Abstract
Tissue engineering has emerged as a possible alternative to current treatments for bone injuries and defects. However, the common tissue engineering approach presents some obstacles to the development of functional tissues, such as insufficient nutrient and metabolite transport and non-homogenous cell distribution. Culture of bone cells in three-dimensional constructs in bioreactor systems is a solution for those problems as it improves mass transport in the culture system. For bone tissue engineering spinner flasks, rotating wall vessels and perfusion systems have been investigated, and based on these, variations that support cell seeding and mechanical stimulation have also been researched. This review aims at providing an overview of the concepts, advantages and future applications of bioreactor systems for bone tissue engineering with emphasis on the design of different perfusion systems and parameters that can be „optimized.
Introduction
Current medical treatments for large bone defects or injuries caused by trauma, tumor, tissue degeneration or congenital deformities are based on bone-grafting surgeries.Citation1,Citation2 The bone grafts consist of autografts, allografts, xenografts, synthetic materials or de-mineralized bone matrix, depending on the bone source. The most common treatment is the autograft transplant. However, it is restricted by the limited availability of bone, which is harvested from the iliac crest, and by medical complications following the harvesting such as infection, nerve and arterial injury and chronic pain.Citation2-Citation4 Allografts and xenografts are also limited due to the risk of rejection and disease.Citation5,Citation6 The synthetic materials that can be used as bone substitutes include mainly hydroxyapatite, coralline hydroxyapatite, tricalcium phosphate, biphasic calcium phosphates and bioglasses. De-mineralized bone matrix, produced through the decalcification of cortical bone, can also be used to try and reduce the potential for immunogenic host response.Citation7,Citation8 All of the synthetic materials commonly used are hard, porous and osteoconductive, yet medical applications are limited due to possible resorption after long-term implantation and brittleness.Citation9,Citation10 Tissue engineering has emerged as a possible alternative for the described treatments as it would provide functional substitutes for the native tissues and models for studies on tissue formation and development. A tissue engineering approach involves the implantation in the site of injury of a 3D porous biodegradable structure seeded with osteoblastic or mesenchymal stem cells, which are one valuable cell source for orthopedic applications and its growth rate and osteogenic differentiation have been studied for years.Citation11 The construct would provide a template for tissue development and should be biodegradable in order to eliminate the need for surgical removal.Citation12,Citation13 Biodegradable polymers used for bone tissue engineering include silk, chitosan, collagen and polyglycolic acids.Citation14 For this strategy to lead to a functional substitute it would be important that certain parameters such as cell seeding density, culture period, scaffold architecture, scaffold composition, cell source, matrix deposition and mineralization are optimized.Citation15-Citation17 Although the described approach allows cell growth and proliferation, when dealing with 3D structures in static culture (where there is not any mixing or circulation of the culture medium), nutrient transport only occurs by diffusion which leads to higher concentrations of nutrients and metabolites at the scaffold’s surface which in turn may constrain cell migration to the interior of the construct and tissue formation.Citation6,Citation18 Limitations of cell culturing on three-dimensional scaffolds under static conditions include insufficient nutrient and oxygen transport and waste removal which will cause decreased proliferation and differentiation and non-uniform cell distribution. This also limits the dimensions of the scaffolds that can be used on static culture.Citation19 In traditional two-dimensional culture techniques diffusion is enough to provide nutrients and oxygen to all the cells and waste removal, but when using three-dimensional constructs, diffusion is not sufficient.Citation20 Hence more complex bioreactor systems can be used to improve culture media circulation and convective transport of nutrients to the cells, allowing the development of a more uniform tissue. Bioreactors use materials that are widely used for bone tissue engineering. These materials are seeded with cells capable of proliferating and differentiating into osteoblasts and are maintained under dynamic culture. Bioreactors bring several advantages into the culture of functional tissues. They not only increase mass transport inside three-dimensional structures, but also reduce the number of handling steps, hence reducing contamination potential. They also permit control and monitoring of environmental conditions such as pH, temperature, oxygen and carbon dioxide concentrations and nutrient supply.Citation1,Citation21 For bone tissue engineering it is also worth considering the fluid shear stress caused by mixing or perfusion of culture medium that will expose the cells to mechanical stimulation. In vivo, mechanical loading driven fluid can increase production of prostaglandins, alkaline phosphatase (ALP), collagen type I, along with osteoblast proliferation and mineralization.Citation22 It is thought that interstitial fluid flow through lacunar and canalicular spaces in bones is caused by mechanical loading of the skeleton. The cells lining these spaces are then influenced by the mechanostimulation provided by the fluid flow, differentiating or proliferating accordingly.Citation23,Citation24 Thus, this becomes a clear advantage when using fluid flow in in vitro culture. Bioreactor design should account for the following basic requirements: controlled and fast cell expansion, efficient exchange of nutrients, oxygen and metabolites in all parts of the scaffold, enhanced cell seeding and provision of physical or biochemical stimuli.Citation25 The most common bioreactor types are spinner flasks,Citation26 rotating wall vesselsCitation7,Citation27 and perfusion systems.Citation28-Citation30 Spinner flask and rotating wall vessel are two alternatives to static culture that try to minimize gradients in nutrient and metabolite concentrations. Both use convection to ensure that culture media around the scaffolds is well mixed thus leading to enhanced nutrient transport into the porous structure.Citation27 Perfusion systems, on the other hand, are the most complex as they can perfuse fluid directly through the structures, ensuring good mass transport inside the constructs, and they have been shown to upregulate expression of osteoblastic markers.Citation27,Citation31,Citation32
Spinner Flasks
To address the issues of low seeding efficiency and other limitations present in static culture, spinner flask bioreactors were introduced in order to create a convective flow and produce hydrodynamic forces that will help increase mass transport.Citation28 Spinner flasks are simple bioreactor systems composed by a cylindrical container with side arms for removal of cells and medium and a stirring element at the bottom ensuring circulation and mixing of culture medium. The scaffolds used in these systems are in fixed positions, threaded in needles attached to the cap of the container.Citation14,Citation33,Citation34 Spinner flask bioreactors can mimic some aspects of native bone environment which allows them to be used for several studies regarding bone tissue formation and cell function.Citation26 The use of spinner flask culture systems has shown a positive effect in accelerating human mesenchymal stem cells (hMSCs) differentiation into osteoblasts cultured in silk fibroin scaffolds. These were maintained in both static and dynamic culture for 84 d and the peak for ALP activity was, respectively, on days 28 and 56. Also, constructs cultured on spinner flasks showed connective tissue and mineralized nodule formation after 14 d of culture whereas in static culture this was only seen on day 56.Citation22 ALP activity is used as a marker for early osteoblast differentiation because it peaks during the matrix maturation phase when the cells are committing to the differentiated phenotype and decreases at a later stage when the cells are starting to mature and form mineralized ECM.Citation35 hMSCs cultured in PLGA [poly(lactic-co-glycolic acid)] foams in this system also had higher ALP activity and calcium deposition, compared with those cultures in static conditions and gene expression of COLA1A (collagen type I α 1), Runx2 (runt-related transcription factor-2), OSX (osterix), ON (osteonectin), BMP2 (bone morphogenetic protein protein-2) genes was upregulated at days 7 and 21 of culture.Citation19 In collagen scaffolds these cells also exhibit higher ALP activity and its peak was followed by mineralization but mineralized matrix only formed in the outer rim of the scaffold, not penetrating more than 0.5 mm to 1 mm of the 11 mm diameter scaffolds, which corresponds to the penetration depth of the fluid.Citation17 Culture of immortalized hMSCs on coralline hydroxyapatite scaffolds showed that the pore size affects in-growth and differentiation of hMSCs.Citation36,Citation37 ALP expression and rate of differentiation was higher on 200 µm pore size scaffolds compared with 500 µm pore size scaffolds. Although spinner flasks present advantages when compared with static culture and, in some cases, even with rotating wall vessels, they only permit extracellular matrix production at the scaffolds surface. Mixing the media is associated with turbulent shear at the surfaces which can also be detrimental to cell growth and tissue formation.Citation17,Citation30 It is then necessary to look at alternatives that allow for a better penetration of cells and culture medium in the scaffolds.
