Abstract
For as long as the human blood-brain barrier (BBB) has been evolving to exclude bloodborne agents from the central nervous system (CNS), pathogens have adopted a multitude of strategies to bypass it. Some pathogens, notably viruses and certain bacteria, enter the CNS in whole form, achieving direct physical passage through endothelial or neuronal cells to infect the brain. Other pathogens, including bacteria and multicellular eukaryotic organisms, secrete toxins that preferentially interact with specific cell types to exert a broad range of biological effects on peripheral and central neurons. In this review, we will discuss the directed mechanisms that viruses, bacteria, and the toxins secreted by higher order organisms use to enter the CNS. Our goal is to identify ligand-mediated strategies that could be used to improve the brain-specific delivery of engineered nanocarriers, including polymers, lipids, biologically sourced materials, and imaging agents.
Barriers to CNS Delivery
Peripherally administered molecules encounter many barriers before they reach their target. Active agents must avoid degradation and clearance while traveling through blood, cells, and extracellular matrix. These processes will direct the distribution of molecules within tissue compartments, thus determining whether an agent is capable of exerting a biological effect in its target tissue. The blood-brain barrier (BBB) remains one of the prototypical examples of a tissue barrier that restricts the action of peripherally administered substances.Citation1 Non-fenestrated brain endothelial cells possess cell-cell junctions that inhibit passive diffusion of circulating agents into the parenchyma, while efflux transporters, such as P-glycoprotein (Pgp), actively deplete the concentration of molecules that have achieved entry into cells.
Maintenance of BBB integrity is a critical physiological process in human health, since the concentrations of ions, hormones, and metabolic products must be regulated within tight ranges to achieve proper neuronal function. It is well-appreciated that many chemicals and biomolecules that are considered innocuous in peripheral tissue are in fact neurotoxic when present in the CNS. Accordingly, the BBB is evolutionarily conserved, and known to exclude nearly 98% of small molecules and 100% of large molecules.Citation2 The BBB thus poses an often insurmountable blockade to the preclinical evaluation and clinical translation of novel therapies to treat CNS disease.
For as long as the human BBB has evolved to exclude bloodborne agents, pathogens have adopted a multitude of strategies to bypass it.Citation3-5 CNS entry may be achieved through various routes, which are described in . Pathogens or pathogen-derived substances are capable of reaching the CNS by direct passage across the BBB (transcellular), through spaces created between cells (paracellular), by carriage within peripherally circulating leukocytes that engage in immune surveillance of the CNS (Trojan horse), via the axons of neurons that originate the periphery (retrograde), or through regions of the brain with distinct BBB physiology (regions of altered permeability, for example, in the choroid plexus). We propose that the mechanisms utilized by human pathogens for achieving CNS tropism could be adapted to engineer the delivery of drugs to the brain. By linking drugs or drug-loaded carriers to ligands that instruct pathogen entry into the CNS, it may be possible to improve the action of drugs in the brain while reducing systemic dose.
Figure 1. The mechanisms by which human pathogens and the toxins they secrete achieve CNS entry include: (1) receptor- and non-receptor mediated transcytosis, (2), paracellular transport, (3), carriage within immune cells, (4) retrograde transport from the periphery, and (5) entry through regions of altered BBB permeability, such as those found in the choroid plexus of the cerebral ventricles or olfactory neurons.
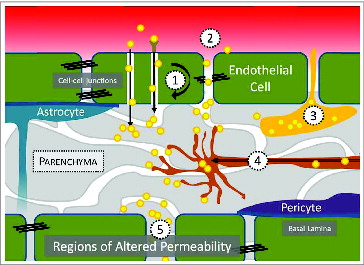
In this review, we will discuss the directed mechanisms that viruses, bacteria, and toxins use to enter the CNS. Our goal is to identify ligand-mediated strategies that could be used to improve the brain-specific delivery of engineered nanocarriers, including polymers, lipids, biologically sourced materials, and imaging agents. Within each main class of virus or organism, example pathogenic strategies that have particular relevance to current drug delivery literature are discussed. In the final section, we describe in vitro and in vivo studies where payloads have been tethered to a pathogen-derived agent to achieve specific delivery to neurons or the CNS.
Directed Mechanisms of CNS Entry by Pathogens and Toxins
Viruses
Viruses are perhaps nature's first targeted nanocarriers, depositing their bioactive payload not only within specific cell populations, but also to precisely targeted intracellular locations. Viral particle size and structure play important roles in determining viral infectivity.Citation6-8 Genomic material, either DNA or RNA, is encapsulated within a 20–750 nm viral capsid, a protein coat consisting of structurally similar subunits called capsomeres, whose composition ultimately dictates capsid size and shape. Viruses are classified structurally by the symmetry of their capsid, either as icosahedral, with 20 identical equilateral triangle faces arranged with 5:3:2 rotational symmetry, or helical, for which the protein coat is tightly wound around the viral genome to form a rod. Icosahedral viruses contain a minimum of 60 capsomeres with larger viruses containing additional capsomeres, termed hexameres, that are arranged along the flat faces of the icosahedron. Thus, additional volume for containing greater quantities of genomic material may be achieved by increasing the protein content of each face. Complex virus morphologies that incorporate both icosahedral and helical symmetries are also possible.
One of the first steps in viral anchorage to target tissue is their interaction with negatively charged glycosaminoglycans (GAGs) expressed on host cells, including heparin sulfate and chondroitin sulfate.Citation9 Many viruses are naked, or non-enveloped, in which case the capsid proteins are exposed for direct interaction with target cells.Citation10 Naked viruses replicate within the cytoplasm and, by necessity, must destroy the host cell to release viral particles. Other viruses are enveloped, whereby a host-derived lipid bilayer is obtained by passage of the viral particle through organelle or host cell membranes, which can alter both shape and flexibility of viral particles. Because the lipid bilayer is host-derived, the viral envelope contributes to immune evasion, facilitates interaction with target cells, and enables virus propagation to occur non-destructively.Citation11,12 Importantly, viral and host proteins incorporate with the envelope, either orienting centrally (matrix proteins, which engage capsid with envelope) or outwardly (glycoprotein spikes, which engage envelope with host cell). Overall viral avidity for host cells will be a balance between the receptor affinity and the number and arrangement of those receptors. Some evidence suggests that increasing glycoprotein-receptor affinity above a critical threshold does not further increase viral uptake.Citation13
The interaction of a virus with host membrane proteins not only brings the virus in close proximity to the host cell, but can also trigger conformational changes to exposed proteins or activate molecular machinery for viral internalization and transport. Envelope proteins are therefore important in mediating the virus release in to the cytoplasm, which makes these proteins a first lead in identifying potential ligands for receptor mediated internalization of synthetic constructs.Citation14,15 However, viral tropism is not determined exclusively by surface-host interactions. This is especially evident when considering that virus receptors are frequently distributed across a much broader range of tissues than what the virus is known to actually infect. Intracellular trafficking patterns and the relative abundance of necessary transcription factors for viral replication will contribute to selectivity of infection, thus determining overall virulence.
There are many examples of viruses that are known to be infective to the nervous system ().Citation14 Viruses achieve physical passage into the brain by a variety of mechanisms, including via transcellular, paracellular, immune cell-mediated, and retrograde routes. Some of these viruses – notably, rabies virus, human immunodeficiency virus, and West Nile virus—possess CNS tropism that is associated with their severe health burden. However, virulence typically results from the presence and replication of the virus, as opposed to acute neurotoxicity of the substances that the virus produces. Many viruses specialize in immune evasion strategies that contribute to their CNS tropism. The viral proteins that facilitate CNS invasion are therefore an interesting starting point to direct the tropism of engineered nanocarriers to treat human disease.
Table 1. Examples of neurotropic viruses and their receptors
Rabies virus
Rabies virus (RV) is well-adapted for CNS entry, and infection in humans without timely immunization is nearly unexceptionally fatal. Virus is introduced into muscle tissue through the saliva of an infected host, where it enters neurons for retrograde transport to the brain. Infection via aerosol exposure has also been reported.Citation15 The virus is understood to enrich at the neuromuscular junction (NMJ) or sensory neurons, after which it travels along axons at a speed of up to 3mm/hr to reach the brain. The RV envelope is comprised of a host-derived lipid membrane and both a matrix protein and a glycoprotein. The full-length type I transmembrane glycoprotein is 505 amino acids in length with 3 N-glycosylation sites, and it protrudes from the viral envelope as trimeric spikes. A 29 amino acid (aa) sequence of the rabies virus glycoprotein (RVG) was demonstrated in several independent studies to be a critical requirement for both entry and transport of virus in the CNS, although the molecular mechanisms by which entry and transport occur remain under discussion.Citation16 Potential receptors for RV include the p75 neurotrophin receptor (p75NTR), neural cell adhesion molecule (NCAM), and the nicotinic acetylcholine receptor (nAChR).Citation17-19 Virus is believed to interact with nAChR at the apex of NMJ folds, which could facilitate enrichment of RV to improve the efficiency of neuronal entry. There is evidence implicating NCAM in specific uptake of virus; for example, the susceptibility of different cell lines to RV infection was a function of NCAM expression, and infection-resistant lines were rendered susceptible when transfected with an NCAM-encoding gene.Citation18 Viral particles have been reported to achieve entry via adsorptive endocytosis, being localized in clathrin pits, uncoated vesicles, and ultimately lysosomes.Citation20
Human immunodeficiency virus
Human immunodeficiency virus (HIV) is the virus that causes acquired immunodeficiency syndrome (AIDS). Although HIV may be best known for its ability to infiltrate the human immune system (which is one of multiple mechanisms by which the virus achieves entry into the CNSCitation21), the HIV-derived trans-activating regulatory protein (Tat) has gained notoriety in its own right.Citation22 Tat (86–101 aa) is a small, positively charged protein that stabilizes transcription to support viral replication, and it is actively released from HIV-infected cells to produce a variety of biological effects. Among other functions, Tat is neurotoxic and immunosuppressive.Citation23,24 Peptides derived from the full sequence are cell penetrating and potently capable of crossing the BBB.Citation25 Of note, although the CNS is not the dominant target tissue for HIV virulence, Tat penetration of brain endothelial cells is well established in vitro and in vivo, with evidence presented for both active and passive (diffusion-mediated) mechanisms of entry.Citation25,26 Three cell-surface domains have been identified for active interaction of Tat with endothelial cells, including GAGs, integrins, and the vascular endothelial growth factor receptor-2.Citation27
Bacteria
Bacteria are ubiquitous in the human environment, displaying a broad diversity of sizes and shapes that have evolved over millions of years to facilitate their colonization and proliferation in a diverse set of environments. While bacteria are tremendously successful in achieving long-term and relatively innocuous or even beneficial residence within the human periphery, the presence of bacteria in the human CNS is almost always a severe health concern.
