Abstract
Human pluripotent stem (hPS) cells provide an attractive opportunity for the manufacture of a wide array of therapeutic cell types. The challenges to commercialization include the thousand-fold diversity of cell types emerging from hPS cells and the associated difficulties in validating processes to reliably generate cells with precise identity and purity. Improved methods of controlling the dosage and migration of hPS-derived cells in solid tissues are also needed. To directly address these issues, we clonally expanded proliferating lineages of cells that were intermediate in regard to their state of differentiation between hPS and terminally differentiated cells. These cells called monoclonal embryonic progenitors (hEP), are expandable mortal lineages with diverse site-specific homeobox gene expression and multipotentiality. In this review, we discuss methods of generating combination products wherein the fate space of precisely identified monoclonal hEP cells is mapped by differentiating the cells in vitro in HyStem-3D bead arrays in the presence of diverse growth factors. This combination of discovery processes has the potential to translate directly into cell-matrix formulations that can be used to generate pre-clinical data leading to human clinical trials and potentially new medical therapies.
Introduction
The rising prevalence of age-related degenerative disease in many countries is triggering significant interest in the development of novel cell-based therapies to regenerate tissue function—a prospect that has long escaped the capabilities of drug and antibody-based therapeutic strategies.Citation1 Of particular interest is the use of human pluripotent stem (hPS) cells such as human embryonic stem (hES) and induced pluripotent stem (iPS) cells to generate the diverse cellular components of the human body on an industrial scale for use in therapy. Pluripotent stem cell technologies offer at least two distinct advantages over previous approaches to the manufacture of living-cell therapies. First, pluripotent stem cells in the undifferentiated state are capable of indefinite proliferation due to the abundant expression of the telomerase catalytic component TERT.Citation2 This allows a manufacturing scheme wherein master cell banks of hPS cells are precisely characterized and used for the indefinite manufacture of differentiated cell types consistent with good manufacturing practices (GMP). The second advantage of the pluripotent stem cell platform is the ability of these cells to differentiate into all somatic cell lineages.Citation3
In the adult human, certain cells types are proposed to have multipotency; such cells include hematopoietic stem cells (HSCs), bone marrow-derived mesenchymal stem cells (MSCs), and brain subventricular zone neuronal stem cells (NSCs). However, no adult stem cell sources have been identified to date that are effective at regenerating most vital tissues of the bodyCitation4 and adult stem cell cultures typically have limited scale up potential. Taken together, the unique capacity of hPS cells to be expanded in the immortal state while maintaining long telomere length, as well as the ability of hPS cells to cascade into all body cell types are leading to the increasing interest in pluripotent stem cells.
Challenges of Pluripotency
While hPS cells offer these exciting prospects, there remain significant challenges to making these products compatible for human clinical use. As opposed to multipotent cells, such as HSCs or MSCs, hPS cells and their immediate derivatives are: (1) unique in displaying the potential to differentiate into derivatives of all three embryonic germ layers (a complexity of > 1,000-fold), (2) are often highly proliferative, (3) appear to occasionally express regenerative patterns of gene expression lost in subsequent fetal and adult tissues, and (4) and can generate both parenchymal and associated stromal cell types capable of the cross-talk required to generate tissue structures.
The above complexity is at once as much a challenge to researchers as it is an opportunity. The opportunity is obvious, the challenge is often overlooked. Formulations of targeted differentiated cells derived from hPS cells may, for instance, contain contaminating cell types, many of which are currently uncharacterized, resulting in unpredictable ectopic tissues developing at the site of engraftment. While most of the undesired ectopic tissues reported appear to be benign (cystic structure and cartilage), they may not manifest during the relatively short period of animal model preclinical testing, and therefore, their full risk to a human patient over years or decades is currently unknown. Similarly, the manifestation of such ectopic cells may require higher dosages than is routine or even possible in animal models, but required for efficacy in humans. Lastly, human hPS-derived formulations may respond differently in a human host than non-human animal, or they may simply require greater numbers of patients before the nature and frequency of adverse events became clear. As a result of these concerns, successful clinical development may necessitate increased standards of purity, identity, and control over migration to reasonably ensure the safety of human patients.