Rotating Wall Vessels
The rotating wall vessel was first developed to create a microgravity environment, but when it was first tested using cell suspensions it was observed that the cells aggregated and formed tissue-like spheroids which opened the possibility of using this type of reactor for co-cultures of different cell types or for differentiation in early steps of tissue formation.Citation38 Rotating bioreactors provide controlled supply of oxygen and have low shear stress and turbulence, which are major advantages when compared with the stirred flasks.Citation39 The most common rotating wall bioreactor is composed by two concentric cylinders: the outer cylinder consists of the culture chamber filled with culture medium and where the scaffolds or microcarriers are placed and the inner cylinder is static and permits gas exchange.Citation6 The culture chamber rotates horizontally around its axis, randomizing gravitational forces that act on the cell surface and as it rotates the culture medium inside is accelerated until the entire fluid mass is rotating at the same angular rate as the wall.Citation38 Comparing the use of a rotating wall bioreactor and a spinner flask using PLGA scaffolds seeded with rat MSCs, it was observed that the ALP activity for cells in scaffolds cultured in the rotating wall vessel was lower than for samples cultured in the spinner flask and in static culture.Citation27 Osteocalcin secretion was also observed to be lower than in samples cultured in stirred flasks. In both systems cell growth and mineralization were limited to the outside region of the scaffolds.Citation6 hMSCs cultured in gelatin-hyaluronic acid scaffolds also presented similar behavior.Citation26 Particle dynamics studies show that the shear stress endured by a microcarrier increases with the density difference between the culture medium and the microcarrier. Most of the scaffolds used in these systems are denser than the surrounding medium in rotating vessels, thus they impart higher shear stress and centrifugal forces cause them to collide with the walls of the culture chamber during rotation.Citation7 This leads to cell damage and interferes with cell attachment and deposition of mineralized matrix.Citation2 This could be the explanation behind the disappointing results obtained for the comparison of rotating wall reactors with spinner flasks. Bearing this problem in mind, several groups developed lighter-than-water scaffolds or microcarriers that exhibit migration toward the center and avoid collisions with the walls.Citation40 Using lighter-than-water microcarriers of PLGA seeded with human osteoblastic cells (Saos-2), ALP staining was positive on day 7 and was significantly higher for samples cultured in the rotating wall bioreactor compared with non-rotating 3D controls. Substantial amount of calcified matrix was also detected by alizarin histochemical staining.Citation41 These cells, however, appear to migrate toward the inner region of the microcarriers as cell in-growth covered the entire depth of the 2.5 mm PLGA microcarriers.Citation42 When rat calvarial osteoblastic cells were used, similar results were found for ALP expression and matrix mineralization and also the expression levels of osteopontin and osteocalcin significantly increased under rotating conditions.Citation2 Values of fluid shear were estimated and were in the range of 0.16–32 N/m2 (1.6–320 dyn/cm2), which are similar to the estimates of physiological level of shear stress on osteocytes under flow.Citation43 hMSCs were cultured in silk scaffolds in rotating bioreactors for 36 d and the presence of mineralized matrix and collagen type I were visible by staining and microCT. Also the mineralized structures were distributed throughout the entire volume of the constructs.Citation44 Hollow ceramic microspheres were also used as microcarriers for rotating bioreactors with rat MSCs and rat osteosarcoma cells (ROS 17/2.8). The formation of an aggregate was seen after 10 d of culture. Most of the beads were entirely covered with cells and extensive production of extracellular matrix was also visible. Early stages of mineralization were identified by the presence of nodules in the matrix.Citation39 Similar results were obtained using rat MSCs.Citation45 A variation of the common rotating bioreactor was developed and it consists in a new rotational oxygen-permeable bioreactor system (ROBS). The objective of this system is to supply optimal oxygen levels and continuous hydrostatic pressure to biodegradable polymer scaffolds. It consists of a polypropylene centrifuge tube modified with a silicon elastomer to allow gas exchange. The tube is then placed in a roller device and maintained in an incubator.Citation46 PLGA foams were seeded with rat MSCs and cultured for 5 weeks in this system and histological analysis showed that the density of the extracellular matrix produced increased and became partially calcified after 2 weeks. After 3 weeks extensive calcification was observed. The same system was also used with polycaprolactone (PCL) scaffolds and after 4 weeks there was abundant presence of calcified matrix and collagen type I and cell migration had occurred inside the scaffold.Citation47 One other variation of the rotating wall bioreactor system that consists on attaching the scaffolds to the vessels wall was used to culture rat osteoblasts on bio-derived bone scaffolds and showed higher proliferation rates, more ECM production and mineralization when compared with both spinner flasks and static culture.Citation48
Rotating wall bioreactors managed to solve some of the problems associated with static cultures and partially improved culture conditions with regards to stirred flasks, but when it comes to expression of osteoblastic markers the results are not satisfactory probably due to the low shear stresses or collisions with the vessel wall. Still, the advantages over the stirred flasks are not clear which is why it is important to try and develop better alternatives.Citation6,Citation26,Citation27,Citation38,Citation48
Perfusion Bioreactors
To overcome the limitations of the system already described, systems that use flow perfusion have started being used for bone regeneration. These reactors present an advantage when compared with stirred flasks and rotating wall vessels because they provide more uniform mixing of the media hence allowing for a better environmental control and physical stimulation of the cells in large constructs.Citation49 These bioreactors use a pump system that can perfuse media through the scaffolds in a continuous or non-continuous way. Several types of perfusion bioreactors have been tested, but most of them have a similar basic design which consists of a pump, a culture media reservoir, a tubing circuit and cartridges, chambers or columns that hold the scaffolds.Citation1,Citation16,Citation50 The scaffolds need interconnective pores and should have between 70% to 99% porosity in order to facilitate direct perfusion. In most cases the major difference between the systems is the design of the perfusion chamber because it is the key element to ensure thorough perfusion of the center of the structures. One of the most common systems that uses perfusion is described in detail by Bancroft et al.Citation29 This system consists of six flow chambers and two media reservoirs. Each flow chamber contains a cassette where the scaffold is held between two neoprene o-rings that are held tightly against the cassette screw on top. The media flows from top to bottom through the scaffold to prevent the trapping of air bubbles.Citation29,Citation51 A schematic representation as well as a detail of the perfusion chamber can be seen in .
