Abstract
We have developed an assay for single strand DNA and RNA detection which is based on novel pyrene−perylene FRET pairs attached to short LNA/DNA probes. The assay is based on ratiometric emission upon binding of target DNA/RNA by three combinations of fluorescent LNA/DNA reporter strands. Specific geometry of the pyrene fluorophore attached to the 2′-amino group of 2′-amino-LNA in position 4 allows for the first time to efficiently utilize dipole−dipole orientation parameter for sensing of single-nucleotide polymorphisms (SNPs) in nucleic acid targets by FRET. Using novel probes, SNP detection is achieved with advantages of large Stokes shift (115 nm), high fluorescence quantum yields and low limit of target detection values (< 5 nM). Rapid and accurate genotyping of highly polymorphic HIV Pol cDNA and RNA fragments performed herein proves the possibility for broad application of the novel pyrene−perylene FRET pairs, e.g., in imaging and clinical diagnostics.
Introduction
As detailed single-nucleotide polymorphism (SNP) maps become more accessible, there is a growing understanding that rapid and efficient detection methods are required to bring SNP analysis into mainstream use in molecular diagnostics laboratories. Through constant developing of SNPs under the selectional pressure of the global use of antiviral therapy, RNA viruses such as HIV-1 have evolved complex mechanisms for resistance to treatment with drugs.Citation1,Citation2 In modern complex anti-viral HIV-1/AIDS therapy, SNPs causing drug resistance to protease inhibitors (PIs) are some of the most important, that demand a change in treatment when the resistance is developed.Citation3,Citation4 However, current SNP genotyping is a time-consuming and rather expensive procedure typically performed using sequencing or microarray techniques.Citation5
Förster resonance energy transfer (FRET) is one of the major fluorescence effects applied in nucleic acid biosensing which has been studied thoroughly.Citation6-Citation19 Being a physical process whereby the excited state energy of a donor molecule can be transferred to a neighboring acceptor fluorophore in the ground state, FRET can take place whenever the two fluorophores with sufficient spectral overlap between the donor fluorescence and acceptor absorbance are in close proximity (distance typically below 7 nm), and importantly, provided favorable donor−acceptor dipole−dipole orientation. FRET can be monitored quantitatively and in real time (e.g., real-time PCR),Citation20-Citation26 with additional possibilities for multiplexing and growing applications in imaging in vivo.Citation10,Citation13 Several FRET pairs have already been applied in SNP genomics in different assays, which have their own advantages and drawbacks (e.g., Taqman probes, molecular beacons, dye-labeled oligonucleotide ligation and template-directed dye-terminator incorporation).Citation27 However using mainly dye−quencher design of FRET probes, none of these assays explore an important dipole−dipole orientation factor, which is known to influence FRET significantly and therefore might be a useful tool in creating nucleic acid probes for clinical diagnostics and bioimaging.Citation28
Recently, sensitive polyaromatic hydrocarbon (PAH) dyes attached to locked nucleic acids (2′-amino- and isomeric 2′-α-L-amino-LNA) have been proven to be effective for SNP analysis of highly polymorphic model and natural targets.Citation29-Citation31 This was done using short LNA/DNA probes and terminal excimer interactions between two pyrene or (phenylethynyl)pyrene residues. High sensitivity of the PAH-LNA monomers to minor changes in local microenvironments such as SNPs is mainly caused by precise positioning of PAHs within the double-stranded nucleic acid complexes provided by attachment to a rigid bicyclic LNA skeleton. High binding affinity and selectivity to DNA/RNA targets and sensitivity to SNPs was however often accompanied by low quantum yield of the pyrene derivatives substituted at position 1 (monomer MCitation1, ).Citation30
Figure 1. Chemical structures of modified PAH-LNA monomers MCitation1−MCitation4 used in this study and homogeneous fluorescence assay for SNP detection using MCitation1−MCitation4 within terminally labeled FRET probes.
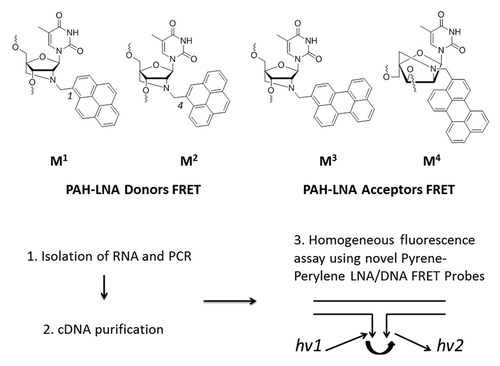
Herein, we aimed at improving fluorescence properties of the pyrene attached to 2′-amino-LNA by alternating the position of substitution in the pyrene core from 1 to 4, since this modification has already showed promising results in labeling of nucleic acids (monomer M2, ).Citation31-Citation34 We demonstrate that this modification significantly improved photophysical and spectral characteristics of the pyrene with a strong effect on its interactions with the commonly used PAH acceptor FRET perylene (monomers M3 and MCitation4, ).Citation35,Citation36 We rationalized these results by molecular modeling studies and furthermore utilized the novel FRET pairs M2/M3−MCitation4 within short LNA/DNA probes for SNP sensing in cDNA fragments obtained from patients currently receiving anti-HIV-1/AIDS therapy ().
Results
Synthesis of monomer M2 was performed starting from 2′-amino-LNA nucleoside 1Citation37,Citation38 and commercially available pyrene-4-carbaldehyde under reductive amination conditionsCitation39 followed by phosphitylationCitation40 of 2 providing phosphoramidite 3 in 79% overall yield (Scheme 1). Previously investigated (pyrene-1-yl)methyl 2′-amino-LNA (monomer MCitation1) was incorporated into oligonucleotides as a reference for the new FRET donor MCitation2,Citation41 monomers M3 and MCitation4 were prepared as previously described.Citation42,Citation43
Scheme 1. Preparation of modified monomers MCitation1−MCitation4. Reagents, conditions (and yields): (i) pyrene-4-carbaldehyde, NaBH(OAc)3, 1,2-dichloroethane, rt (87%); (ii) NC(CH2)2OP(Cl)N(iPr)2, DIPEA, CH2Cl2, rt (91%); (iii) DNA synthesizer. DMT = 4,4´-dimethoxytrityl.