hPS-Derived Monoclonal Embryonic Progenitor Cell Lines
The problems of cellular contamination in current hPS cell differentiation protocols are a result of the processes currently in use. Most published strategies currently utilized in industry and academia carry out the propagation of hPS cells as the primary scale-up point (). The expanded hPS cells are subsequently differentiated under a wide array of protocols (http://discovery.lifemapsc.com) to generate enriched populations of differentiated cells. From these heterogeneous mixtures of differentiated cells, desired cell types are purified by varied protocols such as affinity purification. Then, a series of quality assays are performed to measure the degree of purification, including screening for the presence of residual hPS cells as a measure of the potential for teratoma formation, and assays to measure the potency of the target cell types. These manufacturing protocols are designated as “heterogeneous,” since the raw product prior to purification contains an indeterminate number of diverse cellular contaminants. Remarkably, there are currently no known methods of identifying these cellular contaminants from among the thousand-fold diversity of possible cell types emerging from hPS cells, and this deficiency in assaying for problematic contaminants compromises the ability to effectively purify out and assay for the cells. The impurity of these products, and the associated costs of attempting to further purify them and/or to demonstrate the safety of the impure formulations in very large numbers of models has historically been a significant challenge to the industry.
Figure 1. Heterogeneous differentiation of hPS cells vs. the clonal expansion of hEPs as a means of manufacturing therapeutic products. (A) With heterogeneous differentiation, hPS cells such as hES cells are scaled in the undifferentiated immortal state, exposed to a differentiation protocol, and the desired cell type is purified from the heterogeneous mixture. (B) Clonal lines are generated from partially differentiated hPS cells, the clonal lines are expanded in a mortal state, then terminally differentiated for research or therapeutic use.
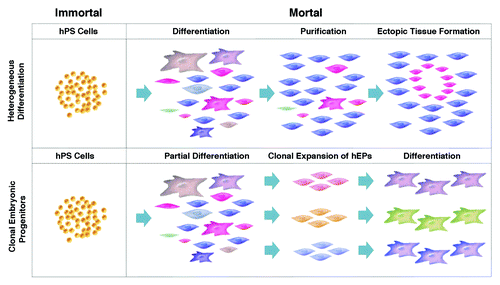
In addition, heterogeneous differentiation protocols typically result in poor lot-to-lot reproducibility, since they typically involve numerous temporally oriented differentiation steps. With each fate decision, there is a cumulative potential that the cells will differentiate into an undesired cell lineage rather than into the intended cell type. When the multiple differentiation steps are linked, the probability of obtaining a product lot of cells of the intended differentiated state is often relatively low. Thus, efforts are ongoing to map the gene expression markers of all cells derived from pluripotent stem cells, which would potentially provide more reliable differentiation protocols as well as more definition of cell-surface antigens to improve the affinity purification process (http://discovery.lifemapsc.com). In the meantime, there is an urgent need to identify alternative protocols to enable a rapid, reliable, and economic scale-up process of hPS-derived cells that meet the regulatory criteria of purity and identity.
One such alternative strategy is the clonal expansion of cells intermediate in their differentiated state between hPS cells and terminally differentiated cells. These intermediate cells are designated human embryonic progenitor (hEP) cells (trade named PureStem™ progenitors http://discovery.lifemapsc.com). In vivo examples of hEP cells would be the neuroepithelial cells of the neural tube, propagating and migrating neural crest in the cranial mesenchyme, paraxial mesoderm and lateral plate limb bud mesenchyme. Like hPS cells, monoclonal embryonic progenitor cell lines do not necessarily correspond to stem cells in vivo, capable of propagating in a niche while maintaining their relatively undifferentiated state, or making fate decisions to differentiate. In that sense, technically, hES cells are not “stem cells,” but rather reflect an in vitro artifact, or stasis, wherein the culture of the cells in particular conditions results in the expansion of the cell in number without further downstream differentiation. Thus, an account of the propagation of monoclonal embryonic progenitor cell lines may rest instead with the possibility that both hPS and hEP cell lines represent cells that are propagating within developmental stasis; in other words, neither type of cell progresses to differentiation due to the lack of a requisite signal.