A typical bacterium is 500–5000 nm in length, taking on spherical (cocci), rod-shaped (bacilli), or more complex morphologies (e.g., vibrio, spirilla, spirochaetes, stella, haloarcula, or filamentous).Citation28 Bacteria are enveloped by an exoskeletal cell wall, termed the sacculus, which is composed of peptidoglycan, a sugar with alternating N-acetylglucosamine and N-acetylmuramic acid repeats.Citation29 A variety of bacteria are capable of entering the CNS, either directly via exposed protein and receptor interactions, through immune cells, or by altering BBB permeability.Citation4,30 It is important to note that CNS entry is not always the primary goal of bacterial pathogenesis, and in fact often results as a secondary infection (meningitis) that, if left untreated, will be rapidly fatal to the host.
Clostridium tetani and Clostridium botulinum
Perhaps the most widely appreciated neurotropic bacterial toxin is the tetanus toxin C-fragment (TTC), secreted by Clostridium tetani. Tetanus toxin enters the CNS via neuromuscular junctions to cause the spastic paralysis known as tetanus; with an LD50 of 1 ng/kg, intact toxin is quite deadly. However, when broken down into its 3 main components, one light chain (L, 50 kDa) and 2 heavy chains (HN and HC, 100kDa combined), independent functions for toxin pathophysiology are observed. TTC virulence originates from the L chain, which binds and cleaves the membrane protein VAMP and degrades synaptobrevin in inhibitory neurons, preventing the release of GABA and glycine and producing painful muscle spasms.Citation31,32 The HC (C-terminal) and HN (N-terminal) chains alone are non-toxic, being responsible for different phases of neuronal transport of the toxin to target inhibitory neurons. The HC (TTC) fragment of tetanus toxin binds to highly enriched areas of polysialogangliosides on peripheral nerve terminals of motor neurons. Once endocytosed, it is transported by retrograde routes to synaptic terminals connecting to inhibitory interneurons located within the spinal cord. Once within the spinal cord, the toxin is endocytosed into the connecting neuron, where the HN fragment burrows into the vesicle membrane, translocating the disulfide-linked L chain into the cytosol of the inhibitory neuron.Citation33,34
Given the neuronal-targeting action of the H fragments, TTC is an attractive candidate for facilitating drug delivery to neurons. However, one challenge to applying TTC in humans is that vaccination will promote rapid clearance of TTC linked agents by immune cells, which could prevent effective administration of TTC-linked agents. As of 2010, an estimated 64% of the United States population was reported by the CDC to have been vaccinated against tetanus.Citation35 Botulinum toxin (BoNT) is more potent than TTC, and immunization against the bacteria is infrequent in humans. Thus, the Hc fragment of BoNT-A, which also presents strong neuronal tropism, would be expected to be a candidate for CNS delivery. However, BoNT-A is known to exert its primary effect on the peripheral nervous system on cholinergic nerve terminals. The question of whether BoNT-A can achieve CNS entry has not yet been resolved.Citation36,37
Borrelia, Escherichia coli, and others
Members of the phylum spirochaetes include the neuroinvasive genera Treponema and Borrelia, which contain the species that cause syphilis and Lyme disease, respectively. Spirochaetes are long and slender bacteria, with a corkscrew-type, helical conformation that enables them to propel effectively through highly viscous fluids. The mechanisms that facilitate Borrelia infection in the CNS include immune evasion, ECM degradation via activation of host cell enzymes, and direct passage across the BBB. These activities are coordinated by a highly diverse set of surface proteins promoting pathogen interaction with host cells to achieve tissue tropism.Citation38,39 Borrelia mode of entry into the CNS remains under discussion, with evidence presented for both paracellular (via opening of tight junctions) and transcellular (via receptor mediated mechanisms) movement across brain endothelial cells. Borrelia interacts with a range of extracellular or cell-surface-associated host molecules, including fibronectin, glycosaminoglycans, integrins, and receptors such as CD40.Citation40,41
Although it is a relatively rare complication of human infection, the bacterium Escherichia coli is capable of achieving physical entry by paracellular routes into the CNS to cause bacterial meningitis. Bacterial passage through the BBB is believed to involve rearrangement of the host cell actin cytoskeleton via activation of Rho GTPases.Citation42,43 E. coli K1 invasion through human brain microvascular endothelial cells (HBMEC) was studied to identify ligands necessary for physical entry of the bacteria.Citation44 Outer-membrane protein A (OmpA) and type 1 fimbriae (FimH) on the surface of E. coli bind gp96 and CD48 on the surface of HBMEC, respectively, which will lead to cytoskeletal rearrangement to enable pathogen invasion.
The 37kDa/67kDa laminin receptor (LamR) has come to the forefront within the past decade as an important receptor for multiple neurotropic pathogens, both bacterial and viral (). E. coli, Streptococcus pneumoniae, Neisseria meningitidis, and Haemophilus influenzae are bacteria known to use LamR as a receptor for entry into the BBB; their presence in the CNS will produce bacterial meningitis.Citation45,46 Ligand binding domains on these organisms for LamR are conserved structurally, rather than on an amino acid level, and are typically found on surface-exposed loops of porins within the bacterial membrane.Citation45,47 Competitive binding studies have revealed that most bacterial ligands target the laminin binding site (residues 161-182) or an extracellular domain (residues 263-282) on LamR.Citation47
Table 2. Pathogenic ligands known to bind to 37/67kDa receptor (LamR)
Listeria
In both healthy and diseased brain, peripherally circulating leukocytes enter the brain parenchyma for immune surveillance of the CNS.Citation48 Bacteria, viruses, and protozoa are thus capable of infecting leukocytes as a means to achieve BBB passage. This process is termed the “Trojan horse” mechanism, and is a well-characterized point of entry for Listeria monocytogenes. Multiple in vitro and in vivo studies have demonstrated the ability of L. monocytogenes to cross the BBB via infected monocytes, macrophages, or microglia.Citation49-51 In vitro, L. monocytogenes is capable of directly crossing HBMECs with the aid of bacterial internalin proteins, which are thought to be the mechanism used to cross intestinal epithelium following bacterial ingestion.Citation52 In the presence of human serum, however, direct passage of L. monocytogenes through HBMECs is grossly inhibited. Citation50 These data suggest that entry is primarily mediated by circulating immune cells.
Eukaryotes: secreted toxins
Toxins secreted in animal venoms often contain complex mixtures of organic molecules and proteins that act in a variety of ways to affect cellular function. Spider venom, for example, contains over 10 million bioactive peptides, while cone snails produce over 1,000 conopeptides per species.Citation53 Given this high biological diversity, venom-derived peptides are of intense interest for therapeutic applications.Citation54 In nature, toxins are used for both defense and as a mechanism for immobilizing food sources, and thus venom-derived peptides must often be modified to circumvent toxicity while maintaining target specificity. The efficacy of native toxin action may also be improved by peptide engineering. For example, teprotide, a nonapeptide derived from the venom of the pit viper Bothrops jaraca, was found to have antihypertensive effects by inhibiting angiotensin converting enzyme (ACE). Its lack of oral bioavailability prompted the isolation of the peptide's active site, and further modifications eventually brought about captopril, an FDA-approved drug now prescribed for hypertension and congestive heart failure.
Bioactive peptides can alter cellular function via interaction with cell surface receptors or channels by occlusion or by modifying the properties of ion channel gates to interfere with the kinetics of channel opening. The diversity of venom-derived peptide structures is extremely broad; however, conserved function enables grouping. For example, many spider, cone snail, and snake derived neurotoxins that bind to calcium or sodium ion channels present a similar structural organization where the peptides form compact disulfide-bonded hydrophobic cores with short loops. This conserved structure is termed the inhibitor cystine knot motif (ICK).Citation55 It is important to recognize that tertiary peptide structure may be an important component of bioactivity and should be considered while modifying peptide chemistry. For example, many types of neurotropic peptides contain a high number of disulfide bridges for increased stability in biological fluids.Citation56 Although stability could be an advantage for maintaining bioactivity of ligands in vivo, disulfide-linked peptides are more difficult to produce synthetically.
Spiders
Voltage-gated ion channels, including calcium, potassium, and sodium, are a common target for neurotropic spider venoms. Hanatoxin1 (HaTx1) is a 35 amino acid peptide with an ICK motif extracted from the venom of the tarantula Grammostola spatulata. HaTx1 inhibits the Kv2.1 potassium channel by altering gating energetics.Citation57 Interestingly, HaTx1 also binds to voltage-gated calcium channels, suggesting that the receptor binding motif may be conserved among different ion channel types. Another class of potassium channel toxins contains the phrixotoxins, which are derived from the venom of the Chilean copper tarantula Phrixotrichus auratus and block Kv4 channels in a voltage-dependent manner.Citation58 There are millions of sodium channel toxins found in taxonomically diverse species, suggesting that this channel has been evolutionarily conserved and was potentially initiated at an early stage of venom gland evolution. For example, the d-HXTX-Ar1a toxin from the Sydney funnel-web spider, Atrax robustus, inhibits activation of the sodium ion channel, while μ-agatoxins from the American funnel spiders, Agalenopsis aperta, change the voltage to more negative potentials for channel activation and opening.Citation53 The venom of the Phoneutria genus of spiders, also known as the Brazilian wandering spiders, secrete venom containing phoneutria nigriventer toxin-3 (PhTx3), which is a broad-spectrum calcium channel inhibitor that affects glutamate transport. Components of Phoneutria nigriventer venom also have temporary but potent effects on the BBB, disrupting cell-cell junctions and reducing Pgp efflux pump function to increase permeability.Citation59 Perhaps most interestingly, this disruption is spatially heterogeneous and observed primarily in the hippocampus.Citation60 Peripherally administered dye achieved hippocampal entry via transcellular (microtubule-dependent) routes, with paracellular dye observed only in arterioles and venules, and not in capillaries. Venom neurotoxicity was not directly associated with increased BBB permeability, raising the intriguing possibility that venom components could be used for brain-region specific drug delivery.