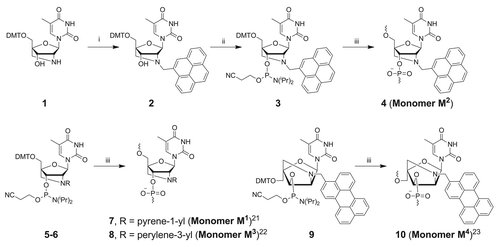
For initial evaluation of pyrene−perylene FRET and SNP diagnostic potential, monomers MCitation1–MCitation4 were incorporated in a model dual-probe FRET system ON1−ON4 and covalently linked double-labeled analogs ON5−ON8 adopted from previous research on PAH-LNA monomers ().Citation29,Citation30 The model FRET probes were prepared using automated phosphoramidite DNA synthesis and first characterized by their melting temperatures with complementary and mismatched DNA/RNA targets (Tm; Tables S1‒S6). Generally high melting temperatures of the short LNA/DNA probes ON1−ON4 (37−63°C) were significantly decreased by mismatches in DNA and RNA targets located at the four neighboring positions to the modified monomers, while a single mismatch opposite to monomers MCitation1−MCitation4 led to small Tm changes in the complexes containing monomer M2 displaying ΔTm values of -0.5°C to +3.0°C (for example, Tm values of ON2 upon binding DNA containing mismatch T→C at neighboring position 6 and mismatch A→C opposite to monomer M2 were 19.0°C and 40.0°C, respectively; Table S4). Having compared Tm data for ON1‒ON2 and corresponding LNA/DNA reference Citation5′-(CAL CCA ACTL), we observed that the incorporated fluorophores had a large impact on mismatch discrimination in the two neighboring positions 6−7, although the duplexes were less destabilized and even stabilized for all the probes when a mismatch was opposite to the 3′-end nucleotide (Tables S3‒S4). For example, Tm decrease upon binding RNA containing mismatch G→C at neighboring position 7 by ON1, ON2 and reference LNA/DNA compared with fully complementary complexes (ΔTm values) were -17.5°C, -19.5°C and ± 0.0°C, respectively. However, ΔTm values were -0.5°C, -0.5°C and +4.0°C for ON1, ON2 and reference LNA/DNA, respectively, upon binding RNA target having A→U mismatch at position 8 (Tables S3‒S4). As expected, all the longer hybrids ON5−ON8 exhibited melting temperatures that were higher than 70°C and were generally less affected by mismatches compared with the dual probes ON1−ON4 (; e.g., Tm 71.0°C, 61°C and 70°C for duplexes of ON7 with cDNA, DNA containing mismatch T→C at position 6 and mismatch A→C opposite to monomer MCitation1; Table S6). The major differences between the Tm discrimination of the mismatched nucleotides in central and peripheral positions to monomers MCitation1−MCitation4 can be attributed to several factors. First, owing to lower thermodynamic stability short fluorescent oligonucleotides generally display higher sensitivity of hybridization toward mismatched DNA/RNA targets than their longer analogs.Citation29-Citation33 Second, flexibility of the terminally attached PAHs might lead to better positioning of the bulky fluorophores within the dual-probe complementary duplexes with respect to those containing single-base mismatches leading to strongly decreased Tm values for the latter complexes. Third, increased stability of the duplexes containing mismatches opposite to modifications within the dual-probe format might result from proximity of the fluorophores to the end of the duplex, where fraying of the doubles-stranded complex diminishes the impact of mismatches relative to those located at internal positions.
Table 1. Sequences and MALDI-MS of fluorescent oligonucleotides prepared in this study[a]
Figure 2. Representation of fluorescent probes prepared in this study (A), thermal denaturation curves (B), and steady-state fluorescence emission spectra (C) of single-stranded model probes ON2, ON4 and their complexes with cDNA and RNA obtained in a medium salt phosphate buffer ([Na+] = 110 mM) using absorbance at 260 nm (B), excitation wavelength 340 nm (C) and 1.0 µM concentration of oligonucleotides.
![Figure 2. Representation of fluorescent probes prepared in this study (A), thermal denaturation curves (B), and steady-state fluorescence emission spectra (C) of single-stranded model probes ON2, ON4 and their complexes with cDNA and RNA obtained in a medium salt phosphate buffer ([Na+] = 110 mM) using absorbance at 260 nm (B), excitation wavelength 340 nm (C) and 1.0 µM concentration of oligonucleotides.](/cms/asset/5a094644-1f33-471b-a1f1-63af2c6cc4a3/kdpx_a_10925903_f0003.gif)
Excitation was performed at 340 nm for all the FRET probes, since the monomers MCitation1 and M2 revealed similar position and structure of their visible absorbance, excitation and fluorescence bands (). Photophysical and spectral properties of the model systems indicated superior quantum yields Фf 0.15−0.18 of M2 compared with Фf 0.06−0.07 of MCitation1, although both FRET donors were quenched within single strands (ON1−ON2: Фf 0.04−0.05; ; ). Fluorescence light-up upon hybridization points at the positioning of PAH fluorophores in nonpolar minor groove of the duplexes. Minor interactions of the fluorophores with the nucleobases within the complementary complexes were also confirmed by low sensitivity of the fluorophore’s absorbance to the elevated temperature (Fig. S3). Moreover bright fluorescence of the complexes containing monomers M2/M3−MCitation4 contradicts intercalation typically leading to quenched PAH fluorescence due to interaction with nucleobases.Citation32
Table 2. Spectral properties of selected model fluorescence probes and their duplexes with cDNA/RNA targets [a]
The quantum yields and, hence, fluorescence brightness values FB of double-labeled probes ON5−ON8 were very high (Фf 0.72−1.00 and FB up to 52.0), however without sensitivity of the fluorescence to single-base mismatches (Fig. S1). The mismatches were in turn strongly discriminated by dual-probes ON2−ON4 containing M2/M3−MCitation4 FRET pairs (discrimination factor DF up to 16.7 using the ratio between fluorescence intensity of acceptor to donor; Fig. S1). Importantly, quantum yields of the donor FRET in absence of acceptor and extinction coefficient of the corresponding acceptor in absence of donor evaluated prior to FRET measurements were similar for fully complementary and singly-mismatched complexes (Fig. S3). High FRET efficiency values of the fully complementary complexes (E up to 1.00) were furthermore decreased in presence of a single mismatch opposite to the donor FRET M2, but not opposite to acceptors M3−MCitation4 (E 0.22−0.45 and 0.89−0.95, respectively; Table S7). Finally, mismatch discrimination in RNA targets was as efficient as in DNA, although with slight alterations of E and discrimination factor values, which can be attributed to the structural differences between the complexes with DNA and RNA.
Additional information about FRET can be obtained from calculation of donor−acceptor spectral overlap integral J and Förster distance R0 (the donor−acceptor distance at which E = 50%), and from analysis of distance dependence of E values ().Citation28,Citation44,Citation45 The calculations were performed assuming dipole‒dipole orientation factor χ2 = 2/3 due to emission dipole of the donor and the absorption dipole of the acceptor randomized by segmental motions of the nucleic acid components, while the average dipole orientations were favorable for FRET within the complementary complexes.Citation28 First, novel donor FRET M2 displayed higher J compared with MCitation1, although the R0 values and distance dependence of E were similar for the two monomers (J ~5.2 × 10−14 cmCitation6 mmol−1 vs. 3.2 × 10−14 cmCitation6 mmol−1 for M2 and MCitation1, respectively; R0 ~20 Å; see Materials and Methods for calculation details). Being compared with previously studied Cy3−Cy5 FRET pair terminally attached to series of DNA duplexes, overlap integral J was lower for the pairs M2/M3‒MCitation4, which is most likely caused by distinctive spectral properties of the PAH dyes (J ~7.2 × 10−13 cmCitation6 mmol−1 for Cy3−Cy5).Citation45
Figure 3. Dependence of FRET efficiency E on distance between donor and acceptor FRET. Probes ON1+ON4 and ON2+ON4 (FRET pairs MCitation1/MCitation4 and M2/MCitation4) are shown as blue and red lines, respectively. The data presented was obtained assuming E = 1.0 for duplexes with target DNA/RNA at n = 0.