Figure 1. Schematic of the perfusion system described by Bancroft et al. (A) Top view of the perfusion chamber with six scaffold holders. (B) Representation of the complete system with the scaffold represented in gray, press-fit between the two O-rings, in black. The two medium reservoirs, 1 and 2, allow for complete medium change when the connection between the two is closed. Arrows represent medium flow.
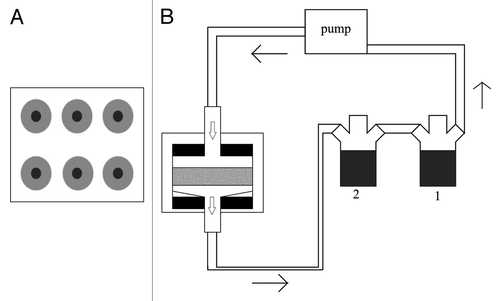
This perfusion system has been used with several types of materials and scaffolds. Perhaps one of the most used is the titanium fiber mesh scaffold.Citation23,Citation35,Citation51,Citation52 When cultured with rat MSCs, these scaffolds in the flow perfusion system have been shown to accelerate differentiation, as shown by ALP activity. Several studies showed that flow perfusion, when compared with static culture, leads to significantly higher values of ALP activity.Citation27,Citation53,Citation54 Late osteoblast differentiation is evaluated by osteopontin expression. Osteopontin is a glycoprotein synthesized by osteoblasts that is produced during the stages of differentiation that precede mineralization.Citation51 It has also been shown that flow perfusion culture facilitates an increase in the osteopontin secretion, which leads to believe that osteoblast differentiation is enhanced by flow perfusion culture.Citation52 Similar results have been obtained using porous calcium phosphate ceramics.Citation55 Real-time RT-PCR performed on hMSCs cultured on silicate-substituted tricalcium phosphate on the same perfusion system also corroborated the increase of ALP activity and osteopontin expression, showing significantly higher expression than in static culture.Citation31 The endpoint of osteoblastic differentiation is the production of a mineralized matrix and should be seen in long-term culture if the cells are differentiating.Citation23 A mineralized calcium matrix has been obtained in a number of studies that aim at differentiating marrow stromal cells under flow perfusion,Citation55-Citation57 but most importantly a more uniform ECM distribution has been obtained in flow perfusion systems, as shown by micro CT with PLLA non-woven scaffolds, while the matrix produced under static conditions was denser in the scaffold peripheral regions.Citation23,Citation57
To increase biological relevance biodegradable scaffolds were also used with the same perfusion system.Citation53,Citation58,Citation59 One study was performed using starch-based biodegradable scaffold and again, ALP activity was used as a marker for early differentiation of marrow stromal cells and calcium deposition for late differentiation. Although there was an increase in ALP activity for both dynamic and static cultures, it was significantly higher for the scaffolds culture under flow perfusion. Also calcium deposition increased dramatically after two weeks of culture under flow perfusion suggesting that it enhanced mineralization and differentiation due to fluid shear induced mechanical stimulation and improvement of possible nutrient transport limitations in static culture.Citation53 PLLA non-woven resorbable scaffolds were also cultured with rat MSCs, and an increase was also seen in calcified matrix deposition in dynamic culture.Citation59 A modification of the system was used to allow the use of oscillating flow and study its influence on cell seeding, showing that oscillating perfusion yields higher seeding densities, more homogenous cell distributions and stronger cell-matrix interactions.Citation28,Citation60 Looking only at the results it seems that perfusion systems present clear advantages when compared with spinner flasks and rotating wall vessels. Mass transport limitations are not an issue with most perfusion reactors and better results have been obtained with respect to differentiation, proliferation and expression of osteoblastic phenotype markers. Yet, further research is still required to improve these systems to allow standardization of the procedures necessary to the development of a functional bone substitute in a bioreactor. Parameters that can help toward the development of more efficient systems are described in following sections.
The Use of Different Perfusion Chambers
The design of the perfusion chamber is of the outmost importance. Bancroft et al. have described a system that holds six samples in individual chambers. The culture medium enters though a single hole on the top of the chamber and exits through another one in the bottom.Citation29 The culture medium is forced through the chamber and goes around and through the porous scaffold (). Another system () incorporates a chamber for a single scaffold with perforated lid and bottom.Citation30,Citation61 In this case this perforated basket is placed in the medium flow path and the medium is forced through the scaffolds. Because this chamber presents a perforated top there seems to be a better distribution of medium on the surface of the scaffolds which may allow for a more uniform penetration throughout the structure than with the previous one. The system described by Cartmell et al. is similar to these two with the exception that it holds eight samples in individual chambers (), but the scaffolds are held in a very similar manner.Citation62 Finally another example is the one described by Grayson et al.Citation32 This one consists on a circular chamber with the dimensions of a Petri dish where six samples are held in individual spaces (). The medium goes in through one end and it is distributed equally between the six scaffolds.
Figure 2. Schematic of the perfusion system described by Janssen et al. (A) Top view of the perforated lid and bottom, (B) detail of the perfusion chamber (scaffolds in gray and O-rings in black) and (C) representation of the complete system. Oxygen sensors are placed before and after the perfusion chamber.