According to the hEP cell protocol, hPS cells are not initially scaled up, but instead are partially differentiated under a variety of differentiation conditions to obtain heterogeneous cultures of hEP cells. From these heterogeneous cultures, hPS cells are clonally isolated and expanded under a combinatorial array of propagation conditions (). An initial “shotgun cloning” of hEP cells demonstrated that the resulting cells, if continually propagated in their relatively undifferentiated state, will exhibit a broad diversity of gene expression profiles, including diverse site-specific homeobox gene expression and an estimated diversity of 140 distinct cell types within the 242 cell lines tested.Citation5 These hEP cells typically display a uniform morphology, high levels of mitotic activity, and appear to be telomerase negative (mortal). But since most hES cell lines, when properly cultivated, maintain telomeres at a long and stable length, the clonal progenitor cells in our hands are often capable of clonal expansion from a single initial cell into stable cultures (approximately 20 doublings to generate one million cells), as well as capable of further expansion to create master and working cell banks, and direct scale-up to produce > 1011 cells before the cultures become impaired by telomere shortening (replicative senescence). The hEP cell line 4D20.8, for example, remains multipotent up to passage 33,Citation6 far in excess of the limited capacity to propagate bone marrow-derived MSCs before losing chondrogenic potential. At late passage (passage 38) copy number variations are detected including a trisomy in chromosome 16 and a monosomy in chromosome 17, similar to that reported in long-term cultures of hES cells. However, at earlier passages, the cells displayed only minor variations common to all cultured cells. In the case of a more limited scale up of this line, for instance to a maximum of passage 30, it would be possible to generate an estimated 10 billion doses of 100 million cells for potential therapeutic use from only one existing clonal cell line. Therefore, clonally-purified hEP cells can be directly expanded, cryopreserved, thawed and expanded again as a point of scale up as opposed to the scale up of hPS cells typically planned in the case of heterogeneous differentiation protocols.
Defining an Optimum Cell Transplantation Matrix
The use of clonal and expandable hEP cells may provide a means of manufacturing diverse types of human progenitors from the hPS cell platform in vitro, however, the ultimate goal is to define the dosage and potency of cells engrafted in vivo. Ideally, the cells would be co-developed with an injectable matrix that would improve the reliability of survival of the engrafted cells by providing key cell attachment sites as well as a hyaluronate-rich environment similar to that prevalent in early embryonic development.Citation7,Citation8 This co-development would increase the understanding of the effectiveness (potency) of the cells in the defined matrix. HyStem-C hydrogels are an example of such a matrix.Citation9,Citation10 Composed of thiol-modified gelatin and thiolated hyaluronan crosslinked with (polyethylene glycol diacrylate (PEGDA), HyStem-C hydrogels appear to increase the reliability of cell viability in diverse target tissues such as myocardium,Citation11 brain,Citation12 vocal cordsCitation13 and adipose tissue.Citation14 HyStem-C also appears to be capable of safely crosslinking in vivo to potentially anchor the introduced cells at the injection site.Citation15 We therefore undertook studies to determine whether this matrix could be utilized in screening the differentiation potential of hEP cell lines to streamline the translation from bench top experiments to relevant animal in vivo transplantation studies.
In vitro Testing of hEP Cell-Matrix Combination Products: Fate Space Screening
To map the fate space of the diverse clonal hEP cell lines, we utilize two methods to generate high-density cultures, thereby predisposing hEP cells to differentiation in the presence of exogenous factors. The first approach, commonly referred to as micromass differentiation,Citation16 is a system wherein 10 μl aliquots of 2.0 × 107 cells/mL (200,000 cells/micromass) are plated in growth medium to allow for attachment, then the micromasses are incubated in a differentiation cocktail. The second approach, referred to herein as HyStem-4D bead arrays, is a system by which high density is achieved with the plating of 25 μl aliquots of 2.0 × 107 cells/mL in 1% w/v HyStem-C (500,000 cells/construct),Citation6 then following polymerization into hemispherical beads arrayed to the tissue culture dish, the constructs are similarly exposed to growth/differentiation factors.
As shown in , micromass conditions provide a relatively heterogeneous environment in which a subset of cells are typically observed spreading at the edges of the mass, while the majority are embedded in a dense mound. Immunocytochemical staining of the micromasses with antibodies directed to antigens expected in the micromasses, often show evidence of heterogeneity, such as different differentiation pathways taken in the peripheral cells than the majority of cells in the micromass (not shown). In contrast, the cells embedded in HyStem-4D bead arrays () in our experience, typically appear to be more uniformly dispersed in a three-dimensional matrix, and allow the additional temporal dimension of extended incubation or cryopreservation (the fourth dimension hence the designation “-4D”). The more uniform constructs generated using HyStem-4D bead arrays combined with the potential to directly translate the combination product into preclinical animal models wherein the same hydrogel is designed to increase viable engraftment and prevent undesired migration, may therefore provide significant advantages of the beads compared with micromass differentiation for in vitro discovery research.
Figure 2. Phase-contrast photographs of a representativ hEP cell line micromass and HyStem-4D bead used in fate-space screening A. hEP cell micromass from the cell line T42 cultured in the presence of BMP4. B. hEP cell HyStem-4D bead constructs from the cell line T42 cultured in the presence of BMP4. (Scale bar, 100 microns).