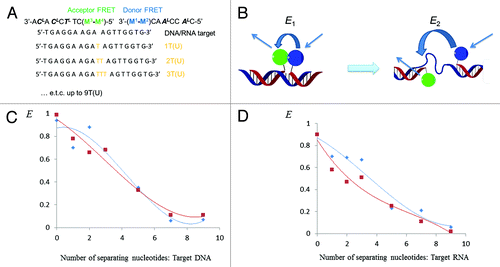
Second, dependence of the FRET efficiency values E on the distance between the donor and acceptor FRET was studied using a series of DNA/RNA targets complementary to ON1−ON4 and containing increasing number of separating nucleotides dTn (rUn) between the modified monomers (n = 0−9; ).Citation44 Taking length of one nucleotide unit 3.5 Å,Citation46 the single-stranded gap model allowed measurements of FRET efficiency when the distance between the modified monomers upon hybridization of ON1−ON4 to the targets varied from zero to 31.5 Å (). This assay format was applied because of small Förster distance of the pyrene‒perylene FRET pair,Citation44 although the single-stranded gap model could not disclose orientation dependence of FRET pairs M2/M3‒MCitation4 within long double-stranded complexes.Citation45 Having annealed the FRET probes to the series of DNA/RNA targets and having measured the FRET efficiency values E of the resulting complexes as described above, the resulting E values were plotted against the distance between the monomers MCitation1−M2 and M3−MCitation4 (). Importantly, the FRET process in the new pairs M2/M3−MCitation4 was inversely proportional to the sixth power of the distance between donor and acceptor, which was of stronger magnitude for DNA targets compared with RNA and in all the cases was in agreement with classic FRET theory ().Citation28
Molecular models of the complementary and singly-mismatched complexes containing MCitation1−MCitation4 showed distance changes r = 2−4 Å between the donors and acceptors FRET in presence of mismatch, which is too small in order to decrease the FRET efficiency as indicated by the E−r plots ( and ). Moreover, molecular modeling showed closer positioning of the M2 within the minor groove compared with isomeric monomer MCitation1, and revealed changes in pyrene orientation in the presence of a mismatch opposite to the pyrene-4-yl monomer M2 ( compared with ). This is to the best of our knowledge the first example of dipole−dipole orientation change between donor and acceptor FRET observed in presence of a single-nucleotide mismatch in a nucleic acid target.Citation27 Notably, improvement of pyrene’s properties as donor FRET was achieved by changing position of the substitution from 1 to 4, which underlines a very strong dependence of the pyrene’s spectral properties on the fluorophore’s symmetry and adoption within the nucleic acid complexes. Finally, molecular modeling indicated partial positioning of perylene within minor groove of the duplexes accompanied by exposure of the fluorophore into the medium, in all cases with minor interactions between perylene and nucleobases (). Such positioning of acceptor FRET is most likely one of the most important factors for obtaining high fluorescence quantum yields of the prepared probes upon binding DNA/RNA targets, since fluorescence response of PAH monitored during the assay is not quenched by the surrounding nucleobases.Citation28
Figure 4. Low-energy structures of complementary and single-mismatched duplexes containing FRET pairs MCitation1/MCitation4 and M2/MCitation4: A) MCitation1/MCitation4 pair within fully matched complex ON1:ON4:DNA; B) MCitation1/MCitation4 pair within complex containing mismatch opposite to monomer MCitation1 (ON1:ON4:dmA(8)T); C) M2/MCitation4 pair within fully matched complex ON2:ON4:DNA; D) M2/MCitation4 pair within complex containing mismatch opposite to monomer M2 (ON2:ON4:dmA(8)T).
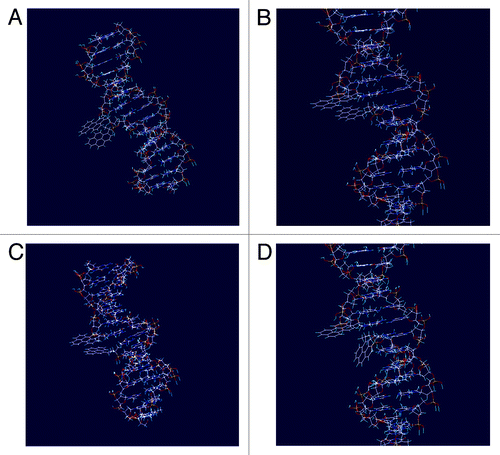
Detection of SNP in PCR fragments is a promising method for rapid genotyping in absence of sequencing and microarray equipment.Citation25 In this study we aimed to develop a homogeneous fluorescence assay for SNP analysis in the clinically important HIV-1 gene fragment encoding protease (HIV Pol) using novel M2/M3−MCitation4 FRET pairs (). We furthermore aimed at creating a fluorescence assay which could be further applied in e.g., real-time imaging of viral RNA/cDNA and quantative multiplex PCR assays. Initially, a panel of 200 plasma samples obtained from patients currently receiving complex HIV-1/AIDS antiviral therapy at the Russian Federal AIDS Centre was analyzed. We subsequently isolated the total RNA from the plasma samples followed by RT-PCR and Nested PCR amplification rounds using specific primers for the 570 nucleotide Pol fragment.Citation29 The resulting PCR products were purified and sequenced (; Fig. S4). Having analyzed the resulting sequences, we concluded that within region 2332–2354 mutation G2340A causing resistance of HIV-1 to protease inhibitor (PI) virasept (nelfinavire) is most often accompanied by one or two polymorphisms located three to four nucleotides apart from position 2340. Moreover, the region 2332–2354 often contains mutation G2346A causing resistance of HIV-1 to all PIs available to date (Table S8).Citation47 We obtained model targets (cDNA and RNA variants) corresponding to the sequenced Pol regions 2332–2354 from commercial suppliers. In order to evaluate selectivity of target binding, highly polymorphic targets not observed in screening were obtained as well. Finally, we isolated six 570 nt long Pol cDNA and characterized them by sequencing (Fig. Five and the Supplementary Information).
Figure 5. Detection of HIV PR cDNA and RNA fragments. Ss1−ss3 = ON10+ON14, ON9+ON12 and ON9+ON11, respectively. The data are presented according to homogeneous fluorescence genotyping assay described in Patients, Material and Methods section. Target abbreviations: Wt, wild type; Wt + pol, wild-type at positions 2340 and 2346 containing polymorphic mutations; G2340A (G2346A) + pol, mutation G→A at position 2340 (2346) and additional polymorphic mutations; r, purine as low-complexity or repetitive element.Citation1 For complete sequencing data of the cDNA fragments, see the Supplemental Material.