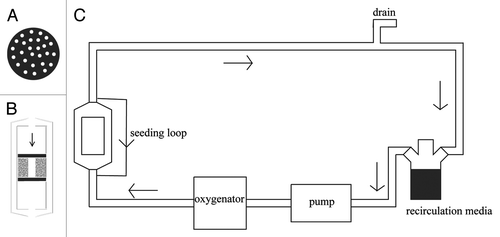
Figure 3. (A) Representation of the perfusion chamber described by Grayson et al.Citation32 The media goes in through one end and it is distributed equally by the six individual chambers (each holding one scaffold shown in gray) and finally goes out through the opposite end. (B) Representation of the system described by Cartmell et al.Citation62 The perfusion block is composed by eight individual chambers (each holding one scaffold, entrance of the chamber shown in gray). Each chamber is fed individually by a tube that comes from the reservoir.
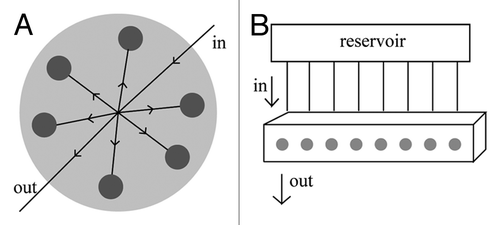
All of these examples have shown interesting results and there is not one that seems superior to the other, but it is important to consider that they are other parameters to consider and that depending on the material being used and on its dimensions there may be need to optimize the design of the chamber. Most importantly to actually obtain clinically relevant amounts of regenerated bone the perfusion chambers described might not be suitable as it is more complicated to force medium through a thicker or larger structure and ensure a uniform distribution of media and oxygen throughout the scaffold’s depth and surface.
The Importance of Flow Rate and Shear Stress
One of the most important parameters when optimizing a bioreactor for this application is the flow rate and it needs to be considered that its value will also be affected by the design of the reactor and by the architecture of the three dimensional structure used in the system. Bearing this in mind, different flow rates have been experimented on some of the different perfusion systems investigated. In the values of flow rate for some of the reactors available can be seen. Still, there is a big variation of values and there are not many studies that compare a significant range of flow rates. However, in some cases, it is possible to detect a tendency. It appears that, up to a point, the increase in flow rate leads to an increase in the deposition of mineralized matrix.Citation51 Very low flow rates such as 0.01 ml/min have been reported to lead to higher cell viability than higher values, but this does not seem an optimal flow rate for bone tissue engineering applications as it might be too low to actually accomplish an adequate distribution of nutrients, oxygen and removal of waste products.Citation51,Citation62 It is also necessary to bear in mind that lower values of flow rate will provide lower values of shear stress which might facilitate cell attachment and spreading, hence leading to higher values of cell viability. Despite the wide variation of flow rates tested, it seems that the optimal values would range from 0.2 to 1 ml/min, depending obviously on the system being used. This is the range of values that seems to have a more positive effect on osteoblastic differentiation, ECM deposition and distribution. Higher values such as 4 ml/min have been tested in comparison with static culture and after 19 d of culture there was not a significant difference in the amount of newly formed bone between the two.Citation30 Although this is not of the most disappointing results, very high flow rates can have a negative effect on cell attachment and disrupt formation and deposition of ECM, therefore obliterating the benefits associated with perfusion systems. Considering all of this, it seems of the outmost importance to optimize this parameter, but most studies in the field only look at distribution of seeded cells, micro-architecture and cell differentiation without actually optimizing the design and its flow characteristics. One example that shows the importance of this is described by Jaasma et al.Citation63 and in this work a new reactor design is validated by evaluating its performance within a range of flow rates of interest and assessing flow profiles for steady, oscillatory and pulsatile flow. However, it is obvious that testing so many different parameters is exhausting and time consuming. One possible alternative is the use of computational fluid dynamics,Citation64,Citation65 but these simulations are computationally intensive and can end up being as time consuming as the actual testing. To avoid these problems it has been suggested that approximations to model the fluid flow can be done based on the Darcy law (Eqn. 1, where u represents the volume-averaged velocity and it is proportional to K, the permeability tensor divided by the viscosity, µ, and to the pressure gradient, p) that describes fluid flow through a porous medium and this can be a simpler approach to understanding what actually goes on inside the constructs and the perfusion chamber.Citation66
Table 1. Selected perfusion systems corresponding flow rates and scaffolds used with them and respective pore sizes
Another very important parameter that is closely related with the flow rate (τ) is the shear stress. The shear stress on a point y at a distance from the surface is given by Equation 2, where µ is the viscosity and
the velocity of the fluid on the surface.Citation67
In vivo, bone cells are subjected to shear stresses that range from 8 to 30 dyn/cm2 and in vitro has been shown that values from 2 to 10 dyn/cm2 are sufficient to stimulate osteoblasts.Citation59,Citation68 In the three dimensional constructs used under flow perfusion the values of shear stress to which cells are subjected are represented on . It can be seen that in these cases, the shear stress is very low, barely reaching 1 dyn/cm2, which is lower than the values that have been shown to stimulate osteoblasts. As seen in Equation 2, the viscosity also influences the shear stress. This has also been studied by the supplementation of culture medium with different concentrations of dextran (0%, 3% and 6%). The increase of the concentration of dextran leads to an increase in viscosity. It was seen that the increasing concentration led to an increase in shear stress from 0.1 to 0.3 dyn/cm2 and that it also improved distribution and amount of mineralized matrix.Citation23 Varying viscosity might be another alternative to study the effect of shear stress without altering scaffold architecture.
Table 2. Selected perfusion systems and respective shear stresses
Bearing in mind the importance of this parameter, it is necessary to try and optimize it as it might have a great influence on the osteoblastic behavior. Still, it is a difficult parameter to alter as it is influenced by characteristics such as pore size and flow rate and, although it can be easily estimated in some cases, there are situations where it is not possible to obtain accurate values and this is mainly due to the scaffold’s architecture. The commonly used fluid flow model assumes that the scaffolds present a cylindrical pore geometry which is not precise in cases where fibrous meshes are used, for example, but the approximation can be made nonetheless.Citation52
As seen in , the pore size of the constructs used varies greatly and there are only a few studies comparing different pore sizes. One study that used two titanium meshes with different diameters (20 and 40 µm) showed that the large diameter had a positive influence on early osteoblast differentiation and the smaller diameter had a more significative influence on the later differentiation and matrix deposition. The authors explain this by stating that the decrease in mesh size increases the shear stress and it seems to prove that the importance of shear stress on cell differentiation may vary depending on its stage.Citation52 A different group also tested different pore sizes (from 200 to 500 µm) in coralline hydroxyapatite but the results were not very conclusive regarding the effect of the varying pore size.Citation36 These studies clearly show that there is still a lot of work to be done regarding pore size and scaffold architecture and these have to be investigated alongside flow rate and shear stress to better understand the how the fluid behaves in the perfusion systems.