Snakes and snails
Both snake and snail venoms contain peptide motifs that antagonize neuronal nicotinic acetylcholine receptors (nAChR). The nAChR is a pentameric integral membrane protein complex arranged around a central pore permeable to cations. The neuronal receptors are composed of homomeric species or a mixture of α- or β- subunits. Receptor subunit composition is specific to different tissue types, making the nAChR a promising lead for achieving CNS tropism. For example, the α7-nAChR is widely expressed in the brain and spinal cord, while α9- and α10-nAChR have not been found in the brain, being restricted to the ear and some ganglia.Citation61
Snake venom members include 3-finger toxins, α-neurotoxins, and muscarinic toxins, among others.Citation62 Three-finger toxins (TFT) contain 2 major structural domains: the 3-finger domain which is responsible for binding the nAChR and forming an interaction with the target cell membrane, and a globular region which is also necessary for membrane interaction.Citation63 NAChR-toxin complexes cross cells via endocytosis, which occurs in 2 distinct phases. In the first phase, the complex is isolated within a vesicle induced by a Rac1 GTPase-initiated actin polymerization step. This is followed by the second phase where the vesicle is delivered to the lysosome, a mechanism similar to the internalization of lipoproteins by macrophages.Citation64
TFTs are often classified by toxin length. lists BBB-specific long alpha neurotoxins, which bind to homopentameric neuronal nAChR that are typically comprised of α7 or α3 subunits.Citation65-67 A well-known example of a TFT nAChR agonist is α-bungarotoxin (α-Bgtx), which is derived from the genus of venomous snakes known as Kraits.Citation63 Studies of α-Bgtx have shown the second loop interacts directly with the nAChR, directly followed by an influx of CaCitation2+.Citation68
Table 3. Three-finger toxins that specifically target nAChR and have been associated with BBB penetration
Conopeptides, or conotoxins, are isolated from marine cone snails from the genus Conus. Predatory cone snails use their venom to incapacitate worms, mollusks, and small fish.Citation69,70 Several conopeptides are in clinical trial. Ziconotide (Prialt) is a conotoxin derived drug that is clinically approved and marketed as a therapy to reduce severe pain. Conopeptides act on voltage-gated ion channels, ligand-gated ion channels, G-protein-coupled receptors and neurotransmitter receptors.Citation71 The α-conotoxins are between 10–30 amino acids and typically contain disulfide bonds. Although they are not members of the TFT family, these peptides demonstrate a diverse array of bioactivity, including their ability to antagonize the nAChR by interacting with the same domain as α-Bgtx. The α-conotoxins have shown specificity to various nAChR α-subunits, with a number of α-conotoxins identified that specifically interact with α7-nAChR, which are expressed highly in brain ().Citation61,72
Table 4. Conopeptides that specifically interact with nAChR and are associated with BBB penetration
One potential challenge to conotoxin-based drug delivery strategies is that α-conotoxins containing the sequence of XCCXPACGXXXXCX are on the CDC's select agent list, which would severely limit their laboratory investigation. This regulation was put into place due to the high affinity and acute toxicity that these peptides display. Under these regulations, no research or commercial entity may possess more than 100 mg at a time without conforming to significant US federal security protocols.Citation73
Scorpions
Scorpion venom contains hundreds of individual components, including peptides, proteins, lipids, amines, and nucleotides. The amino acid sequence of at least 250 individual neurotoxins has been determined, with the majority comprising short (23–67 aa), disulfide-bonded peptides. These peptides primarily target ion channels, and are of focused interest as biopharmaceuticals.Citation74 Chlorotoxin (CTX) is one particularly interesting peptide taken from the venom of Leiurus quinquestriatus, or deathstalker scorpion, that blocks chloride-selective ion channels. CTX also appears to interact specifically with matrix metalloprotease-2 (MMP-2) that is expressed in high levels in brain tumor cells but not expressed in normal tissue. In one study, CTX demonstrated remarkable histological specificity for primary brain tumor and primitive neuroectodermal tumors.Citation75 CTX binding was undetectable in normal brain (neurological disease or healthy brain controls), skin, kidney, or lung. Evidence for the ability of CTX and CTX-bound agents to bypass the BBB is found in translational drug delivery work, discussed below. With 8 cysteines forming 4 disulfide bonds, CTX is relatively resistant to hydrolysis, but expensive to produce. It remains uncertain how disulfide binding contributes to function. Given the varied tropism of scorpion neurotoxins for specific receptors that are expressed differentially in the peripheral and central nervous system, the potential for targeted therapy is high.Citation76 Citation77
Drug Delivery
Nature is a rich source of biomimicry, having evolved a multitude of strategies that could be used as inspiration for engineering CNS-targeted nanocarriers (). Some pathogens, notably viruses and certain bacteria, enter the CNS in whole form, achieving direct physical passage across cells to infect the brain. Other pathogens, including bacteria and multicellular eukaryotic organisms, secrete toxins that preferentially interact with neurons to exert a broad range of biological effects on peripheral and central neurons. In many instances, pathogen-type strategies would be considered dangerous. For example, whole tetanus toxin causes painful muscle spasms. However, isolation of the minimally necessary component of the protein that enables its neurotropism achieves neuronal targeting without deleterious effect. These observations suggest that pathogen-derived strategies for achieving CNS entry could be engineered to facilitate the accumulation of synthetic or biologically derived nanocarriers in the brain. Here, we will review drug delivery literature reporting pathogenic mechanisms for achieving neuronal targeting.
Figure 2. Bio-inspired nanocarriers can be engineered to improve drug delivery to the CNS. In this example, a solid polymer nanoparticle encapsulates a therapeutic (small molecule, nucleic acid, or protein) or imaging agent. The surface of the viral-sized nanoparticle is modified to display a pathogen-derived peptide (e.g., rabies virus glycoprotein) that will facilitate passage of nanoparticle with cargo across the BBB. Therapeutic compounds that have been encapsulated in biodegradable nanoparticles will be released slowly over time for targeted treatment. A solid polymer nanoparticle is shown as one example of a targeted drug carrier; many other types of carriers or conjugates can be similarly modified to improve CNS delivery (for example, liposomes, micelles, drug-antibody conjugates, and othersCitation115).
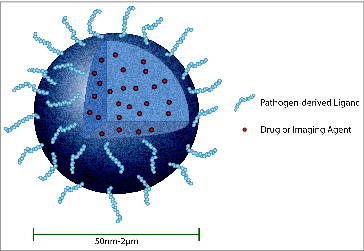
Example strategies for delivering payloads to the brain via pathogenic mechanisms are summarized in . Several themes emerge. First, pathogenic ligands may be tethered to a therapeutic (small molecule, protein, or gene), to a vehicle containing therapeutic, or to an imaging agent. Tethered agents include low molecular weight polymers, protein carriers, and viral-sized nanoparticles. Second, when the pathogenic ligand is tethered to a vehicle capable of releasing its payload before reaching its target cell, demonstrating true target specificity can be challenging. Third, the majority of characterization is performed in vitro, typically by utilizing fluorescence microscopy or competitive binding assays to determine how modification of a ligand mediates specific uptake of the payload into target cells. In vivo evaluation sometimes involves measurement of uptake or distribution in the CNS by fluorescence or radiographic methods, however, more compelling evidence is often provided by therapeutic endpoints. For example, Alvarez-Erviti et al. engineered self-derived exosomes to express the neurotropic ligand RVG on their surface.Citation78 Exosomes were loaded with siRNA against GAPDH and delivered systemically, with knockdown observed specifically in neurons, microglia, and oligodendrocytes in the brain. In separate experiments, delivery of siRNA against BACE1 was confirmed at both the mRNA and protein level. Since siRNA must be delivered intracellularly to achieve gene silencing, these results provide compelling evidence that exosomes targeted to the CNS with RVG actually reach specific cellular targets within the brain parenchyma.
Table 5. Examples of CNS-directed delivery achieved by pathogenic strategies
Both RVG and CTX have been recent but highly popular candidates for improving brain-specific delivery of systemically administered carriers, the former achieving impressive preclinical success in carrying therapeutics to the CNS in rodent models, and the latter being a component of a targeted radiotherapy that reached phase II clinical trial (NCT00114309). One reason that RVG has risen as a surface-modifying ligand may be the fact that the portion of glycoprotein understood to be responsible for its highly specific CNS tropism is known and of reasonably short length (29-aa). The peptide is commercially available and can be conjugated to the surface of a nanocarrier with well-defined chemistry that would be expected to preserve orientation of the ligand for interaction with its cell surface receptor. The short sequence of RVG should be taken in direct contrast to what is – or isn’t, as the case may be – known about other viral envelope proteins, whose full sequences can be hundreds of amino acids long and may possess multiple binding sites for attachment to the surface of a nanocarrier.
The first report describing delivery of a therapeutic payload linked to RVG was published in 2007 by Kumar and colleagues and serves as an excellent example of the direct translation of viral strategies for CNS entry to improve the brain-specific delivery of synthetic nanocarriers.Citation79 RVG-pseudotyped lentivirus was observed to be infective to neuronal (Neuro2A) but not peripheral (HeLa) cells, whereas vesicular stomatitis virus (VSV) pseudotyped vectors were infective to both cell types. This result is perhaps not unsurprising, given the broad peripheral tropism of VSV. Correspondingly, RVG-pseudotyped virus encoding antiviral siRNA protected against Japanese encephalitis virus challenge, whereas VSV pseudotyped vectors did not provide protection. RVG was then linked to a 9-arginine repeat to carry antiviral siRNA from peripheral circulation into the brain; 80% of mice treated with RVG-linked antiviral siRNA survived JEV challenge, which was fatal in mice treated with antiviral siRNA linked to a control rabies virus matrix protein (RVMAT). Since this initial report, RVG-linked polymers, liposomes, and biologically derived nanocarriers have been used for successful delivery of proteins, small molecules, and nucleic acids to the CNS, with functionally improved outcomes in several distinct disease models. This research presents compelling evidence that pathogenic strategies for achieving CNS entry can be adapted to improve delivery of engineered drug carriers.