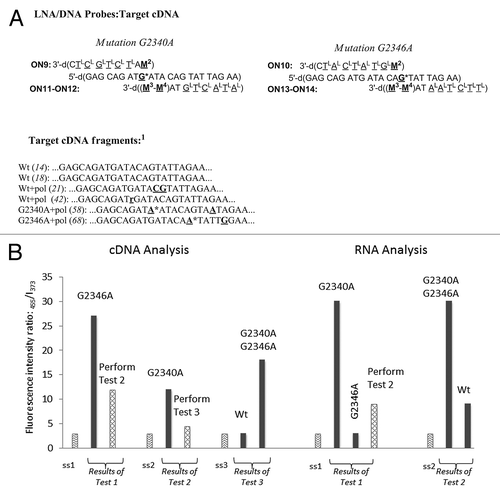
Probes ON9−ON14 were designed for detection of G2340A and G2346A so the fluorescence enhancing signal of full complementarity corresponded to mutations G2340A and G2346A (; preparation and characterization of ON9−ON14 is described in the Supplemental Material). The probes ON9−ON14 showed binding to the model 2332–2354 targetsCitation29 T1−T20 at the temperature of fluorescence measurements (19°C; Tm data not shown). On the other hand, ON9−ON14 did not bind to highly polymorphic sequences T21−T22, which proves their selectivity to the complementary Pol region 2332–2354. Next, fluorescence studies using model targets T1−T20 allowed evaluation of mutation sensing by the probes ON9−ON14 and their characterization with limit of target detection (LOD) values (). Thus, fluorescence sensing by dual-probe systems ON10+ON13 and ON10+ON14 was quite similar with higher fluorescence quantum yield for the latter system (data not shown). Interestingly, we observed difference in sensing cDNA and RNA targets, although in both cases all the genotypes were efficiently analyzed, also in the presence of additional polymorphic sequence variations (; ). The LOD values for ON9−ON14 were < 5 − 10 nM which is comparable and in some cases superior to e.g., widely applied molecular beacons and is sufficient for direct sensing of long PCR fragments.Citation48,Citation49 Finally, annealing of the six purified cDNA fragments with the probe mixtures ON10+ON14, ON9+ON12 and ON9+ON11 (ss1−ss3) in three analytical tubes was followed by fluorescence measurements and calculation of ratiometric parameter I455/I373 (1−3) (; see Patients, Materials and Methods section for detailed description of the assay). As can be seen, the performed fluorescence assay allowed rapid and accurate detection of all the six genotypes within the region of interest Pol in full agreement with the previously obtained sequencing data ( and the Supplemental Material).
Table 3. Detection of mutations G2340A and G2346A in cDNA/RNA targets using FRET LNA/DNA probes ON9−ON14[a]
Discussion
Based on our data, attachment of pyrene in position 4 to 2′-amino-LNA remarkably improved its spectral and photophysical properties compared with the usually applied pyrene-1-yl substituent, also with respect to FRET properties as donor to perylene within nucleic acid complexes. Moreover, fluorescence quantum yield within complementary complexes with DNA/RNA and spectral overlap with perylene were increased for pyrene-4-yl fluorophore compared with the pyrene-1-yl isomer, although the single-stranded probes were quenched by the interactions with polar media in both cases. Changes in dipole−dipole orientation between the pyrene-4-yl and perylene LNA monomers within a dual-probe format allowed rapid and accurate SNP genotyping in cDNA and RNA with a sensitivity lower than 5 nM. This is an important result for the future design of FRET nucleic acid probes for SNP genotyping. Clearly, synthesis of novel pyrene derivatives is a promising strategy for development of novel effective biosensors, as the distinctive advantage of PAH fluorophores in contrast to e.g., cyanines and xanthenes is their high photo- and chemical stability. Together with large Stokes shift (115 nm), target binding affinity and selectivity, pyrene−perylene LNA/DNA FRET probes described herein provide great potential for the development of nucleic acid based biosensors for imaging and clinical diagnostics.
Patients, Materials and Methods
Phosphoramidite reagents 5,Citation41 6Citation42 and 9Citation43 were synthesized following published procedures; phosphoramidite reagent 3 was prepared as described below. Pyrene-1- and pyrene-4-carbaldehydes were obtained from commercial suppliers (Sigma-Aldrich, cat. no. 144037, and Achemica Corp., custom synthesis, respectively). 9,10-Diphenylantracene (DPA), 1-pyrenebutyric acid and perylene used in spectral studies were recrystallized. HPLC grade light petroleum ether, 1,2-dichloroethane, n-hexane and ethyl acetate were distilled and stored over activated 4Å molecular sieves. DCM was distilled over CaH2. All other reagents and solvents obtained from commercial suppliers were used as received. Photochemical studies were performed using spectroquality cyclohexane and methanol.
NMR spectra were recorded at 303 K on Varian Gemini 2000 300 MHz instrument. Chemical shifts are reported in ppm relative to solvents peaks (CDCl3: 7.26 ppm for 1H and 77.0 ppm for 13C; 85% aq. H3PO4: 0.00 ppm for 31P). 1H NMR coupling constants are reported in Hz and refer to apparent multiplicities. High resolution MALDI mass spectra were recorded in positive ion mode using an IonSpec Fourier Transform ICR mass spectrometer. Analytical thin-layer chromatography was performed on Kieselgel 60 F254 precoated aluminum plates (Merck). Silica gel column chromatography was performed using Merck Kieselgel 60 0.040–0.063 mm.
Unmodified DNA/RNA strands were obtained from commercial suppliers and used without further purification. Oligonucleotide synthesis of the fluorescent probes used in this study was performed on a PerSpective Biosystems Expedite 8909 instrument in 200 nmol scale using manufacturer’s standard protocols. For incorporation of monomers MCitation1−MCitation4 a hand-coupling procedure was applied (25 min coupling). The coupling efficiencies of standard DNA phosphoramidites and reagents 3, 5−6 and 9 based on the absorbance of the dimethoxytrityl cation released after each coupling varied between 92% and 100%. Cleavage from solid support and removal of nucleobase protecting groups was performed using 32% aqueous ammonia and methylamine 1:1, v/v, for 4 h at room temperature (5′-terminal and internally labeled probes). In case of 3′-modified probes, a universal support 40 (GE Healthcare) was used. Cleavage from the latter and removal of nucleobase protecting groups was performed using 4 M ammonia in abs. MeOH (1 h, rt), followed by addition of 32% aqueous ammonia (6 h, 55°C). The product oligonucleotides were purified by DMT-ON reverse-phase (RP) HPLC using the Waters System 600 equipped with Xterra MS C18-column (5 μm, 150 mm × 7.8 mm). Elution was performed starting with an isocratic hold of A-buffer for 5 min followed by a linear gradient to 70% B-buffer over 40 min at a flow rate of 1.0 mL/min (A-buffer: 0.05 M triethyl ammonium acetate, pH 7.4; B-buffer: 25% buffer A, 75% CH3CN). RP purification was followed by detritylation (80% aq. AcOH, 20 min), precipitation (acetone, -18°C, 12 h) and washing with acetone three times. The identity and purity of oligonucleotides was then verified by MALDI-TOF mass spectrometry and IE HPLC, respectively. IE HPLC was performed using the Merck Hitachi LaChrom instrument equipped with Dionex DNAPac Pa-100 column (250 mm × 4 mm). Elution was performed starting with an isocratic hold of A- and C-buffers for 2 min followed by a linear gradient to 60% B-buffer over 28 min at a flow rate of 1.0 mL/min (A-buffer: MQ water; B-buffer: 1 M NaClO4, C-buffer: 25 mM Tris-Cl, pH 8.0). MALDI-TOF mass spectrometry analysis was performed using a MALDI-LIFT system on the Ultraflex II TOF/TOF instrument from Bruker and using HPA-matrix (10 mg 3-hydroxypicolinic acid, 50 mM ammonium citrate in 70% aqueous acetonitrile).