The Effect of Dynamic Flow
Most of the systems described in previous sections support steady perfusion, meaning that there is a continuous flow through the scaffolds, but there are several systems that incorporate oscillatory or pulsatile flow (see for details). Overall oscillatory flow seems to have a beneficial effect over static culture. Du et al.Citation69 showed that it leads to increases in the amount of DNA and in ALP activity, with the last one being justified by the mechanical stimulation due to the oscillatory shear flow. This work only compares the oscillatory flow with static culture and it could be of interest to also compare with steady flow. The authors also suggest that the use of a non-continuous flow might be an advantage in a way that allows cells that detach to reseed again on the scaffold. Other studies have compared the use of continuous and non-continuous flow. The same author has performed this comparison and also showed an increase in ALP activity over the unidirectional continuous flow. The system described by that work also supports seeding and it also showed good results: it was seen a uniform distribution and proliferation of cells throughout the scaffolds.Citation70 Another work reporting cyclic compression also showed improvements when compared with steady perfusion, as it led to an increase in OCN and Runx2 expression after 21 d of culture.Citation71 Although there are a few studies showing that non-continuous flow has several advantages when compared with continuous flow, there are also a few studies that show that, although there is a slight increase in osteogenic markers, there doesn’t seem to be a significant difference between the two and that it is not possible to detect a trend in the varying frequencies, but this might only be due to the use of different perfusion systems, different cell types, scaffold and range of frequencies.Citation72 The use of pulsatile flow also appears to have an effect on the expression of BMPs 2, 4 and 7, TGF-β and prostaglandin E2 expression.Citation73,Citation74
Table 3. Selected perfusion systems that support oscillatory or pulsatile flow and some relevant parameters
Although it has not been shown that these systems are clearly superior to the ones that only support steady flow, there seems to an advantage when looking at cell seeding. Most of the dynamic flow systems support seeding and it seems to lead to more homogenous cell distributions and more uniform proliferation. Also, in most perfusion systems, the seeding is performed statically which will definitely have an effect on the final cell distribution and might not be an ideal alternative when studying dynamic culture.Citation69,Citation70 Nevertheless there are a few systems that have been developed to support cell seeding and have shown promising results.Citation30,Citation61
Concluding Remarks
Bioreactor systems allow monitoring of environmental factors that provide the means to a better understanding of biological, chemical and physical factors involved in the formation of a specific tissue. Spinner flasks and rotating wall vessels have shown improvements over static culture, but they still are unable to promote adequate cell migration to the inner regions of the three-dimensional constructs and uniform formation of extracellular matrix. Although they still present some disadvantages, these systems are quite easy to implement and might be optimized. Although perfusion systems present a more complex alternative, they have shown better results regarding the induction of osteogenic differentiation and de novo bone formation in vivo. Still, there is a lot of progress to be made with these systems. Flow rates need to be optimized to try and supply the cells with a shear stress that approaches the in vivo values. It is also relevant to look at the cell seeding. Most systems don’t support dynamic seeding and they should as this would reduce handling steps and it would make the reactors more clinically relevant. Also the reactors that support oscillatory or pulsatile flow seem to be a promising approach to solving this problem. More progress needs to be made to allow the implementation of these systems in a clinical environment. To achieve that they should support cell extraction from the patient, cell proliferation in order to obtain the necessary cell number, seeding in a biodegradable construct and automatic culture medium change to decrease the number of handling steps and supply adequate biochemical and physical differentiation cues, thus producing a ready-to-use functional substitute. For a generalized use of a tissue engineering approach based on bioreactors it is also necessary to make the up-scaling step from the laboratory to an industrial-scale. Only then tissue engineering based treatments will be economically viable and widely available.
References
- Yeatts AB, Fisher JP. Bone tissue engineering bioreactors: dynamic culture and the influence of shear stress. Bone 2011; 48:171 - 81; http://dx.doi.org/10.1016/j.bone.2010.09.138; PMID: 20932947
- Yu X, Botchwey EA, Levine EM, Pollack SR, Laurencin CT. Bioreactor-based bone tissue engineering: the influence of dynamic flow on osteoblast phenotypic expression and matrix mineralization. Proc Natl Acad Sci U S A 2004; 101:11203 - 8; http://dx.doi.org/10.1073/pnas.0402532101; PMID: 15277663
- Hill NM, Horne JG, Devane PA. Donor site morbidity in the iliac crest bone graft. Aust N Z J Surg 1999; 69:726 - 8; http://dx.doi.org/10.1046/j.1440-1622.1999.01674.x; PMID: 10527350