The first experiments demonstrating affinity of CTX for brain tumor cells were published in 1998 by Soroceanu et al.Citation80 I-125-labeled CTX was shown to bind to at least two sites (high and low affinity) on glioma cells, with impressive specificity of delivery to orthotopic glioma xenografts (39% of ID/g in tumor bearing versus 12% ID/g in healthy brain). Subsequent studies have suggested very high specificity of CTX binding to malignant brain tumor cells in patient biopsy samples compared to non-target tissue, with CTX staining more than 90% of brain tumor cells, compared to almost no detectable staining in uninvolved brain tissue.Citation80 When CTX-linked Cy5.5 was delivered systemically to mice bearing orthotopic medulloblastoma, fluorescence was detected widely in tumor and not in healthy brain, in spite of an apparently intact BBB, which was demonstrated by the lack of Evan's Blue dye extravasation from the vascular compartment and albumin immunostaining.Citation81 These data suggest that CTX agents may be capable of bypassing intact BBB, although specific mechanisms of entry have yet to be characterized. I-131-labeled chlorotoxin (TM-601) reached phase II clinical trial for targeted radiotherapy by direct application to the tumor resection cavity. Phase I results demonstrated that intracavitary administration of TM-601 was safe, with no dose-limiting toxicity observed and some evidence of therapeutic benefit.Citation82 In the time since the phase I results were reported, additional preclinical work has not only confirmed specificity of CTX for a range of malignant cell types, but also demonstrated the ability of CTX to ferry systemically administered agents into the brain.Citation83-105
KC2S, a synthetic peptide derived from toxin b of the king cobra (Ophiophagus hannah), demonstrates similar affinity for neuronal nAChR as RVG, as judged by competitive inhibition assay against α-Bgtx (IC50s of 33nM and 28nM for KC2S and RVG, respectively). KC2S-linked micelles were taken up in brain capillary endothelial cells but not in HeLa cells.Citation106 When delivered systemically, dye-loaded KC2S-micelles were observed to accumulate slowly in the brain, and drug-loaded KC2S-micelles provided a modest survival benefit in an orthotopic glioma model. Interestingly, although blood pharmacokinetic parameters were nearly identical for KC2S-targeted and non-targeted micelles, Cmax and AUC in the brain was significantly higher for the targeted formulation, suggesting active transport of toxin linked micelles from blood to brain. Toxins from snails have also been used to deliver tethered payload to neuronal cells, although work remains to test delivery effectiveness in vivo.Citation104,107
TTC bound agents are popular tools for studying neuronal transport and have been used to achieve targeting in vivo. The specificity of TTC for gangliosides predominantly concentrated in neuronal membranes allows TTC-tethered agents to selectively bind neurons. For example, a recombinant fusion of TTC and wild type SOD-1, a protein whose mutant form is implicated in the disease pathology of amyotrophic lateral sclerosis (ALS), was found to have a significantly higher spread and retention compared to free SOD-1 when infused into the brain of mice.Citation108 In another study, Ciriza et al. demonstrated that weekly limb injection of a TTC-GDNF fusion, a neurotrophic factor known to increase the survival and proliferation of neurons, was able to increase survival and quality of life in SOD-1 mutant mice, while the injection of an insect recombinant TTC-IGF-1 fusion protein in SOD-1 mutant mice was able to sufficiently reach the spinal cord but not affect the overall survival in treatment groups.Citation109,110 Perhaps most interestingly, evidence for retrograde transport of SOD-1-TTC to the brain was found after intramuscular injection in mice, raising the question of whether a larger carrier (for example, a drug loaded nanoparticle), could achieve CNS entry by retrograde route.Citation111 For a more comprehensive review of therapeutic recombinant fusion TTC proteins, the authors refer the reader to Toivonen et al.Citation31
We focus here primarily on ligand-receptor strategies for receptor-mediated delivery of therapeutic payloads. However, it is important to note that for certain pathogenic sequences, including Tat, peptide-modified nanoparticle entry may occur via non-receptor mediated mechanisms.Citation112 In several intriguing and recent reports, non-receptor mediated biomimicry has been used to engineer the interaction of nanocarriers with cell targets. For example, Ng and colleagues mimicked bacterial surface composition to target intracellular actin networks, with the ultimate goal of overcoming diffusional barriers to actively propel polymer or lipid nanoparticles through the cytoplasm.Citation113 In other work, Niu et al. mimicked viral surface topography to dramatically enhance silica nanoparticle internalization by cells.Citation114 Viral and bacterial derived carriers have also been used as vehicles for targeted drug delivery, although the emerging field of biologically sourced nanocarriers remains beyond the scope of this review. These and other examples highlight the potential broad range of pathogen-derived strategies that could be used as inspiration for engineering targeted drug delivery vehicles.
Conclusion
Drug delivery remains a critical challenge to achieving better therapy of disease in the CNS. Most systemically delivered drugs do not cross the BBB, and those that do are often poorly bioavailable. However, many viruses, bacteria, and neurotoxins are well-adapted for achieving CNS entry. A variety of pathogenic mechanisms have already been used to improve the brain or neuronal tropism of drugs, drug-loaded vehicles, or imaging agents. These strategies have the potential to improve delivery of peripherally administered agents to the CNS, which could facilitate the translation of novel therapeutics to the clinic to treat human disease.
Disclosure of Potential Conflicts of Interest
No potential conflicts of interest were disclosed.
Acknowledgments
We thank Danielle DiPerna for providing detailed edits and feedback.
Funding
This review was supported by grants from the Barrow Neurological Foundation (RS), the Ben and Catherine Ivy Foundation (RS, RM, TPC) and the NIH U54 CA151881(TPC).
References
- Friden PM, Walus LR, Musso GF, Taylor MA, Malfroy B, Starzyk RM. Anti-transferrin receptor antibody and antibody-drug conjugates cross the blood-brain barrier. Proc Natl Acad Sci 1991; 88:4771-5.
- Pardridge WM. The blood-brain barrier: bottleneck in brain drug development. NeuroRx 2005; 2:3-14; PMID:15717053; http://dx.doi.org/10.1602/neurorx.2.1.3
- Bencurova E, Mlynarcik P, Bhide M. An insight into the ligand-receptor interactions involved in the translocation of pathogens across blood-brain barrier. FEMS Immunol Med Microbiol 2011; 63:297-318; PMID:22092557; http://dx.doi.org/10.1111/j.1574-695X.2011.00867.x
- Kim KS. Mechanisms of microbial traversal of the blood-brain barrier. Nat Rev Microbiol 2008; 6:625-34; PMID:18604221
- Salinas S, Schiavo G, Kremer EJ. A hitchhiker's guide to the nervous system: the complex journey of viruses and toxins. Nat Rev Microbiol 2010; 8:645-55; PMID:20706281; http://dx.doi.org/10.1038/nrmicro2395
- Donaldson EF, Lindesmith LC, Lobue AD, Baric RS. Viral shape-shifting: norovirus evasion of the human immune system. Nat Rev Microbiol 2010; 8:231-41; PMID:20125087; http://dx.doi.org/10.1038/nrmicro2296
- Moody MF. Geometry of phage head construction. J Mol Biol 1999; 293:401-33; PMID:10529353
- Morton VL, Stockley PG, Stonehouse NJ, Ashcroft AE. Insights into virus capsid assembly from non-covalent mass spectrometry. Mass Spectrom Rev 2008; 27:575-95; PMID:18498137; http://dx.doi.org/10.1002/mas.20176
- Jolly CL, Sattentau QJ. Attachment factors. Adv Exp Med Biol 2013; 790:1-23; PMID:23884583; http://dx.doi.org/10.1007/978-1-4614-7651-1_1
- Mateu MG. Assembly, stability and dynamics of virus capsids. Arch Biochem Biophys 2013; 531:65-79; PMID:23142681
- Dokland T. Freedom and restraint: themes in virus capsid assembly. Structure 2000; 8:R157-62; PMID:10997898; http://dx.doi.org/10.1016/S0969-2126(00)00181-7
- Wu E, Nemerow GR. Virus yoga: the role of flexibility in virus host cell recognition. Trends Microbiol 2004; 12:162-9; PMID:15051066; http://dx.doi.org/10.1016/j.tim.2004.02.005
- Hasegawa K, Hu C, Nakamura T, Marks JD, Russell SJ, Peng KW. Affinity thresholds for membrane fusion triggering by viral glycoproteins. J Virol 2007; 81:13149-57; PMID:17804513; http://dx.doi.org/10.1128/JVI.01415-07
- McGavern DB, Kang SS. Illuminating viral infections in the nervous system. Nat Rev Immunol 2011; 11:318-29; PMID:21508982; http://dx.doi.org/10.1038/nri2971
- Johnson N, Phillpotts R, Fooks AR. Airborne transmission of lyssaviruses. J Med Microbiol 2006; 55:785-90; PMID:16687600; http://dx.doi.org/10.1099/jmm.0.46370-0
- Lafon M. Rabies virus receptors. J Neurovirol 2005; 11:82-7
- Langevin C, Jaaro H, Bressanelli S, Fainzilber M, Tuffereau C. Rabies virus glycoprotein (RVG) is a trimeric ligand for the N-terminal cysteine-rich domain of the mammalian p75 neurotrophin receptor. J Biol Chem 2002; 277:37655-62; PMID:12163480; http://dx.doi.org/10.1074/jbc.M201374200
- Thoulouze MI, Lafage M, Schachner M, Hartmann U, Cremer H, Lafon M. The neural cell adhesion molecule is a receptor for rabies virus. J Virol 1998; 72:7181-90; PMID:9696812
- Lentz TL. Rabies virus binding to an acetylcholine receptor alpha-subunit peptide. J Mol Recognit 1990; 3:82-8
- Superti F, Derer M, Tsiang H. Mechanism of rabies virus entry into CER cells. J Gen Virol 1984; 65(Pt 4):781-9; PMID:6423770; http://dx.doi.org/10.1099/0022-1317-65-4-781
- Ivey NS, MacLean AG, Lackner AA. Acquired immunodeficiency syndrome and the blood-brain barrier. J Neurovirol 2009; 15:111-22; PMID:19306229; http://dx.doi.org/10.1080/13550280902769764
- Strazza M, Pirrone V, Wigdahl B, Nonnemacher MR. Breaking down the barrier: the effects of HIV-1 on the blood-brain barrier. Brain Res 2011; 1399:96-115; PMID:21641584; http://dx.doi.org/10.1016/j.brainres.2011.05.015
- King JE, Eugenin EA, Buckner CM, Berman JW. HIV tat and neurotoxicity. Microbes Infect 2006; 8:1347-57; PMID:16697675; http://dx.doi.org/10.1016/j.micinf.2005.11.014
- Cohen SS, Li C, Ding L, Cao Y, Pardee AB, Shevach EM, Cohen DI. Pronounced acute immunosuppression in vivo mediated by HIV Tat challenge. Proc Natl Acad Sci U S A 1999; 96:10842-7; PMID:10485913; http://dx.doi.org/10.1073/pnas.96.19.10842
- Banks WA, Robinson SM, Nath A. Permeability of the blood-brain barrier to HIV-1 Tat. Exp Neurol 2005; 193:218-27; PMID:15817280; http://dx.doi.org/10.1016/j.expneurol.2004.11.019
- Qin Y, Zhang Q, Chen H, Yuan W, Kuai R, Xie F, Zhang L, Wang X, Zhang Z, Liu J, et al. Comparison of four different peptides to enhance accumulation of liposomes into the brain. J Drug Target 2012; 20:235-45; PMID:22188312; http://dx.doi.org/10.3109/1061186X.2011.639022
- Rusnati M, Presta M. HIV-1 Tat protein and endothelium: from protein/cell interaction to AIDS-associated pathologies. Angiogenesis 2002; 5:141-51; PMID:12831055; http://dx.doi.org/10.1023/A:1023892223074
- Margolin W. Sculpting the bacterial cell. Curr Biol 2009; 19:R812-22; PMID:19906583
- Typas A, Banzhaf M, Gross CA, Vollmer W. From the regulation of peptidoglycan synthesis to bacterial growth and morphology. Nat Rev Microbiol 2012; 10:123-36;
- Huang SH, Jong AY. Cellular mechanisms of microbial proteins contributing to invasion of the blood-brain barrier. Cell Microbiol 2001; 3:277-87; PMID:11298651; http://dx.doi.org/10.1046/j.1462-5822.2001.00116.x
- Toivonen JM, Olivan S, Osta R. Tetanus toxin C-fragment: the courier and the cure? Toxins 2010; 2:2622-44; PMID:22069568; http://dx.doi.org/10.3390/toxins2112622
- Rossetto O, Scorzeto M, Megighian A, Montecucco C. Tetanus neurotoxin. Toxicon: Off J Int Soc Toxinol 2013; 66:59-63; PMID:23419592; http://dx.doi.org/10.1016/j.toxicon.2012.12.027
- Matsuda M, Lei DL, Sugimoto N, Ozutsumi K, Okabe T. Isolation, purification, and characterization of fragment B, the NH2-terminal half of the heavy chain of tetanus toxin. Infect Immun 1989; 57:3588-93; PMID:2478476
- Boquet P, Duflot E. Tetanus toxin fragment forms channels in lipid vesicles at low pH. Proc Natl Acad Sci U S A 1982; 79:7614-8; PMID:6296842; http://dx.doi.org/10.1073/pnas.79.24.7614
- Centers for Disease Control and Prevention. Adult vaccination coverage–United States, 2010. MMWR Morbidity and mortality weekly report 2012; 61:66-72.