Synthesis of modified monomer M2
(1R,3R,4R,7S)-1-(4,4′-Dimethoxytrityloxymethyl)-7-hydroxy-5-(pyren-4-yl)methyl-3-(thymin-1-yl)-2-oxa-5-azabicyclo[2.2.1]heptane (2)
Reductive alkylation of nucleoside 1 (470 mg, 1 mmol) was performed with pyrene-4-carbaldehyde (230 mg, 1 mmol) in the presence of NaBH(OAc)3 (320 mg, 1.5 mmol) in 1,2-dichloromethane (15 mL) at rt for 12 h. A mixture of absolute EtOH with toluene and Et3N (9:9:2, v/v/v) was then added, and the resulting mixture was evaporated to dryness under reduced pressure. The residue obtained was purified by column chromatography (55→60% EtOAc in a light petroleum ether, v/v) to afford nucleoside 2 as a white solid material (560 mg, 87%). 1H NMR (CDCl3) δ 9.25 (br s, 1H, NH), 8.36 (d, J = 7.9 Hz, 1H), 8.14–8.10 (m, 3H), 8.05 (s, 1H), 8.00–7.91 (m, 4H), 7.70 (s, 1H, H6), 7.46–7.43 (m, 2H), 7.34–7.20 (m, 7H), 6.80 (dd, J = 2.1 and 8.8 Hz, 4H), 6.10 (s, 1H, H1′), 4.77 (d, J = 13.4 Hz, 1H, CH2-pyrene), 4.50 (d, J = 13.3 Hz, 1H, CH2-pyrene), 4.26 (s, 1H, H3′), 3.75 (s, 6H, 2 x OCH3), 3.72 (s, 1H, H2′), 3.38 (d, J = 11.5 Hz, 1H, H5′a), 3.34 (d, J = 12.8 Hz, 1H, H5′b), 2.95 (d, J = 10.0 Hz, 1H, H5′′a), 2.65 (d, J = 10.0 Hz, 1H, H5′′b), 1.72 (s, 3H, 5-CH3); 13C NMR (CDCl3) δ 164.1 (C4), 158.7, 150.1 (C2), 144.6, 135.7, 135.5, 135.2, 132.7, 131.6, 131.0, 130.6, 130.2, 130.1, 128.2, 128.1, 128.0, 127.6, 127.3, 127.1, 126.1, 125.9, 125.4, 125.2, 124.4, 121.4, 113.3, 110.4 (C5), 88.9 (C4′), 86.6 (CAr3), 82.6 (C1′), 70.8 (C3′), 66.7 (C5′), 59.7 (C2′), 56.4 (CH2-pyrene), 55.3 (2 × OCH3), 53.7 (C5′′), 12.7 (5-CH3); MALDI-HRMS: m/z 808.2992 ([M+Na]+, C49H43N3O7Na+ calcd 808.2999).
(1R,3R,4R,7S)-7-(2-Cyanoethoxy(diisopropylamino)phosphinoxy)-1-(4,4′-dimethoxytrityloxymethyl)-5-(pyren-4-yl)methyl-3-(thymin-1-yl)-2-oxa-5-azabicyclo[2.2.1]heptane (3)
2-Cyanoethyl N,N-diisopropylphosphoramidochloridite (250 mg, 1 mmol) was added dropwise to a stirred solution of nucleoside 2 (550 mg, 0.7 mmol) and N,N-(diisopropyl)ethylamine (DIPEA, 2.5 mL) in DCM (10 mL). After stirring for 12 h at rt, the reaction mixture was diluted with DCM and the resulting mixture was washed with sat. aq. NaHCO3, dried (Na2SO4) and evaporated to dryness under reduced pressure. The residue obtained was purified by column chromatography (50–55% EtOAc in n-hexane, v/v) to afford phosphoramidite 3 as a white foam (630 mg, 91%). 31P NMR (CDCl3) δ 149.6, 149.4; MALDI-HRMS: m/z 1008.4065 ([M+Na]+, C58H60N5O8PNa+ calcd 1008.4072).
UV-visible absorbance spectra and thermal denaturation experiments
UV-visible absorbance spectra and thermal denaturation experiments were performed on a Perkin Elmer Lambda 35 UV/VIS Spectrometer equipped with PTP 6 (Peltier Temperature Programmer) in a medium salt phosphate buffer (100 mM NaCl, 10 mM Na-phosphate, 0.1 mM EDTA, pH 7.0). Concentrations of oligonucleotides were calculated using the following extinction coefficients (OD260/μmol): G, 10.5; A, 13.9; T/U, 7.9; C, 6.6; MCitation1 20.2; M2, 16.9; M3−MCitation4, 33.2. For determination of the extinction coefficient of pyrene-4-ylmethyl modification, a solution of pyrene-4-carbaldehyde in 0.5% DMSO−water was used. Oligonucleotides (1.0 μM each strand) were thoroughly mixed, denatured by heat (5 min at 85°C) and subsequently cooled to the starting temperature of experiment. Thermal denaturation temperatures (Tm values, °C) were determined as the maximum of the first derivative of the thermal denaturation curve at the corresponding wavelength (A vs. temperature). Reported Tm values are averages of at least two measurements with resulting Tm within ± 0.5°C.
Fluorescence spectra
Fluorescence spectra were recorded in a medium salt phosphate buffer described above on a PerkinElmer LS 55 luminescence spectrometer equipped with a Peltier temperature controller using an excitation wavelength of 340 nm, excitation slit of 4.0 nm, emission slit of 2.5 nm, scan speed of 120 nm/min and 0.25−1.0 μM concentrations of the single-stranded probe or the corresponding complementary complex.
The fluorescence quantum yields
The fluorescence quantum yields (Φf) were measured by the relative methodCitation28,Citation29 using standards of highly diluted solutions of 1-pyrenebutyric acid (Φf = 0.07) in methanol, perylene (Φf = 0.93) and 9,10-diphenylantracene (Φf = 0.95) in cyclohexane. The samples used in quantum yield measurements were not degassed; concentrations were 0.25 μM. Excitation spectra were obtained using emission wavelength at 510 nm.
Discrimination factor
Discrimination factor (DF) valuesCitation50 were determined as a ratio of fluorescent signal (fluorescence intensity or fluorescence intensity ratio) at corresponding λfl of fully-matched duplex to single-base mismatched one. DF > 1 = quenching of fluorescence at corresponding λfl; DF < 1 = fluorescence light-up.
FRET efficiency values
FRET efficiency values E for pyrene-perylene FRET pairs were calculated using equiation:Citation35 E = εA/εD × (IAD/IA - 1), where εA and εD are the molar extinction coefficients of the acceptor and donor at the excitation wavelength, and IAD and IA are the fluorescence intensities at the acceptor emission wavelength in the presence and absence of the donor, respectively.
Limit of target detection (LOD) values
Limit of target detection (LOD) values were determined by series of dilution experiments following previously described protocol.Citation29 Relevant fluorescent oligonucleotides at concentrations 500, 250, 100, 50, 20, 10, 5, and 1 nM were mixed in a medium salt phosphate buffer in molar ratios described above. Upon annealing, a fluorescence signal was measured at λfl 455 nm. LOD value of each complex was then defined as a lowest complex concentration such that the fluorescence signal to noice ratio (S/N) relative to the blank solution of a medium salt phosphate buffer was minimum three.