- Seiler JG 3rd, Johnson J. Iliac crest autogenous bone grafting: donor site complications. J South Orthop Assoc 2000; 9:91 - 7; PMID: 10901646
- Cypher TJ, Grossman JP. Biological principles of bone graft healing. J Foot Ankle Surg 1996; 35:413 - 7; http://dx.doi.org/10.1016/S1067-2516(96)80061-5; PMID: 8915864
- Sikavitsas VI, Bancroft GN, Mikos AG. Formation of three-dimensional cell/polymer constructs for bone tissue engineering in a spinner flask and a rotating wall vessel bioreactor. J Biomed Mater Res 2002; 62:136 - 48; http://dx.doi.org/10.1002/jbm.10150; PMID: 12124795
- Gao H, Ayyaswamy PS, Ducheyne P. Dynamics of a microcarrier particle in the simulated microgravity environment of a rotating-wall vessel. Microgravity Sci Technol 1997; 10:154 - 65; PMID: 11543416
- Moore WR, Graves SE, Bain GI. Synthetic bone graft substitutes. ANZ J Surg 2001; 71:354 - 61; http://dx.doi.org/10.1046/j.1440-1622.2001.02128.x; PMID: 11409021
- Bongio M, van den Beucken JJJP, Leeuwenburgh SCG, Jansen JA. Development of bone substitute materials: from ‘biocompatible’to ‘instructive’. J Mater Chem 2010; 20:8747 - 59; http://dx.doi.org/10.1039/c0jm00795a
- Calori GM, Mazza E, Colombo M, Ripamonti C. The use of bone-graft substitutes in large bone defects: any specific needs?. Injury 2011; 42:Suppl 2 S56 - 63; http://dx.doi.org/10.1016/j.injury.2011.06.011; PMID: 21752369
- Wendt D, Marsano A, Jakob M, Heberer M, Martin I. Oscillating perfusion of cell suspensions through three-dimensional scaffolds enhances cell seeding efficiency and uniformity. Biotechnol Bioeng 2003; 84:205 - 14; http://dx.doi.org/10.1002/bit.10759; PMID: 12966577
- Griffith LG, Naughton G. Tissue engineering--current challenges and expanding opportunities. Science 2002; 295:1009 - 14; http://dx.doi.org/10.1126/science.1069210; PMID: 11834815
- Langer R. Tissue engineering. Mol Ther 2000; 1:12 - 5; http://dx.doi.org/10.1006/mthe.1999.0003; PMID: 10933907
- Godara P, McFarland CD, Nordon RE. Design of bioreactors for mesenchymal stem cell tissue engineering. J Chem Technol Biotechnol 2008; 83:408 - 20; http://dx.doi.org/10.1002/jctb.1918
- Bernhardt A, Lode A, Peters F, Gelinsky M. Optimization of culture conditions for osteogenically-induced mesenchymal stem cells in β-tricalcium phosphate ceramics with large interconnected channels. J Tissue Eng Regen Med 2010; 5:444 - 53; PMID: 20848550
- Martin I, Wendt D, Heberer M. The role of bioreactors in tissue engineering. Trends Biotechnol 2004; 22:80 - 6; http://dx.doi.org/10.1016/j.tibtech.2003.12.001; PMID: 14757042
- Meinel L, Karageorgiou V, Fajardo R, Snyder B, Shinde-Patil V, Zichner L, et al. Bone tissue engineering using human mesenchymal stem cells: effects of scaffold material and medium flow. Ann Biomed Eng 2004; 32:112 - 22; http://dx.doi.org/10.1023/B:ABME.0000007796.48329.b4; PMID: 14964727
- Botchwey E. A., Dupree MA, Pollack SR, Levine EM, Laurencin CT. Tissue engineered bone: Measurement of nutrient transport in three-dimensional matrices. J Biomed Mater Res A 2003; 67A:357 - 67; http://dx.doi.org/10.1002/jbm.a.10111
- Stiehler M, Bünger C, Baatrup A, Lind M, Kassem M, Mygind T. Effect of dynamic 3-D culture on proliferation, distribution, and osteogenic differentiation of human mesenchymal stem cells. J Biomed Mater Res A 2009; 89:96 - 107; PMID: 18431785
- Sailon AM, Allori AC, Davidson EH, Reformat DD, Allen RJ Jr., Warren SM. A novel flow-perfusion bioreactor supports 3D dynamic cell culture. J Biomed Biotechnol 2009; 2009:873816; http://dx.doi.org/10.1155/2009/873816; PMID: 20037739
- Carpentier B, Layrolle P, Legallais C. Bioreactors for bone tissue engineering. Int J Artif Organs 2011; 34:259 - 70; http://dx.doi.org/10.5301/IJAO.2011.6333; PMID: 21374561
- Kim HJ, Kim UJ, Leisk GG, Bayan C, Georgakoudi I, Kaplan DL. Bone regeneration on macroporous aqueous-derived silk 3-D scaffolds. Macromol Biosci 2007; 7:643 - 55; http://dx.doi.org/10.1002/mabi.200700030; PMID: 17477447
- Sikavitsas VI, Bancroft GN, Holtorf HL, Jansen JA, Mikos AG. Mineralized matrix deposition by marrow stromal osteoblasts in 3D perfusion culture increases with increasing fluid shear forces. Proc Natl Acad Sci U S A 2003; 100:14683 - 8; http://dx.doi.org/10.1073/pnas.2434367100; PMID: 14657343
- Sikavitsas VI, Temenoff JS, Mikos AG. Biomaterials and bone mechanotransduction. Biomaterials 2001; 22:2581 - 93; http://dx.doi.org/10.1016/S0142-9612(01)00002-3; PMID: 11519777
- Burdick JA, Vunjak-Novakovic G. Engineered microenvironments for controlled stem cell differentiation. Tissue Eng Part A 2009; 15:205 - 19; http://dx.doi.org/10.1089/ten.tea.2008.0131; PMID: 18694293
- Wang T-W, Wu H-C, Wang H-Y, Lin F-H, Sun J-S. Regulation fo adult human mesenchymal stem cells into osteogenic and chondrogenic lineages by different bioreactor systems. J Biomed Mater Res A 2007; 88A:935 - 46; http://dx.doi.org/10.1002/jbm.a.31914
- Goldstein AS, Juarez TM, Helmke CD, Gustin MC, Mikos AG. Effect of convection on osteoblastic cell growth and function in biodegradable polymer foam scaffolds. Biomaterials 2001; 22:1279 - 88; http://dx.doi.org/10.1016/S0142-9612(00)00280-5; PMID: 11336300
- Alvarez-Barreto JF, Linehan SM, Shambaugh RL, Sikavitsas VI. Flow perfusion improves seeding of tissue engineering scaffolds with different architectures. Ann Biomed Eng 2007; 35:429 - 42; http://dx.doi.org/10.1007/s10439-006-9244-z; PMID: 17216348
- Bancroft GN, Sikavitsas VI, Mikos AG. Design of a flow perfusion bioreactor system for bone tissue-engineering applications. Tissue Eng 2003; 9:549 - 54; http://dx.doi.org/10.1089/107632703322066723; PMID: 12857422