- Akaike N, Shin MC, Wakita M, Torii Y, Harakawa T, Ginnaga A, Kato K, Kaji R, Kozaki S. Transsynaptic inhibition of spinal transmission by A2 botulinum toxin. J Physiol 2013; 591:1031-43; PMID:23109108; http://dx.doi.org/10.1113/jphysiol.2012.242131
- Antonucci F, Rossi C, Gianfranceschi L, Rossetto O, Caleo M. Long-distance retrograde effects of botulinum neurotoxin A. J Neurosci: Off J Soc Neurosci 2008; 28:3689-96; PMID:18385327; http://dx.doi.org/10.1523/JNEUROSCI.0375-08.2008
- Pulzova L, Bhide M. Outer surface proteins of Borrelia: peerless immune evasion tools. Curr Protein Pept Sci 2014; 15:75-88; PMID:24555888; http://dx.doi.org/10.2174/1389203715666140221124213
- Leong JM, Wang H, Magoun L, Field JA, Morrissey PE, Robbins D, Tatro JB, Coburn J, Parveen N. Different classes of proteoglycans contribute to the attachment of Borrelia burgdorferi to cultured endothelial and brain cells. Infect Immun 1998; 66:994-9; PMID:9488387
- Moriarty TJ, Shi M, Lin YP, Ebady R, Zhou H, Odisho T, Hardy PO, Salman-Dilgimen A, Wu J, Weening EH, et al. Vascular binding of a pathogen under shear force through mechanistically distinct sequential interactions with host macromolecules. Mol Microbiol 2012; 86:1116-31; PMID:23095033; http://dx.doi.org/10.1111/mmi.12045
- Pulzova L, Kovac A, Mucha R, Mlynarcik P, Bencurova E, Madar M, Novak M, Bhide M. OspA-CD40 dyad: ligand-receptor interaction in the translocation of neuroinvasive Borrelia across the blood-brain barrier. Sci Rep 2011; 1:86; PMID:22355605; http://dx.doi.org/10.1038/srep00086
- Reddy MA, Prasadarao NV, Wass CA, Kim KS. Phosphatidylinositol 3-kinase activation and interaction with focal adhesion kinase in Escherichia coli K1 invasion of human brain microvascular endothelial cells. J Biol Chem 2000; 275:36769-74; PMID:10973983; http://dx.doi.org/10.1074/jbc.M007382200
- Schmidt G, Sehr P, Wilm M, Selzer J, Mann M, Aktories K. Gln 63 of Rho is deamidated by Escherichia coli cytotoxic necrotizing factor-1. Nature 1997; 387:725-9; PMID:9192900; http://dx.doi.org/10.1038/42735
- Khan NA, Shin S, Chung JW, Kim KJ, Elliott S, Wang Y, Kim KS. Outer membrane protein A and cytotoxic necrotizing factor-1 use diverse signaling mechanisms for Escherichia coli K1 invasion of human brain microvascular endothelial cells. Microb Pathogenesis 2003; 35:35-42; PMID:12860457; http://dx.doi.org/10.1016/S0882-4010(03)00090-1
- Abouseada NM, Assafi MS, Mahdavi J, Oldfield NJ, Wheldon LM, Wooldridge KG, Ala'Aldeen DA. Mapping the laminin receptor binding domains of Neisseria meningitidis PorA and Haemophilus influenzae OmpP2. PloS One 2012; 7:e46233; PMID:23049988
- Kim KJ, Chung JW, Kim KS. 67-kDa laminin receptor promotes internalization of cytotoxic necrotizing factor 1-expressing Escherichia coli K1 into human brain microvascular endothelial cells. J Biol Chem 2005; 280:1360-8; PMID:15516338; http://dx.doi.org/10.1074/jbc.M410176200
- Orihuela CJ, Mahdavi J, Thornton J, Mann B, Wooldridge KG, Abouseada N, Oldfield NJ, Self T, Ala'Aldeen DA, Tuomanen EI. Laminin receptor initiates bacterial contact with the blood brain barrier in experimental meningitis models. J Clin Investi 2009; 119:1638-46; PMID:19436113; http://dx.doi.org/10.1172/JCI36759
- Ransohoff RM, Kivisakk P, Kidd G. Three or more routes for leukocyte migration into the central nervous system. Nat Rev Immunol 2003; 3:569-81; PMID:12876559; http://dx.doi.org/10.1038/nri1130
- Drevets DA, Dillon MJ, Schawang JS, Van Rooijen N, Ehrchen J, Sunderkotter C, Leenen PJ. The Ly-6Chigh monocyte subpopulation transports Listeria monocytogenes into the brain during systemic infection of mice. J Immunol 2004; 172:4418-24; http://dx.doi.org/10.4049/jimmunol.172.7.4418
- Hertzig T, Weber M, Greiffenberg L, Holthausen BS, Goebel W, Kim KS, Kuhn M. Antibodies present in normal human serum inhibit invasion of human brain microvascular endothelial cells by Listeria monocytogenes. Infect Immun 2003; 71:95-100; PMID:12496153; http://dx.doi.org/10.1128/IAI.71.1.95-100.2003
- Remuzgo-Martinez S, Pilares-Ortega L, Icardo JM, Valdizan EM, Vargas VI, Pazos A, Ramos-Vivas J. Microglial activation and expression of immune-related genes in a rat ex vivo nervous system model after infection with Listeria monocytogenes. Glia 2013; 61:611-22; PMID:23322603; http://dx.doi.org/10.1002/glia.22459
- Greiffenberg L, Goebel W, Kim KS, Weiglein I, Bubert A, Engelbrecht F, Stins M, Kuhn M. Interaction of Listeria monocytogenes with human brain microvascular endothelial cells: InlB-dependent invasion, long-term intracellular growth, and spread from macrophages to endothelial cells. Infect Immun 1998; 66:5260-7; PMID:9784531
- Klint JK, Senff S, Rupasinghe DB, Er SY, Herzig V, Nicholson GM, King GF. Spider-venom peptides that target voltage-gated sodium channels: pharmacological tools and potential therapeutic leads. Toxicon: Off J Int Soc Toxinol 2012; 60:478-91; PMID:22543187; http://dx.doi.org/10.1016/j.toxicon.2012.04.337
- King GF. Venoms as a platform for human drugs: translating toxins into therapeutics. Expert Opin Biol Ther 2011; 11:1469-84; PMID:21939428; http://dx.doi.org/10.1517/14712598.2011.621940
- Norton RS, Pallaghy PK. The cystine knot structure of ion channel toxins and related polypeptides. Toxicon: Off J Int Soc Toxinol 1998; 36:1573-83; PMID:9792173; http://dx.doi.org/10.1016/S0041-0101(98)00149-4
- Mouhat S, Jouirou B, Mosbah A, De Waard M, Sabatier JM. Diversity of folds in animal toxins acting on ion channels. Biochem J 2004; 378:717-26; PMID:14674883; http://dx.doi.org/10.1042/BJ20031860
- Takahashi H, Kim JI, Min HJ, Sato K, Swartz KJ, Shimada I. Solution structure of hanatoxin1, a gating modifier of voltage-dependent K(+) channels: common surface features of gating modifier toxins. J Mol Biol 2000; 297:771-80; PMID:10731427; http://dx.doi.org/10.1006/jmbi.2000.3609
- Chagot B, Escoubas P, Villegas E, Bernard C, Ferrat G, Corzo G, Lazdunski M, Darbon H. Solution structure of Phrixotoxin 1, a specific peptide inhibitor of Kv4 potassium channels from the venom of the theraphosid spider Phrixotrichus auratus. Protein Sci 2004; 13:1197-208; PMID:15096626; http://dx.doi.org/10.1110/ps.03584304
- Raposo C, Odorissi PA, Oliveira AL, Aoyama H, Ferreira CV, Verinaud L, Fontana K, Ruela-de-Sousa RR, da Cruz-Hofling MA. Effect of Phoneutria nigriventer venom on the expression of junctional protein and P-gp efflux pump function in the blood-brain barrier. Neurochem Res 2012; 37:1967-81; PMID:22684283; http://dx.doi.org/10.1007/s11064-012-0817-y
- de Paula Le Sueur L, Kalapothakis E, da Cruz-Hofling MA. Breakdown of the blood-brain barrier and neuropathological changes induced by Phoneutria nigriventer spider venom. Acta Neuropathol 2003; 105:125-34; PMID:12536223
- Gotti C, Zoli M, Clementi F. Brain nicotinic acetylcholine receptors: native subtypes and their relevance. Trends Pharmacol Sci 2006; 27:482-91; PMID:16876883; http://dx.doi.org/10.1016/j.tips.2006.07.004
- Harvey AL. Snake venom peptides. Handbook of Biologically Active Peptides 2006:355-62.