Förster distances
Förster distances R0 were determined using Förster Energy Transfer module of PhotochemCAD ver. 2.0 softwareCitation51 and using the following equations:Citation44
R0 = (8.79 × 10−5J ϕDn−4 κ2)-1/6 (in Å) (1)
J = ∫ εA (λ) fD (λ) λ4dλ / ∫ fD (λ) λ4 (in cmCitation6 mmol−1) (2)
where J is the normalized spectral overlap of the donor emission spectrum and the acceptor absorption spectrum, ϕD is the quantum yield for donor emission in absence of the acceptor, n is the refractive index of the solution, κ2 is an orientation factor depending on the relative orientation of the emission dipole of the donor and the excitation dipole of the acceptor, εA (λ) is the extinction coefficient of the acceptor (M−1 cm−1) and fD (λ) is the corrected emission spectrum of the donor per unit wavelength interval. Steady-state fluorescence emission and absorbance spectra of the corresponding complementary oligonucleotides containing donor and acceptor FRET were uploaded into the PhotochemCAD database, as well as FRET efficiency and distance D–A values estimated by fluorescence measurements and molecular modeling described below, respectively. Resulting values using κ2 value of 2/3 (lit.Citation44): R0 = 19.8 Å, J = 3.21 × 10−14 cmCitation6 mmol−1, pair MCitation1/M3; R0 = 20.1 Å, J = 3.20 × 10−14 cmCitation6 mmol−1, pair MCitation1/MCitation4; R0 = 21.9 Å, J = 5.08 × 10−14 cmCitation6 mmol−1, pair MCitation1/M3; R0 = 21.5 Å, J = 5.2 × 10−14 cmCitation6 mmol−1, pair MCitation1/MCitation4. Reference values for pyrene–perylene FRET pair in cyclohexane: R0 = 31.3 Å, J = 2.3 × 10−14 cmCitation6 mmol−1 (2.4 × 10−14 cmCitation6 mmol−1, lit.Citation44).
Molecular modeling
Nucleic acid duplexes were built in B-type helical geometry using Chem3D Pro 12.0 for incorporation of fluorophores and further analyzed by MacroModel V9.1.Citation52 When doing this the structures were minimized using the Polak–Ribiere conjugate gradient method, the all-atom AMBER force field,Citation53 and GB/SA solvation modelCitation54 as implemented in MacroModel V9.1. Non-bonded interactions were treated with extended cut-offs (van der Waals 8.0 Å and electrostatics 20.0 Å).
Isolation of total HIV RNA
from the 200 plasma samples followed by RT-PCR and NESTED-PCR amplification were performed using in-house testing system “Amplisense® HIV-genotype-EPh” obtained from Central Institute of Epidemiology (Moscow, Russia) and PCR amplifier GeneAmp PCR System 2400. Selected patients currently receive combined anti-HIV/AIDS therapy; the negative controls included HIV-negative serum samples and MQ water. Purification of the cDNA products was performed by enzymatic method using ExoSAP-IT® on PCR amplifier GeneAmp PCR System 2400, followed by detection of 570 nucleotides long HIV-1 protease cDNA fragments in 1% agarose gel. Additionally, cDNA fragments from six different patients’ samples were analyzed using standard clinical test “Amplisense® HIV-genotype-EPh” for 1302 nt protease and reverse transcriptase gene fragments. Afterwards, five PCR amplification reactions were performed for each clinical sample. The collected single-stranded cDNA were purified as described above; yield of the resulting 570 nt fragments estimated by measuring OD260 was approx. 0.1 nmol (0.4−0.6 mg).
Dye terminator cycle sequencing of the PCR products
Dye terminator cycle sequencing of the PCR products was performed following standard clinical genotyping protocol in an automatic Beckman CEQ2000XL sequenator using GenomeLabTM DTCS Quick Start Kit (Beckman Coulter). Sequencing results interpretation was performed using Stanford Hospital and Clinics HIVdb Program available on the internet.
Homogeneous fluorescence assay for SNP analysis of HIV-1 Pol cDNA using ON9–ON14.
In separate vials the purified cDNA fragment (10 µL of 1.0 µM stock solutions) and water (negative control) were mixed with the probes ss1−ss3 (25 µL of 1.0 µM stock solution; ss1: ON10 and ON14; ss2: ON9 and ON12; ss3: ON9 and ON11) in DNA hybridization buffer (medium salt PBS containing 10 mM EDTA and 0.1% Tween 20). The vials were marked as “test 1−3” for the mixtures of cDNA with ss1−ss3, respectively; the corresponding negative controls were marked “0.” The resulting samples were annealed at 85°C for 5 min followed by cooling to rt over 1 h. Fluorescence measurements were performed using excitation wavelength of 340 nm, and monitoring emission at 455 nm and 373 nm. Fluorescence response was analyzed using the following ratiometric equations: Test 1: I455/I373 (test 1) / I455/I373 (0) = 9.0 → mutation G2346A; I455/I373 (test 1) / I455/I373 (0) = 4.0 → analysis of test 2; Test 2: I455/I373 (test 2) / I455/I373 (0) = 4.0 → mutation G2340A; I455/I373 (test 2) / I455/I373 (0) = 1.5 → analysis of test 3; Test 3: I455/I373 (test 3) / I455/I373 (0) = 1.0 → wild type target at both positions 2340 and 2346; I455/I373 (test 3) / I455/I373 (0) = 6.0 → both mutations G2340A and G2346A are present.
Homogeneous fluorescence assay for SNP analysis of HIV-1 Pol RNA using ON9−ON10, ON12 and ON14
In separate vials the RNA target (10 µL of 1.0 µM stock solutions) and water (negative control) were mixed with the probes ss1−ss2 (25 µL of 1.0 µM stock solution; ss1: ON9 and ON12; ss2: ON10 and ON14) in DNA hybridization buffer described above. The vials were marked as “test 1−2” for the mixtures of RNA with ss1−ss2, respectively; the corresponding negative controls were marked “0.” The resulting samples were annealed at 85°C for 5 min followed by cooling to rt over 1 h. Fluorescence measurements were performed using excitation wavelength of 340 nm, and monitoring emission at 455 nm and 373 nm. Fluorescence response was analyzed using the following ratiometric equations: Test 1: I455/I373 (test 1) / I455/I373 (0) = 10.0 → mutation G2340A; I455/I373 (test 1) / I455/I373 (0) = 1.0 → mutation G2346A; I455/I373 (test 1) / I455/I373 (0) = 3.0 → analysis of test 2; Test 2: I455/I373 (test 2) / I455/I373 (0) = 10.0 → both mutations G2340A and G2346A are present; I455/I373 (test 2) / I455/I373 (0) = 3.0 → wild type target at both positions 2340 and 2346.
Abbreviations: | ||
LNA | = | locked nucleic acid |
PAH | = | polyaromatic hydrocarbon |
SNP | = | single-nucleotide polymorphism |
PIs | = | protease inhibitors |
FRET | = | Förster resonance energy transfer |
Additional material
Download Zip (1.1 MB)Acknowledgments
The authors acknowledge financial support from The Villum Foundation and The Sapere Aude program of The Danish Council for Independent Research.
Disclosure of Potential Conflicts of Interest
No potential conflicts of interest were disclosed.
Supplemental Materials
Supplemental materials may be found here: http://www.landesbioscience.com/journals/artificialdna/article/25903
References
- Hupfeld J, Efferth T. Review. Drug resistance of human immunodeficiency virus and overcoming it by natural products. In Vivo 2009; 23:1 - 6; PMID: 19368117
- Holmes EC. Virology. Helping the resistance. Science 2010; 328:1243 - 4; http://dx.doi.org/10.1126/science.1190994; PMID: 20522766
- Mehellou Y, De Clercq E. Twenty-six years of anti-HIV drug discovery: where do we stand and where do we go?. J Med Chem 2010; 53:521 - 38; http://dx.doi.org/10.1021/jm900492g; PMID: 19785437
- Twyman RM. Encyclopedia of Diagnostic Genomics and Proteomics, Marcel Dekkar Inc., New York, 2005:1202.