- Janssen FW, Oostra J, Oorschot A, van Blitterswijk CA. A perfusion bioreactor system capable of producing clinically relevant volumes of tissue-engineered bone: in vivo bone formation showing proof of concept. Biomaterials 2006; 27:315 - 23; http://dx.doi.org/10.1016/j.biomaterials.2005.07.044; PMID: 16125223
- Bjerre L, Bünger CE, Kassem M, Mygind T. Flow perfusion culture of human mesenchymal stem cells on silicate-substituted tricalcium phosphate scaffolds. Biomaterials 2008; 29:2616 - 27; http://dx.doi.org/10.1016/j.biomaterials.2008.03.003; PMID: 18374976
- Grayson WL, Bhumiratana S, Cannizzaro C, Chao PHG, Lennon DP, Caplan AI, et al. Effects of initial seeding density and fluid perfusion rate on formation of tissue-engineered bone. Tissue Eng Part A 2008; 14:1809 - 20; http://dx.doi.org/10.1089/ten.tea.2007.0255; PMID: 18620487
- Sucosky P, Osorio DF, Brown JB, Neitzel GP. Fluid mechanics of a spinner-flask bioreactor. Biotechnol Bioeng 2004; 85:34 - 46; http://dx.doi.org/10.1002/bit.10788; PMID: 14705010
- Vunjak Novakovic G, Freed LE, Biron RJ, Langer R. Effects of mixing on the composition and morphology of tissue engineered cartilage. AIChE J 1996; 42:850 - 60; http://dx.doi.org/10.1002/aic.690420323
- Datta N, Pham QP, Sharma U, Sikavitsas VI, Jansen JA, Mikos AG. In vitro generated extracellular matrix and fluid shear stress synergistically enhance 3D osteoblastic differentiation. Proc Natl Acad Sci U S A 2006; 103:2488 - 93; http://dx.doi.org/10.1073/pnas.0505661103; PMID: 16477044
- Bjerre L, Bünger C, Baatrup A, Kassem M, Mygind T. Flow perfusion culture of human mesenchymal stem cells on coralline hydroxyapatite scaffolds with various pore sizes. J Biomed Mater Res A 2011; 97:251 - 63; http://dx.doi.org/10.1002/jbm.a.33051; PMID: 21442726
- Mygind T, Stiehler M, Baatrup A, Li H, Zou X, Flyvbjerg A, et al. Mesenchymal stem cell ingrowth and differentiation on coralline hydroxyapatite scaffolds. Biomaterials 2007; 28:1036 - 47; http://dx.doi.org/10.1016/j.biomaterials.2006.10.003; PMID: 17081601
- Granet C, Laroche N, Vico L, Alexandre C, Lafage-Proust MH. Rotating-wall vessels, promising bioreactors for osteoblastic cell culture: comparison with other 3D conditions. Med Biol Eng Comput 1998; 36:513 - 9; http://dx.doi.org/10.1007/BF02523224; PMID: 10198539
- Qiu Q-Q, Ducheyne P, Ayyaswamy PS, Fabrication S. Fabrication, characterization and evaluation of bioceramic hollow microspheres used as microcarriers for 3-D bone tissue formation in rotating bioreactors. Biomaterials 1999; 20:989 - 1001; http://dx.doi.org/10.1016/S0142-9612(98)00183-5; PMID: 10378799
- Botchwey EA, Pollack SR, Levine EM, Johnston ED, Laurencin CT. Quantitative analysis of three-dimensional fluid flow in rotating bioreactors for tissue engineering. J Biomed Mater Res A 2004; 69:205 - 15; http://dx.doi.org/10.1002/jbm.a.10163; PMID: 15057993
- Botchwey EA, Pollack SR, Levine EM, Laurencin CT. Bone tissue engineering in a rotating bioreactor using a microcarrier matrix system. J Biomed Mater Res 2001; 55:242 - 53; http://dx.doi.org/10.1002/1097-4636(200105)55:2<242::AID-JBM1011>3.0.CO;2-D; PMID: 11255176
- Botchwey EA, Pollack SR, El-Amin S, Levine EM, Tuan RS, Laurencin CT. Human osteoblast-like cells in three-dimensional culture with fluid flow. Biorheology 2003; 40:299 - 306; PMID: 12454419
- Weinbaum S, Cowin SC, Zeng Y. A model for the excitation of osteocytes by mechanical loading-induced bone fluid shear stresses. J Biomech 1994; 27:339 - 60; http://dx.doi.org/10.1016/0021-9290(94)90010-8; PMID: 8051194
- Marolt D, Augst A, Freed LE, Vepari C, Fajardo R, Patel N, et al. Bone and cartilage tissue constructs grown using human bone marrow stromal cells, silk scaffolds and rotating bioreactors. Biomaterials 2006; 27:6138 - 49; http://dx.doi.org/10.1016/j.biomaterials.2006.07.015; PMID: 16895736
- Qiu Q-Q, Ducheyne P, Ayyaswamy PS. 3D bone tissue engineered with bioactive microspheres in simulated microgravity. In Vitro Cell Dev Biol Anim 2001; 37:157 - 65; http://dx.doi.org/10.1290/1071-2690(2001)037<0157:BTEWBM>2.0.CO;2; PMID: 11370806
- Terai H, Hannouche D, Ochoa E, Yamano Y, Vacanti JP. In vitro engineering of bone using a rotational oxygen-permeable bioreactor system. Mater Sci Eng C 2002; 20:3 - 8; http://dx.doi.org/10.1016/S0928-4931(02)00006-1
- Yoshimoto H, Shin YM, Terai H, Vacanti JP. A biodegradable nanofiber scaffold by electrospinning and its potential for bone tissue engineering. Biomaterials 2003; 24:2077 - 82; http://dx.doi.org/10.1016/S0142-9612(02)00635-X; PMID: 12628828
- Song K, Liu T, Cui Z, Li X, Ma X. Three-dimensional fabrication of engineered bone with human bio-derived bone scaffolds in a rotating wall vessel bioreactor. J Biomed Mater Res A 2008; 86:323 - 32; http://dx.doi.org/10.1002/jbm.a.31624; PMID: 17969035
- Grayson WL, Fröhlich M, Yeager K, Bhumiratana S, Chan ME, Cannizzaro C, et al. Engineering anatomically shaped human bone grafts. Proc Natl Acad Sci U S A 2010; 107:3299 - 304; http://dx.doi.org/10.1073/pnas.0905439106; PMID: 19820164
- Chen H-C, Hu Y-C. Bioreactors for tisue engineering. Biotechnol Lett 2006; 28:1215 - 9; http://dx.doi.org/10.1007/s10529-006-9111-x; PMID: 16799759
- Bancroft GN, Sikavitsas VI, van den Dolder J, Sheffield TL, Ambrose CG, Jansen JA, et al. Fluid flow increases mineralized matrix deposition in 3D perfusion culture of marrow stromal osteoblasts in a dose-dependent manner. Proc Natl Acad Sci U S A 2002; 99:12600 - 5; http://dx.doi.org/10.1073/pnas.202296599; PMID: 12242339