- Kini RM, Doley R. Structure, function and evolution of three-finger toxins: mini proteins with multiple targets. Toxicon: Off J Int Soc Toxinol 2010; 56:855-67; PMID:20670641; http://dx.doi.org/10.1016/j.toxicon.2010.07.010
- Kumari S, Borroni V, Chaudhry A, Chanda B, Massol R, Mayor S, Barrantes FJ. Nicotinic acetylcholine receptor is internalized via a Rac-dependent, dynamin-independent endocytic pathway. J Cell Biol 2008; 181:1179-93; PMID:18591431; http://dx.doi.org/10.1083/jcb.200709086
- Osipov AV, Rucktooa P, Kasheverov IE, Filkin SY, Starkov VG, Andreeva TV, Sixma TK, Bertrand D, Utkin YN, Tsetlin VI. Dimeric alpha-Cobratoxin X-ray Structure: Localization of intermolecular disulfides and possible mode of binding to nicotinic acetylcholine receptors*. J Biol Chem 2012; 287:6725-34; PMID:22223648; http://dx.doi.org/10.1074/jbc.M111.322313
- Alama A, Bruzzo C, Cavalieri Z, Forlani A, Utkin Y, Casciano I, Romani M. Inhibition of the nicotinic acetylcholine receptors by cobra venom alpha-neurotoxins: is there a perspective in lung cancer treatment? PloS One 2011; 6:e20695; PMID:21695184
- Lesovoy DM, Bocharov EV, Lyukmanova EN, Kosinsky YA, Shulepko MA, Dolgikh DA, Kirpichnikov MP, Efremov RG, Arseniev AS. Specific membrane binding of neurotoxin II can facilitate its delivery to acetylcholine receptor. Biophys J 2009; 97:2089-97; PMID:19804741; http://dx.doi.org/10.1016/j.bpj.2009.07.037
- Colquhoun D, Unwin N, Shelley C, Hatton C, Sivilotti L. Burger's medicinal chemistry and drug discovery. New York: Wiley-Interscience; 2003.
- Redwan el RM. Animal-derived pharmaceutical proteins. J Immunoassay Immunochem 2009; 30:262-90; PMID:19591041; http://dx.doi.org/10.1080/15321810903084400
- Essack M, Bajic VB, Archer JA. Conotoxins that confer therapeutic possibilities. Marine drugs 2012; 10:1244-65; PMID:22822370; http://dx.doi.org/10.3390/md10061244
- Lewis RJ, Dutertre S, Vetter I, Christie MJ. Conus venom peptide pharmacology. Pharmacol Rev 2012; 64:259-98; PMID:22407615; http://dx.doi.org/10.1124/pr.111.005322
- Hone AJ, Ruiz M, Scadden M, Christensen S, Gajewiak J, Azam L, McIntosh JM. Positional scanning mutagenesis of alpha-conotoxin PeIA identifies critical residues that confer potency and selectivity for alpha6/alpha3beta2beta3 and alpha3beta2 nicotinic acetylcholine receptors. J Biol Chem 2013; 288:25428-39; PMID:23846688; http://dx.doi.org/10.1074/jbc.M113.482059
- National Select Agent Registry. Centers for Disease Control; 2013; http://www.selectagents.gov/Select%20Agents%20and%20Toxins%20List.html
- Ding J, Chua PJ, Bay BH, Gopalakrishnakone P. Scorpion venoms as a potential source of novel cancer therapeutic compounds. Exp Biol Med (Maywood) 2014; 239(4):387-93.
- Lyons SA, O'Neal J, Sontheimer H. Chlorotoxin, a scorpion-derived peptide, specifically binds to gliomas and tumors of neuroectodermal origin. Glia 2002; 39:162-73; PMID:12112367; http://dx.doi.org/10.1002/glia.10083
- Gordon D, Gurevitz M. The selectivity of scorpion alpha-toxins for sodium channel subtypes is determined by subtle variations at the interacting surface. Toxicon: Off J Int Soc Toxinol 2003; 41:125-8; PMID:12565730; http://dx.doi.org/10.1016/S0041-0101(02)00294-5
- Zhijian C, Feng L, Yingliang W, Xin M, Wenxin L. Genetic mechanisms of scorpion venom peptide diversification. Toxicon 2006; 47:348-55; PMID:16387337; http://dx.doi.org/10.1016/j.toxicon.2005.11.013
- Alvarez-Erviti L, Seow Y, Yin H, Betts C, Lakhal S, Wood MJA. Delivery of siRNA to the mouse brain by systemic injection of targeted exosomes. Nat Biotechnol 2011; 29:341-5; PMID:21423189; http://dx.doi.org/10.1038/nbt.1807
- Kumar P, Wu H, McBride JL, Jung KE, Kim MH, Davidson BL, Lee SK, Shankar P, Manjunath N. Transvascular delivery of small interfering RNA to the central nervous system. Nature 2007; 448:39-43; PMID:17572664; http://dx.doi.org/10.1038/nature05901
- Soroceanu L, Gillespie Y, Khazaeli MB, Sontheimer H. Use of chlorotoxin for targeting of primary brain tumors. Cancer Res 1998; 58:4871-9; PMID:9809993
- Veiseh M, Gabikian P, Bahrami SB, Veiseh O, Zhang M, Hackman RC, Ravanpay AC, Stroud MR, Kusuma Y, Hansen SJ, et al. Tumor paint: a chlorotoxin:Cy5.5 bioconjugate for intraoperative visualization of cancer foci. Cancer Res 2007; 67:6882-8; PMID:17638899; http://dx.doi.org/10.1158/0008-5472.CAN-06-3948
- Mamelak AN, Rosenfeld S, Bucholz R, Raubitschek A, Nabors LB, Fiveash JB, Shen S, Khazaeli MB, Colcher D, Liu A, et al. Phase I single-dose study of intracavitary-administered iodine-131-TM-601 in adults with recurrent high-grade glioma. J Clin Oncol 2006; 24:3644-50; PMID:16877732; http://dx.doi.org/10.1200/JCO.2005.05.4569
- Costa PM, Cardoso AL, Mendonca LS, Serani A, Custodia C, Conceicao M, Simoes S, Moreira JN, Pereira de Almeida L, Pedroso de Lima MC. Tumor-targeted chlorotoxin-coupled nanoparticles for nucleic acid delivery to glioblastoma cells: a promising system for glioblastoma treatment. Mol Ther Nucleic Acids 2013; 2:e100; PMID:23778499
- Huang R, Ke W, Han L, Li J, Liu S, Jiang C. Targeted delivery of chlorotoxin-modified DNA-loaded nanoparticles to glioma via intravenous administration. Biomaterials 2011; 32:2399-406; PMID:21185076; http://dx.doi.org/10.1016/j.biomaterials.2010.11.079
- Kievit FM, Veiseh O, Fang C, Bhattarai N, Lee D, Ellenbogen RG, Zhang M. Chlorotoxin labeled magnetic nanovectors for targeted gene delivery to glioma. ACS Nano 2010; 4:4587-94; PMID:20731441; http://dx.doi.org/10.1021/nn1008512
- Mok H, Veiseh O, Fang C, Kievit FM, Wang FY, Park JO, Zhang M. pH-Sensitive siRNA nanovector for targeted gene silencing and cytotoxic effect in cancer cells. Mol Pharm 2010; 7:1930-9; PMID:20722417; http://dx.doi.org/10.1021/mp100221h
- Veiseh O, Kievit FM, Fang C, Mu N, Jana S, Leung MC, Mok H, Ellenbogen RG, Park JO, Zhang M. Chlorotoxin bound magnetic nanovector tailored for cancer cell targeting, imaging, and siRNA delivery. Biomaterials 2010; 31:8032-42; PMID:20673683; http://dx.doi.org/10.1016/j.biomaterials.2010.07.016
- Veiseh O, Kievit FM, Gunn JW, Ratner BD, Zhang M. A ligand-mediated nanovector for targeted gene delivery and transfection in cancer cells. Biomaterials 2009; 30:649-57; PMID:18990439; http://dx.doi.org/10.1016/j.biomaterials.2008.10.003
- Kasai T, Nakamura K, Vaidyanath A, Chen L, Sekhar S, El-Ghlban S, Okada M, Mizutani A, Kudoh T, Murakami H, et al. Chlorotoxin fused to IgG-Fc inhibits glioblastoma cell motility via receptor-mediated endocytosis. J Drug Del 2012; 2012:975763; PMID:23304519; http://dx.doi.org/10.1155/2012/975763
- Locatelli E, Naddaka M, Uboldi C, Loudos G, Fragogeorgi E, Molinari V, Pucci A, Tsotakos T, Psimadas D, Ponti J, et al. Targeted delivery of silver nanoparticles and alisertib: in vitro and in vivo synergistic effect against glioblastoma. Nanomedicine 2014; 9(6):839-49; PMID:24433240
- Locatelli E, Broggi F, Ponti J, Marmorato P, Franchini F, Lena S, Franchini MC. Lipophilic silver nanoparticles and their polymeric entrapment into targeted-PEG-based micelles for the treatment of glioblastoma. Adv Healthc Mater 2012; 1:342-7; PMID:23184752; http://dx.doi.org/10.1002/adhm.201100047