- Costabile M, Quach A, Ferrante A. Molecular approaches in the diagnosis of primary immunodeficiency diseases. Hum Mutat 2006; 27:1163 - 73; http://dx.doi.org/10.1002/humu.20412; PMID: 16960849
- Chen X, Kwok PY. Template-directed dye-terminator incorporation (TDI) assay: a homogeneous DNA diagnostic method based on fluorescence resonance energy transfer. Nucleic Acids Res 1997; 25:347 - 53; http://dx.doi.org/10.1093/nar/25.2.347; PMID: 9016564
- Nazarenko IA, Bhatnagar SK, Hohman RJ. A closed tube format for amplification and detection of DNA based on energy transfer. Nucleic Acids Res 1997; 25:2516 - 21; http://dx.doi.org/10.1093/nar/25.12.2516; PMID: 9171107
- Lopez-Crapez E, Bazin H, Andre E, Noletti J, Grenier J, Mathis G. A homogeneous europium cryptate-based assay for the diagnosis of mutations by time-resolved fluorescence resonance energy transfer. Nucleic Acids Res 2001; 29:E70; http://dx.doi.org/10.1093/nar/29.14.e70; PMID: 11452039
- Bengra C, Mifflin TE, Khripin Y, Manunta P, Williams SM, Jose PA, et al. Genotyping of essential hypertension single-nucleotide polymorphisms by a homogeneous PCR method with universal energy transfer primers. Clin Chem 2002; 48:2131 - 40; PMID: 12446468
- Jares-Erijman EA, Jovin TM. FRET imaging. Nat Biotechnol 2003; 21:1387 - 95; http://dx.doi.org/10.1038/nbt896; PMID: 14595367
- Xiao M, Kwok PY. DNA analysis by fluorescence quenching detection. Genome Res 2003; 13:932 - 9; http://dx.doi.org/10.1101/gr.987803; PMID: 12727909
- Asseline U. Development and applications of fluorescent oligonucleotides. Curr Org Chem 2006; 10:491 - 518; http://dx.doi.org/10.2174/138527206776055349
- Roy R, Hohng S, Ha T. A practical guide to single-molecule FRET. Nat Methods 2008; 5:507 - 16; http://dx.doi.org/10.1038/nmeth.1208; PMID: 18511918
- Malakhov AD, Skorobogatyi MV, Prokhorenko IA, Gontarev SV, Kozhich DT, Stetsenko DA, et al. 1-(Phenylethynyl)pyrene and 9,10-bis(phenylethynyl)anthracene, useful fluorescent dyes for DNA labeling: Excimer formation and energy transfer. Eur J Org Chem 2004; 6:1298 - 307; http://dx.doi.org/10.1002/ejoc.200300677
- Skorobogatyj MV, Pchelintseva AA, Ustinov AV, Korshun VA, Malakhov AD. Perylene attached to DNA through stiff or flexible linker: duplex stability and FRET. Nucleosides Nucleotides Nucleic Acids 2005; 24:931 - 4; http://dx.doi.org/10.1081/NCN-200059283; PMID: 16248065
- Chou CC, Huang YH. Nucleic acid sandwich hybridization assay with quantum dot-induced fluorescence resonance energy transfer for pathogen detection. Sensors (Basel) 2012; 12:16660 - 72; http://dx.doi.org/10.3390/s121216660; PMID: 23211753
- Astakhova IK, Kumar TS, Campbell MA, Ustinov AV, Korshun VA, Wengel J. Branched DNA nanostructures efficiently stabilised and monitored by novel pyrene-perylene 2′-α-L-amino-LNA FRET pairs. Chem Commun (Camb) 2013; 49:511 - 3; http://dx.doi.org/10.1039/c2cc37547h; PMID: 23201901
- Preus S, Wilhelmsson LM. Advances in quantitative FRET-based methods for studying nucleic acids. Chembiochem 2012; 13:1990 - 2001; http://dx.doi.org/10.1002/cbic.201200400; PMID: 22936620
- Freeman R, Girsh J, Jou AF, Ho JA, Hug T, Dernedde J, et al. Optical aptasensors for the analysis of the vascular endothelial growth factor (VEGF). Anal Chem 2012; 84:6192 - 8; http://dx.doi.org/10.1021/ac3011473; PMID: 22746189
- Whitcombe D, Brownie J, Gillard HL, McKechnie D, Theaker J, Newton CR, et al. A homogeneous fluorescence assay for PCR amplicons: its application to real-time, single-tube genotyping. Clin Chem 1998; 44:918 - 23; PMID: 9590362
- Whitcombe D, Theaker J, Guy SP, Brown T, Little S. Detection of PCR products using self-probing amplicons and fluorescence. Nat Biotechnol 1999; 17:804 - 7; http://dx.doi.org/10.1038/11751; PMID: 10429248
- Crockett AO, Wittwer CT. Fluorescein-labeled oligonucleotides for real-time pcr: using the inherent quenching of deoxyguanosine nucleotides. Anal Biochem 2001; 290:89 - 97; http://dx.doi.org/10.1006/abio.2000.4957; PMID: 11180941
- Vaughn CP, Elenitoba-Johnson KSJ. Hybridization-induced dequenching of fluorescein-labeled oligonucleotides: a novel strategy for PCR detection and genotyping. Am J Pathol 2003; 163:29 - 35; http://dx.doi.org/10.1016/S0002-9440(10)63627-9; PMID: 12819008
- Ruiz-Ponte C, Carracedo A, Barros F. Duplication and deletion analysis by fluorescent real-time PCR-based genotyping. Clin Chim Acta 2006; 363:138 - 46; http://dx.doi.org/10.1016/j.cccn.2005.05.044; PMID: 16153621
- Marras SAE, Tyagi S, Kramer FR. Real-time assays with molecular beacons and other fluorescent nucleic acid hybridization probes. Clin Chim Acta 2006; 363:48 - 60; http://dx.doi.org/10.1016/j.cccn.2005.04.037; PMID: 16111667
- Martinez-Serra J, Gutiérrez A, Marcús TF, Soverini S, Amat JC, Navarro-Palou M, et al. Four-channel asymmetric Real-Time PCR hybridization probe assay: a rapid pre-screening method for critical BCR-ABL kinase domain mutations. Clin Biochem 2012; 45:345 - 51; http://dx.doi.org/10.1016/j.clinbiochem.2011.12.026; PMID: 22266405
- De Angelis DA, Dino A. Why FRET over genomics?. Physiol Genomics 1999; 1:93 - 9; PMID: 11015566
- Lakowicz JR. Principles of Fluorescence Spectroscopy, 3th Ed., Springer, Singapore, 2006.