- Holtorf HL, Datta N, Jansen JA, Mikos AG. Scaffold mesh size affects the osteoblastic differentiation of seeded marrow stromal cells cultured in a flow perfusion bioreactor. J Biomed Mater Res A 2005; 74:171 - 80; http://dx.doi.org/10.1002/jbm.a.30330; PMID: 15965910
- Gomes ME, Sikavitsas VI, Behravesh E, Reis RL, Mikos AG. Effect of flow perfusion on the osteogenic differentiation of bone marrow stromal cells cultured on starch-based three-dimensional scaffolds. J Biomed Mater Res A 2003; 67:87 - 95; http://dx.doi.org/10.1002/jbm.a.10075; PMID: 14517865
- Wang Y, Uemura T, Dong J, Kojima H, Tanaka J, Tateishi T. Application of perfusion culture system improves in vitro and in vivo osteogenesis of bone marrow-derived osteoblastic cells in porous ceramic materials. Tissue Eng 2003; 9:1205 - 14; http://dx.doi.org/10.1089/10763270360728116; PMID: 14670108
- Holtorf HL, Sheffield TL, Ambrose CG, Jansen JA, Mikos AG. Flow perfusion culture of marrow stromal cells seeded on porous biphasic calcium phosphate ceramics. Ann Biomed Eng 2005; 33:1238 - 48; http://dx.doi.org/10.1007/s10439-005-5536-y; PMID: 16133930
- Porter BD, Lin ASP, Peister A, Hutmacher D, Guldberg RE. Noninvasive image analysis of 3D construct mineralization in a perfusion bioreactor. Biomaterials 2007; 28:2525 - 33; http://dx.doi.org/10.1016/j.biomaterials.2007.01.013; PMID: 17258311
- Sikavitsas VI, Bancroft GN, Lemoine JJ, Liebschner MA, Dauner M, Mikos AG. Flow perfusion enhances the calcified matrix deposition of marrow stromal cells in biodegradable nonwoven fiber mesh scaffolds. Ann Biomed Eng 2005; 33:63 - 70; http://dx.doi.org/10.1007/s10439-005-8963-x; PMID: 15709706
- Gomes ME, Reis RL, Mikos AG. Bone tissue engineering constructs based on starch scaffolds and bone marrow cells cultured in a flow perfusion bioreactor. Trans Tech Publ 2006; 2006:980 - 4
- Sikavitsas VI, Bancroft GN, Lemoine JJ, Liebschner MA, Dauner M, Mikos AG. Flow perfusion enhances the calcified matrix deposition of marrow stromal cells in biodegradable nonwoven fiber mesh scaffolds. Ann Biomed Eng 2005; 33:63 - 70; http://dx.doi.org/10.1007/s10439-005-8963-x; PMID: 15709706
- Alvarez-Barreto JF, Sikavitsas VI. Improved mesenchymal stem cell seeding on RGD-modified poly(L-lactic acid) scaffolds using flow perfusion. Macromol Biosci 2007; 7:579 - 88; http://dx.doi.org/10.1002/mabi.200600280; PMID: 17457938
- Janssen FW, Hofland I, van Oorschot A, Oostra J, Peters H, van Blitterswijk CA. Online measurement of oxygen consumption by goat bone marrow stromal cells in a combined cell-seeding and proliferation perfusion bioreactor. J Biomed Mater Res A 2006; 79:338 - 48; http://dx.doi.org/10.1002/jbm.a.30794; PMID: 16878315
- Cartmell SH, Porter BD, Garciáa AJ, Guldberg RE. Effects of medium perfusion rate on cell-seeded three-dimensional bone constructs in vitro. Tissue Eng 2003; 9:1197 - 203; http://dx.doi.org/10.1089/10763270360728107; PMID: 14670107
- Jaasma MJ, Plunkett NA, O’Brien FJ. Design and validation of a dynamic flow perfusion bioreactor for use with compliant tissue engineering scaffolds. J Biotechnol 2008; 133:490 - 6; http://dx.doi.org/10.1016/j.jbiotec.2007.11.010; PMID: 18221813
- Cioffi M, Boschetti F, Raimondi MT, Dubini G. Modeling evaluation of the fluid-dynamic microenvironment in tissue-engineered constructs: a micro-CT based model. Biotechnol Bioeng 2006; 93:500 - 10; http://dx.doi.org/10.1002/bit.20740; PMID: 16224789
- Grayson WL, Marolt D, Bhumiratana S, Fröhlich M, Guo XE, Vunjak-Novakovic G. Optimizing the medium perfusion rate in bone tissue engineering bioreactors. Biotechnol Bioeng 2011; 108:1159 - 70; http://dx.doi.org/10.1002/bit.23024; PMID: 21449028
- Hidalgo-Bastida LA, Thirunavukkarasu S, Griffiths S, Cartmell SH, Naire S. Modeling and design of optimal flow perfusion bioreactors for tissue engineering applications. Biotechnol Bioeng 2012; 109:1095 - 9; http://dx.doi.org/10.1002/bit.24368; PMID: 22068720
- White FM. Viscous fluid flow. McGraw-Hill New York, 1991.
- van den Dolder J, Bancroft GN, Sikavitsas VI, Spauwen PHM, Jansen JA, Mikos AG. Flow perfusion culture of marrow stromal osteoblasts in titanium fiber mesh. J Biomed Mater Res A 2003; 64:235 - 41; http://dx.doi.org/10.1002/jbm.a.10365; PMID: 12522809
- Du D, Furukawa K, Ushida T. Oscillatory perfusion seeding and culturing of osteoblast-like cells on porous beta-tricalcium phosphate scaffolds. J Biomed Mater Res A 2008; 86:796 - 803; http://dx.doi.org/10.1002/jbm.a.31641; PMID: 18041721
- Du D, Furukawa KS, Ushida T. 3D culture of osteoblast-like cells by unidirectional or oscillatory flow for bone tissue engineering. Biotechnol Bioeng 2009; 102:1670 - 8; http://dx.doi.org/10.1002/bit.22214; PMID: 19160373
- Jagodzinski M, Breitbart A, Wehmeier M, Hesse E, Haasper C, Krettek C, et al. Influence of perfusion and cyclic compression on proliferation and differentiation of bone marrow stromal cells in 3-dimensional culture. J Biomech 2008; 41:1885 - 91; http://dx.doi.org/10.1016/j.jbiomech.2008.04.001; PMID: 18495131
- Kavlock KD, Goldstein AS. Effect of pulse frequency on the osteogenic differentiation of mesenchymal stem cells in a pulsatile perfusion bioreactor. J Biomech Eng 2011; 133:091005; http://dx.doi.org/10.1115/1.4004919; PMID: 22010740
- Sharp LA, Lee YW, Goldstein AS. Effect of low-frequency pulsatile flow on expression of osteoblastic genes by bone marrow stromal cells. Ann Biomed Eng 2009; 37:445 - 53; http://dx.doi.org/10.1007/s10439-008-9632-7; PMID: 19130228
- Jaasma MJ, O’Brien FJ. Mechanical stimulation of osteoblasts using steady and dynamic fluid flow. Tissue Eng Part A 2008; 14:1213 - 23; http://dx.doi.org/10.1089/ten.tea.2007.0321; PMID: 18433309