- Xiang Y, Wu Q, Liang L, Wang X, Wang J, Zhang X, Pu X, Zhang Q. Chlorotoxin-modified stealth liposomes encapsulating levodopa for the targeting delivery against Parkinson's disease in the MPTP-induced mice model. J Drug Target 2012; 20:67-75; PMID:22149216; http://dx.doi.org/10.3109/1061186X.2011.595490
- Huang R, Han L, Li J, Liu S, Shao K, Kuang Y, Hu X, Wang X, Lei H, Jiang C. Chlorotoxin-modified macromolecular contrast agent for MRI tumor diagnosis. Biomaterials 2011; 32:5177-86; PMID:21531455; http://dx.doi.org/10.1016/j.biomaterials.2011.03.075
- Xiang Y, Liang L, Wang X, Wang J, Zhang X, Zhang Q. Chloride channel-mediated brain glioma targeting of chlorotoxin-modified doxorubicine-loaded liposomes. J Control Release: Off J Control Release Soc 2011; 152:402-10; PMID:21435361; http://dx.doi.org/10.1016/j.jconrel.2011.03.014
- Fang C, Veiseh O, Kievit F, Bhattarai N, Wang F, Stephen Z, Li C, Lee D, Ellenbogen RG, Zhang M. Functionalization of iron oxide magnetic nanoparticles with targeting ligands: their physicochemical properties and in vivo behavior. Nanomedicine 2010; 5:1357-69; PMID:21128719; http://dx.doi.org/10.2217/nnm.10.55
- Lee MJ, Veiseh O, Bhattarai N, Sun C, Hansen SJ, Ditzler S, Knoblaugh S, Lee D, Ellenbogen R, Zhang M, et al. Rapid pharmacokinetic and biodistribution studies using cholorotoxin-conjugated iron oxide nanoparticles: a novel non-radioactive method. PLoS One 2010; 5:e9536; PMID:AMBIGUOUS
- Meng XX, Wan JQ, Jing M, Zhao SG, Cai W, Liu EZ. Specific targeting of gliomas with multifunctional superparamagnetic iron oxide nanoparticle optical and magnetic resonance imaging contrast agents. Acta Pharmacol Sinic 2007; 28:2019-26; PMID:18031618; http://dx.doi.org/10.1111/j.1745-7254.2007.00661.x
- Sun C, Du K, Fang C, Bhattarai N, Veiseh O, Kievit F, Stephen Z, Lee D, Ellenbogen RG, Ratner B, et al. PEG-mediated synthesis of highly dispersive multifunctional superparamagnetic nanoparticles: their physicochemical properties and function in vivo. ACS Nano 2010; 4:2402-10; PMID:20232826; http://dx.doi.org/10.1021/nn100190v
- Sun C, Fang C, Stephen Z, Veiseh O, Hansen S, Lee D, Ellenbogen RG, Olson J, Zhang M. Tumor-targeted drug delivery and MRI contrast enhancement by chlorotoxin-conjugated iron oxide nanoparticles. Nanomedicine 2008; 3:495-505; PMID:18694312; http://dx.doi.org/10.2217/17435889.3.4.495
- Sun C, Veiseh O, Gunn J, Fang C, Hansen S, Lee D, Sze R, Ellenbogen RG, Olson J, Zhang M. In vivo MRI detection of gliomas by chlorotoxin-conjugated superparamagnetic nanoprobes. Small 2008; 4:372-9; PMID:18232053; http://dx.doi.org/10.1002/smll.200700784
- Veiseh O, Gunn JW, Kievit FM, Sun C, Fang C, Lee JS, Zhang M. Inhibition of tumor-cell invasion with chlorotoxin-bound superparamagnetic nanoparticles. Small 2009; 5:256-64; PMID:19089837; http://dx.doi.org/10.1002/smll.200800646
- Veiseh O, Sun C, Fang C, Bhattarai N, Gunn J, Kievit F, Du K, Pullar B, Lee D, Ellenbogen RG, et al. Specific targeting of brain tumors with an optical/magnetic resonance imaging nanoprobe across the blood-brain barrier. Cancer Res 2009; 69:6200-7; PMID:19638572; http://dx.doi.org/10.1158/0008-5472.CAN-09-1157
- Wan J, Meng X, Liu E, Chen K. Incorporation of magnetite nanoparticle clusters in fluorescent silica nanoparticles for high-performance brain tumor delineation. Nanotechnology 2010; 21:235104; PMID:20472942; http://dx.doi.org/10.1088/0957-4484/21/23/235104
- Orndorff RL, Rosenthal SJ. Neurotoxin quantum dot conjugates detect endogenous targets expressed in live cancer cells. Nano Letters 2009; 9:2589-99; PMID:19507837; http://dx.doi.org/10.1021/nl900789e
- Safdar S, Payne CA, Tu NH, Taite LJ. Targeted nitric oxide delivery preferentially induces glioma cell chemosensitivity via altered p53 and O(6) -methylguanine-DNA methyltransferase activity. Biotechnol Bioeng 2013; 110:1211-20; PMID:23125026; http://dx.doi.org/10.1002/bit.24775
- Zhan C, Yan Z, Xie C, Lu W. Loop 2 of Ophiophagus hannah toxin b binds with neuronal nicotinic acetylcholine receptors and enhances intracranial drug delivery. Mol Pharm 2010; 7:1940-7; PMID:20964364; http://dx.doi.org/10.1021/mp100238j
- Maus L, Dick O, Bading H, Spatz JP, Fiammengo R. Conjugation of peptides to the passivation shell of gold nanoparticles for targeting of cell-surface receptors. ACS Nano 2010; 4:6617-28; PMID:20939520; http://dx.doi.org/10.1021/nn101867w
- Francis JW, Figueiredo D, vanderSpek JC, Ayala LM, Kim YS, Remington MP, Young PJ, Lorson CL, Ikebe S, Fishman PS, et al. A survival motor neuron:tetanus toxin fragment C fusion protein for the targeted delivery of SMN protein to neurons. Brain Res 2004; 995:84-96; PMID:14644474; http://dx.doi.org/10.1016/j.brainres.2003.09.063
- Ciriza J, Moreno-Igoa M, Calvo AC, Yague G, Palacio J, Miana-Mena FJ, Munoz MJ, Zaragoza P, Brulet P, Osta R. A genetic fusion GDNF-C fragment of tetanus toxin prolongs survival in a symptomatic mouse ALS model. Restor Neurol Neurosci 2008; 26:459-65; PMID:19096133
- Chian RJ, Li J, Ay I, Celia SA, Kashi BB, Tamrazian E, Matthews JC, Bronson RT, Rossomando A, Pepinsky RB, et al. IGF-1:tetanus toxin fragment C fusion protein improves delivery of IGF-1 to spinal cord but fails to prolong survival of ALS mice. Brain Res 2009; 1287:1-19; PMID:19563785; http://dx.doi.org/10.1016/j.brainres.2009.06.066
- Figueiredo DM, Hallewell RA, Chen LL, Fairweather NF, Dougan G, Savitt JM, Parks DA, Fishman PS. Delivery of recombinant tetanus-superoxide dismutase proteins to central nervous system neurons by retrograde axonal transport. Exp Neurol 1997; 145:546-54; PMID:9217090; http://dx.doi.org/10.1006/exnr.1997.6490
- Favretto ME, Wallbrecher R, Schmidt S, van de Putte R, Brock R. Glycosaminoglycans in the cellular uptake of drug delivery vectors - Bystanders or active players? J Control Release: Off J Control Release Soc 2014; 180C:81-90; http://dx.doi.org/10.1016/j.jconrel.2014.02.011
- Ng CP, Goodman TT, Park IK, Pun SH. Bio-mimetic surface engineering of plasmid-loaded nanoparticles for active intracellular trafficking by actin comet-tail motility. Biomaterials 2009; 30:951-8; PMID:19046764; http://dx.doi.org/10.1016/j.biomaterials.2008.10.059
- Niu Y, Yu M, Hartono SB, Yang J, Xu H, Zhang H, Zhang J, Zou J, Dexter A, Gu W, et al. Nanoparticles mimicking viral surface topography for enhanced cellular delivery. Adv Mater 2013; 25:6233-7; PMID:23946251; http://dx.doi.org/10.1002/adma.201302737
- Srikanth M, Kessler JA. Nanotechnology-novel therapeutics for CNS disorders. Nat Rev Neurol 2012; 8:307-18; PMID:22526003; http://dx.doi.org/10.1038/nrneurol.2012.76
- Schneider-Schaulies J, Meulen V, Schneider-Schaulies S. Measles infection of the central nervous system. J Neurovirol 2003; 9:247-52; PMID:12707855; http://dx.doi.org/10.1080/13550280390193993
- Compton T. Receptors and immune sensors: the complex entry path of human cytomegalovirus. Trends Cell Biol 2004; 14:5-8.