- Astakhova IK, Samokhina E, Babu BR, Wengel J. Novel (phenylethynyl)pyrene-LNA constructs for fluorescence SNP sensing in polymorphic nucleic acid targets. Chembiochem 2012; 13:1509 - 19; http://dx.doi.org/10.1002/cbic.201200079; PMID: 22761036
- Umemoto T, Hrdlicka PJ, Babu BR, Wengel J. Sensitive SNP dual-probe assays based on pyrene-functionalized 2′-amino-LNA: lessons to be learned. [and references therein] Chembiochem 2007; 8:2240 - 8; http://dx.doi.org/10.1002/cbic.200700408; PMID: 17979173
- Kalra N, Babu BR, Parmar VS, Wengel J. Conformationally controlled high-affinity targeting of RNA or DNA by novel 2′-amino-DNA/LNA mixmers and pyrenyl-functionalized 2′-amino-DNA. Org Biomol Chem 2004; 2:2885 - 7; http://dx.doi.org/10.1039/b411626g; PMID: 15480448
- Filichev VV, Astakhova IV, Malakhov AD, Korshun VA, Pedersen EB. 1-, 2-, and 4-ethynylpyrenes in the structure of twisted intercalating nucleic acids: structure, thermal stability, and fluorescence relationship. Chemistry 2008; 14:9968 - 80; http://dx.doi.org/10.1002/chem.200800380; PMID: 18810743
- Korshun VA, Malakhov AD, Astakhova IV, Pedersen EB, Filichev VV. DNA glue: 1-, 2- and 4-ethynylpyrenes in the structure of twisted intercalating nucleic acids (TINAs), DNA duplexes/triplexes and interstrand excimer formation. Nucleic Acids Symp Ser 2008; 52:347 - 8; http://dx.doi.org/10.1093/nass/nrn175
- Astakhova IV, Korshun VA. 2- and 4-Phenylethynylpyrenes, novel fluorescent labeles for DNA. Russ J Bioorganic Chem 2008; 34:510 - 2; http://dx.doi.org/10.1134/S1068162008040171
- Lindegaard D, Madsen AS, Astakhova IV, Malakhov AD, Babu BR, Korshun VA, et al. Pyrene-perylene as a FRET pair coupled to the N2′-functionality of 2′-amino-LNA. Bioorg Med Chem 2008; 16:94 - 9; http://dx.doi.org/10.1016/j.bmc.2007.04.056; PMID: 17920888
- Kashida H, Takatsu T, Sekiguchi K, Asanuma H. An efficient fluorescence resonance energy transfer (FRET) between pyrene and perylene assembled in a DNA duplex and its potential for discriminating single-base changes. Chemistry 2010; 16:2479 - 86; http://dx.doi.org/10.1002/chem.200902078; PMID: 20066689
- Singh SK, Kumar R, Wengel J. Synthesis of 2'-amino-LNA: a novel conformationally restricted high-affinity oligonucleotide analogue with a handle. J Org Chem 1998; 63:10035 - 9; http://dx.doi.org/10.1021/jo9814445
- Madsen AS, Jørgensen AS, Jensen TB, Wengel J. Large scale synthesis of 2′-amino-LNA thymine and 5-methylcytosine nucleosides. J Org Chem 2012; 77:10718 - 28; http://dx.doi.org/10.1021/jo302036h; PMID: 23145501
- Baxter EW, Reitz AB. Reductive Aminations of Carbonyl Compounds with Borohydride and Borane Reducing Agents; Organic Reactions, vol. 59, Wiley, New York, 2002.
- Caruthers MH, Barone AD, Beaucage SL, Dodds DR, Fisher EF, McBride LJ, et al. Chemical synthesis of deoxyoligonucleotides by the phosphoramidite method. Methods Enzymol 1987; 154:287 - 313; http://dx.doi.org/10.1016/0076-6879(87)54081-2; PMID: 3431460
- Hrdlicka PJ, Babu BR, Sørensen MD, Wengel J. Interstrand communication between 2′-N-(pyren-1-yl)methyl-2′-amino-LNA monomers in nucleic acid duplexes: directional control and signalling of full complementarity. Chem Commun (Camb) 2004; 1478 - 9; http://dx.doi.org/10.1039/b404446k; PMID: 15216339
- Astakhova IV, Kumar TS, Wengel J. Fluorescent oligonucleotides containing a novel perylene 2′-amino-α-L-LNA: synthesis and analytical potential. Collect Czech Chem Commun 2011; 76:1347 - 60; http://dx.doi.org/10.1135/cccc2011096
- Astakhova IV, Korshun VA, Jahn K, Kjems J, Wengel J. Perylene attached to 2′-amino-LNA: synthesis, incorporation into oligonucleotides, and remarkable fluorescence properties in vitro and in cell culture. Bioconjug Chem 2008; 19:1995 - 2007; http://dx.doi.org/10.1021/bc800202v; PMID: 18771303
- Masuko M, Ohuchi S, Sode K, Ohtani H, Shimadzu A. Fluorescence resonance energy transfer from pyrene to perylene labels for nucleic acid hybridization assays under homogeneous solution conditions. Nucleic Acids Res 2000; 28:E34; http://dx.doi.org/10.1093/nar/28.8.e34; PMID: 10734211
- Iqbal A, Arslan S, Okumus B, Wilson TJ, Giraud G, Norman DG, et al. Orientation dependence in fluorescent energy transfer between Cy3 and Cy5 terminally attached to double-stranded nucleic acids. Proc Natl Acad Sci U S A 2008; 105:11176 - 81; http://dx.doi.org/10.1073/pnas.0801707105; PMID: 18676615
- Zenger V. Principles of Nucleic Acid Structure, Springer Verlag, 1984.
- Kearney M, Palmer S, Maldarelli F, Shao W, Polis MA, Mican J, et al. Frequent polymorphism at drug resistance sites in HIV-1 protease and reverse transcriptase. AIDS 2008; 22:497 - 501; http://dx.doi.org/10.1097/QAD.0b013e3282f29478; PMID: 18301062
- Gerasimova YV, Kolpashchikov DM. Detection of bacterial 16S rRNA using a molecular beacon-based X sensor. Biosens Bioelectron 2013; 41:386 - 90; http://dx.doi.org/10.1016/j.bios.2012.08.058; PMID: 23021850
- Gao Y, Li Y, Zou X, Huang H, Su X. Highly sensitive and selective detection of biothiols using graphene oxide-based “molecular beacon”-like fluorescent probe. Anal Chim Acta 2012; 731:68 - 74; http://dx.doi.org/10.1016/j.aca.2012.04.020; PMID: 22652266
- Xiao Y, Plakos KJI, Lou XH, White RJ, Qian JR, Plaxco KW, et al. An Electrochemical Sensor for Single Nucleotide Polymorphism Detection in Serum Based on a Triple-Stem DNA Probe. Angew Chem Int Ed 2009; 48:4354 - 8; http://dx.doi.org/10.1002/anie.200900369
- Dixon JM, Taniguchi M, Lindsey JS. PhotochemCAD 2: a refined program with accompanying spectral databases for photochemical calculations. Photochem Photobiol 2005; 81:212 - 3; http://dx.doi.org/10.1562/2004-11-06-TSN-361.1; PMID: 15571431
- MacroModel, version 9.1, Schrödinger, LLC, New York, NY, 2005.
- Weiner SJ, Kollman PA, Case DA, Singh UC, Ghio C, Alagona G. A new force field for molecular mechanical simulation of nucleic acids and proteins. J Am Chem Soc 1984; 106:765 - 84; http://dx.doi.org/10.1021/ja00315a051
- Weiner SJ, Kollman PA, Nguen DT, Case DA. An All Atom Force Field for Simulations of Proteins and Nucleic Acids. J Comput Chem 1986; 7:230 - 52; http://dx.doi.org/10.1002/jcc.540070216