Sci U S A 2001; 98:2729-34; PMID:11226308
- Kellogg BA, Poulter CD. Chain elongation in the isoprenoid biosynthetic pathway. Curr Opin Chem Biol 1997; 1:570-8; PMID:9667899
- Vickers CE, Bongers M, Liu Q, Delatte T, Bouwmeester H. Metabolic engineering of volatile isoprenoids in plants and microbes. Plant Cell Environ 2014; 37:1753-75; PMID:24588680
- Koike-Takeshita A, Koyama T, Obata S, Ogura K. Molecular cloning and nucleotide sequences of the genes for two essential proteins constituting a novel enzyme system for heptaprenyl diphosphate synthesis. J Biol Chem 1995; 270:18396-400; PMID:7629164
- Ogura K, Koyama T. Enzymatic aspects of isoprenoid chain elongation. Chem Rev 1998; 98:1263-76; PMID:11848932
- Ohto C, Ishida C, Nakane H, Muramatsu M, Nishino T, Obata S. A thermophilic cyanobacterium Synechococcus elongatus has three different class I prenyltransferase genes. Plant Mol Biol 1999; 40:307-21; PMID:10412909
- Schuller JM, Zocher G, Liebhold M, Xie X, Stahl M, Li SM, Stehle T. Structure and catalytic mechanism of a cyclic dipeptide prenyltransferase with broad substrate promiscuity. J Mol Biol 2012; 422:87-99; PMID:22683356
- Koeduka T, Shitan N, Kumano T, Sasaki K, Sugiyama A, Linley P, Kawasaki T, Ezura H, Kuzuyama T, Yazaki K. Production of prenylated flavonoids in tomato fruits expressing a prenyltransferase gene from Streptomyces coelicolor A3(2). Plant Biol (Stuttg) 2011; 13:411-5; PMID:21309988
- Silver GM, Fall R. Characterization of aspen isoprene synthase, an enzyme responsible for leaf isoprene emission to the atmosphere. J Biol Chem 1995; 270:13010-6; PMID:7768893
- Burke CC, Wildung MR, Croteau R. Geranyl diphosphate synthase: cloning, expression, and characterization of this prenyltransferase as a heterodimer. Proc Natl Acad Sci U S A 1999; 96:13062-7; PMID:10557273
- Rodríguez-Concepción M. Early Steps in Isoprenoid Biosynthesis: Multilevel Regulation of the Supply of Common Precursors in Plant Cells. Phytochemistry Reviews 2006; 5:1-15
- Banerjee A, Wu Y, Banerjee R, Li Y, Yan H, Sharkey TD. Feedback inhibition of deoxy-D-xylulose-5-phosphate synthase regulates the methylerythritol 4-phosphate pathway. J Biol Chem 2013; 288:16926-36; PMID:23612965
- Soll J, Schultz G. Phytol synthesis from geranylgeraniol in spinach chloroplasts. Biochem Biophys Res Commun 1981; 99:907-12; PMID:7247947
- Summers ML, Wallis JG, Campbell EL, Meeks JC. Genetic evidence of a major role for glucose-6-phosphate dehydrogenase in nitrogen fixation and dark growth of the cyanobacterium Nostoc sp. strain ATCC 29133. J Bacteriol 1995; 177:6184-94; PMID:7592384
- Meeks JC, Castenholz RW. Growth and photosynthesis in an extreme thermophile, Synechococcus lividus (Cyanophyta). Arch Mikrobiol 1971; 78:25-41; PMID:4999393; http://dx.doi.org/10.1007/BF00409086
- Argueta C, Yuksek K, Patel R, Summers ML. identification of nostoc punctiforme akinete-expressed genes using differential display. Mol Microbiol 2006; 61:748-57; PMID:16780565; http://dx.doi.org/10.1111/j.1365-2958.2006.05263.x
- Argueta C, Yuksek K, Summers M. Construction and use of GFP reporter vectors for analysis of cell-type-specific gene expression in Nostoc punctiforme. J Microbiol Methods 2004; 59:181-8; PMID:15369854; http://dx.doi.org/10.1016/j.mimet.2004.06.009
- Bligh EG, Dyer WJ. A rapid method of total lipid extraction extraction purification. Can J Biochem Physiol 1959; 37:911-7; PMID:13671378; http://dx.doi.org/10.1139/o59-099