- Li Q, Ali MA, Cohen JI. Insulin degrading enzyme is a cellular receptor mediating varicella-zoster virus infection and cell-to-cell spread. Cell 2006; 127:305-16; PMID:17055432; http://dx.doi.org/10.1016/j.cell.2006.08.046
- Chen JJ, Zhu Z, Gershon AA, Gershon MD. Mannose 6-phosphate receptor dependence of varicella zoster virus infection in vitro and in the epidermis during varicella and zoster. Cell 2004; 119:915-26; PMID:15620351; http://dx.doi.org/10.1016/j.cell.2004.11.007
- Dunfee R, Thomas ER, Gorry PR, Wang J, Ancuta P, Gabuzda D. Mechanisms of HIV-1 neurotropism. Curr HIV Res 2006; 4:267-78; PMID:16842080; http://dx.doi.org/10.2174/157016206777709500
- Wang T, Town T, Alexopoulou L, Anderson JF, Fikrig E, Flavell RA. Toll-like receptor 3 mediates West Nile virus entry into the brain causing lethal encephalitis. Nat Med 2004; 10:1366-73; PMID:15558055; http://dx.doi.org/10.1038/nm1140
- Chu JJ, Ng ML. Interaction of West Nile virus with alpha v beta 3 integrin mediates virus entry into cells. J Biol Chem 2004; 279:54533-41; PMID:15475343; http://dx.doi.org/10.1074/jbc.M410208200
- Racaniello VR. One hundred years of poliovirus pathogenesis. Virology 2006; 344:9-16; PMID:AMBIGUOUS
- Chung JW, Hong SJ, Kim KJ, Goti D, Stins MF, Shin S, Dawson VL, Dawson TM, Kim KS. 37-kDa laminin receptor precursor modulates cytotoxic necrotizing factor 1-mediated RhoA activation and bacterial uptake. J Biol Chem 2003; 278:16857-62; PMID:12615923; http://dx.doi.org/10.1074/jbc.M301028200
- Thepparit C, Smith DR. Serotype-specific entry of dengue virus into liver cells: identification of the 37-kilodalton/67-kilodalton high-affinity laminin receptor as a dengue virus serotype 1 receptor. J Virol 2004; 78:12647-56; PMID:15507651; http://dx.doi.org/10.1128/JVI.78.22.12647-12656.2004
- Bogachek MV, Protopopova EV, Loktev VB, Zaitsev BN, Favre M, Sekatskii SK, Dietler G. Immunochemical and single molecule force spectroscopy studies of specific interaction between the laminin binding protein and the West Nile virus surface glycoprotein E domain II. J Mol Recognit 2008; 21:55-62; PMID:18061925; http://dx.doi.org/10.1002/jmr.866
- Protopopova EV, Sorokin AV, Konovalova SN, Kachko AV, Netesov SV, Loktev VB. Human laminin binding protein as a cell receptor for the tick-borne encephalitis virus. Zbl Bakt 1999; 289:632-8; PMID:10215633
- Hurtado A, Tseng JC, Boivin C, Levin B, Yee H, Pampeno C, Meruelo D. Identification of amino acids of Sindbis virus E2 protein involved in targeting tumor metastases in vivo. Mol Ther: J Am Soc Gene Ther 2005; 12:813-23; PMID:16109508; http://dx.doi.org/10.1016/j.ymthe.2005.06.476
- Wang KS, Kuhn RJ, Strauss EG, Ou S, Strauss JH. High-affinity laminin receptor is a receptor for Sindbis virus in mammalian cells. J Virol 1992; 66:4992-5001; PMID:1385835
- Thongtan T, Wikan N, Wintachai P, Rattanarungsan C, Srisomsap C, Cheepsunthorn P, Smith DR. Characterization of putative Japanese encephalitis virus receptor molecules on microglial cells. J Med Virol 2012; 84:615-23; PMID:22337301; http://dx.doi.org/10.1002/jmv.23248
- Zhan C, Yan Z, Xie C, Lu W. Loop 2 of Ophiophagus hannah toxin b binds with neuronal nicotinic acetylcholine receptors and enhances intracranial drug delivery. Mol Pharm 2010; 7:1940-7; PMID:20964364; http://dx.doi.org/10.1021/mp100238j
- Marcon F, Leblanc M, Vetter I, Lewis RJ, Escoubas P, Nicholson GM. Pharmacological characterization of alpha-elapitoxin-Al2a from the venom of the Australian pygmy copperhead (Austrelaps labialis): an atypical long-chain alpha-neurotoxin with only weak affinity for alpha7 nicotinic receptors. Biochem Pharmacol 2012; 84:851-63; PMID:22771828; http://dx.doi.org/10.1016/j.bcp.2012.06.024
- Kauferstein S, Kendel Y, Nicke A, Coronas F, Possani L, Favreau P, Križaj I, Wunder C, Kauert G, Mebs D. New conopeptides of the D-superfamily selectively inhibiting neuronal nicotinic acetylcholine receptors. Toxicon: Off J Int Soc Toxinol 2009; 54:295-301; PMID:19393680; http://dx.doi.org/10.1016/j.toxicon.2009.04.016
- Whiteaker P, Christensen S, Yoshikami D, Dowell C, Watkins M, Gulyas J, Rivier J, Olivera BM, McIntosh JM. Discovery, synthesis, and structure activity of a highly selective α7 nicotinic acetylcholine receptor antagonist. Biochemistry 2007; 46:6628-38; PMID:17497892; http://dx.doi.org/10.1021/bi7004202
- Dutertre S, Ulens C, Büttner R, Fish A, van Elk R, Kendel Y, Hopping G, Alewood PF, Schroeder C, Nicke A. AChBP-targeted α-conotoxin correlates distinct binding orientations with nAChR subtype selectivity. EMBO J 2007; 26:3858-67; PMID:17660751; http://dx.doi.org/10.1038/sj.emboj.7601785
- Inserra MC, Kompella SN, Vetter I, Brust A, Daly NL, Cuny H, Craik DJ, Alewood PF, Adams DJ, Lewis RJ. Isolation and characterization of α-conotoxin LsIA with potent activity at nicotinic acetylcholine receptors. Biochem Pharmacol 2013; 86:791-9; PMID:23924607; http://dx.doi.org/10.1016/j.bcp.2013.07.016
- Yu R, Craik DJ, Kaas Q. Blockade of neuronal α7-nAChR by α-conotoxin ImI explained by computational scanning and energy calculations. PLoS Computat Biol 2011; 7:e1002011; PMID:AMBIGUOUS
- Liu Y, Guo Y, An S, Kuang Y, He X, Ma H, Li J, Lv J, Zhang N, Jiang C. Targeting caspase-3 as dual therapeutic benefits by RNAi facilitating brain-targeted nanoparticles in a rat model of Parkinson's disease. PloS One 2013; 8:e62905;
- Alvarez-Erviti L, Seow Y, Yin H, Betts C, Lakhal S, Wood MJ. Delivery of siRNA to the mouse brain by systemic injection of targeted exosomes. Nat Biotechnol 2011; 29:341-5; PMID:21423189; http://dx.doi.org/10.1038/nbt.1807
- Hwang do W, Son S, Jang J, Youn H, Lee S, Lee D, Lee YS, Jeong JM, Kim WJ, Lee DS. A brain-targeted rabies virus glycoprotein-disulfide linked PEI nanocarrier for delivery of neurogenic microRNA. Biomaterials 2011; 32:4968-75; PMID:21489620; http://dx.doi.org/10.1016/j.biomaterials.2011.03.047
- Fu A, Wang Y, Zhan L, Zhou R. Targeted delivery of proteins into the central nervous system mediated by rabies virus glycoprotein-derived peptide. Pharmaceut Res 2012; 29:1562-9; PMID:22231987; http://dx.doi.org/10.1007/s11095-012-0667-y
- Kim JY, Choi WI, Kim YH, Tae G. Brain-targeted delivery of protein using chitosan- and RVG peptide-conjugated, pluronic-based nano-carrier. Biomaterials 2013; 34:1170-8; PMID:23122677; http://dx.doi.org/10.1016/j.biomaterials.2012.09.047
- Chen W, Zhan C, Gu B, Meng Q, Wang H, Lu W, Hou H. Targeted brain delivery of itraconazole via RVG29 anchored nanoparticles. J Drug Target 2011; 19:228-34; PMID:20540685; http://dx.doi.org/10.3109/1061186X.2010.492523
- Liu Y, Huang R, Han L, Ke W, Shao K, Ye L, Lou J, Jiang C. Brain-targeting gene delivery and cellular internalization mechanisms for modified rabies virus glycoprotein RVG29 nanoparticles. Biomaterials 2009; 30:4195-202; PMID:19467700; http://dx.doi.org/10.1016/j.biomaterials.2009.02.051
- Andreu A, Fairweather N, Miller AD. Clostridium neurotoxin fragments as potential targeting moieties for liposomal gene delivery to the CNS. Chembiochem: Eur J Chem Biol 2008; 9:219-31; PMID:18076008; http://dx.doi.org/10.1002/cbic.200700277
- Knight A, Carvajal J, Schneider H, Coutelle C, Chamberlain S, Fairweather N. Non-viral neuronal gene delivery mediated by the HC fragment of tetanus toxin. Eur J Biochem/FEBS 1999; 259:762-9; PMID:10092862
- Moreno-Igoa M, Calvo AC, Ciriza J, Munoz MJ, Zaragoza P, Osta R. Non-viral gene delivery of the GDNF, either alone or fused to the C-fragment of tetanus toxin protein, prolongs survival in a mouse ALS model. Restor Neurol Neurosci 2012; 30:69-80; PMID:22124037
- Oliveira H, Fernandez R, Pires LR, Martins MC, Simoes S, Barbosa MA, Pego AP. Targeted gene delivery into peripheral sensorial neurons mediated by self-assembled vectors composed of poly(ethylene imine) and tetanus toxin fragment c. J Control Release: Off J Control Release Soc 2010; 143:350-8; PMID:20093157; http://dx.doi.org/10.1016/j.jconrel.2010.01.018
- Benn SC, Ay I, Bastia E, Chian RJ, Celia SA, Pepinsky RB, Fishman PS, Brown RH, Jr., Francis JW. Tetanus toxin fragment C fusion facilitates protein delivery to CNS neurons from cerebrospinal fluid in mice. J Neurochem 2005; 95:1118-31; PMID:16271047; http://dx.doi.org/10.1111/j.1471-4159.2005.03459.x
- Carlton E, Teng Q, Federici T, Yang J, Riley J, Boulis NM. Fusion of the tetanus toxin C fragment binding domain and Bcl-xL for protection of peripheral nerve neurons. Neurosurgery 2008; 63:1175-82; http://dx.doi.org/10.1227/01.NEU.0000334415.45003.EA
- Francis JW, Hosler BA, Brown RH, Jr., Fishman PS. CuZn superoxide dismutase (SOD-1):tetanus toxin fragment C hybrid protein for targeted delivery of SOD-1 to neuronal cells. J Biol Chem 1995; 270:15434-42; PMID:7797532; http://dx.doi.org/10.1074/jbc.270.25.15434
- Gramlich PA, Remington MP, Amin J, Betenbaugh MJ, Fishman PS. Tat-tetanus toxin fragment C: a novel protein delivery vector and its use with photochemical internalization. J Drug Target 2013; 21:662-74; PMID:23697582; http://dx.doi.org/10.3109/1061186X.2013.796954
- Larsen KE, Benn SC, Ay I, Chian RJ, Celia SA, Remington MP, Bejarano M, Liu M, Ross J, Carmillo P, et al. A glial cell line-derived neurotrophic factor (GDNF): tetanus toxin fragment C protein conjugate improves delivery of GDNF to spinal cord motor neurons in mice. Brain Res 2006; 1120:1-12; PMID:17020749; http://dx.doi.org/10.1016/j.brainres.2006.08.079
- Li J, Chian RJ, Ay I, Kashi BB, Celia SA, Tamrazian E, Pepinsky RB, Fishman PS, Brown RH, Jr., Francis JW. Insect GDNF:TTC fusion protein improves delivery of GDNF to mouse CNS. Biochem Biophys Res Commun 2009; 390:947-51; PMID:19852934; http://dx.doi.org/10.1016/j.bbrc.2009.10.083
- Townsend SA, Evrony GD, Gu FX, Schulz MP, Brown RH, Jr., Langer R. Tetanus toxin C fragment-conjugated nanoparticles for targeted drug delivery to neurons. Biomaterials 2007; 28:5176-84; PMID:17854886; http://dx.doi.org/10.1016/j.biomaterials.2007.08.011