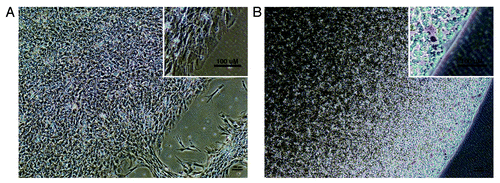
The scalability and the ability to freeze and thaw clonal hEP cell lines allows the lines to be reproducibly and reliably differentiated in either micromass of HyStem-4D beads in diverse differentiation conditions in replicate. As shown in , these differentiation conditions may include members of the TGFβ, FGF, and Wnt-signaling modifier families, retinoic acid, or other factors and combinations of these factors for varied periods of time, typically two or three weeks. RNA is then prepared from the cells, and the transcriptome is then assessed by a variety of methods including qPCR or gene expression microarrays (e.g., Illumina human HT-12 v4 beadchip arrays) and compared with the original undifferentiated cells and to the other differentiated cell lines in order to detect evidence of genes that are differentially expressed.
Figure 3. Strategy of fate-space screening. Diverse clonal hEP cell lines are cultured in high density conditions such as micromasses or HyStem-4D bead arrays in the presence of diverse differentiation conditions such as physiological concentrations of TGFβ family members, FGFs, retinoic acid, and modulators of Wnt signaling. After 14–21 d, RNA is analyzed by gene expression microarrays.
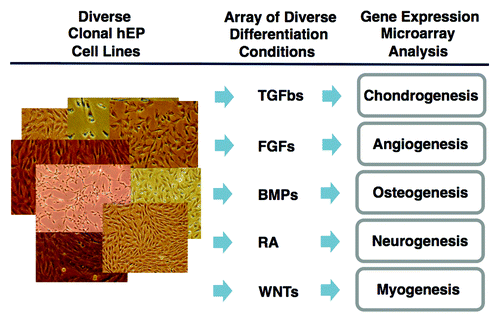
When 100 diverse clonal hEP cell lines were thawed and then plated under high density micromass conditions in the presence of TGFβ3, dexamethasone, and ITS for 14 d, seven of the lines were observed to undergo chondrogenesis as judged by the abundant upregulation of collagen type II, α I (COL2A1) transcript and related chondrocyte markers.Citation6 The seven lines displayed distinctive site-specific homeobox gene expression in the undifferentiated progenitor state, including differential expression of markers such as BARX1, HOXB2, LHX1, LHX8, MSX2, PITX1 and TBX15. Expanded fate-space mapping of these seven lines in HyStem-4D bead arrays in combinations of TGFβ family members showed induction of diverse differentiation markers corresponding to the diverse site-specific origins of the clones, including varied expression of the cartilage markers COL2A1 and CRTAC1,Citation17 osteogenic markers IBSP and ALPL, and the tendon/ligament marker TNMD.Citation18,Citation19
LHX8 is a homeobox gene associated with perioral mesenchyme.Citation20,Citation21 When the LHX8+ BARX1+ cell line 4D20.8 with the most distal HOX gene expression of HOXB2 was screened in HyStem-4D bead arrays using diverse combinations of TGFβ family members, the line showed higher COL2A1 and CRTAC1 expression than early passage mesenchymal stem cells (MSCs) and markedly lower expression of the hypertrophic marker COL10A1 when differentiation occurred in a cocktail containing both TGFβ3 and GDF5. MSCs typically differentiate in vitro in a manner mirroring their role in the growth plate and healing bone fractures in vivo. Differentiating MSCs often express markers of hypertrophic cartilage which provides a transient cartilaginous matrix that degrades and attracts vasculature and associated osteoblasts and bone formation.Citation22-Citation24 This hypertrophic phenotype of MSCs complicates the potential use of MSCs in regenerating definitive chondrocytes in at the joint surface or intervertebral disc. The discovery that clonal hEP cell lines such as 4D20.8, unlike MSCs, are capable of responding to the presence of TGFβ3 and GDF5 in HyStem-4D beads by differentiating with markedly lower levels of markers of hypertrophy, is consistent with the putative role of GDF5 in inducing interzone formation and articular cartilage from embryonic mesenchyme.Citation25,Citation26 It also highlights the unique properties of embryonic progenitor compared with adult mesenchymal cells, as well as differences that may be utilized for therapeutic effect. Indeed, parallel transplantation of 4D20.8 and MSCs formulated in HyStem-C into rat femoral joint defects demonstrated that while MSCs differentiate into poorly organized fibrous tissue and bone, the line 4D20.8 led to regenerating subchondral bone and surface articular cartilage approximating the original joint architecture.Citation6
Histological and mechanical analysis of these chondrogenic hEP cell lines differentiated in combinations of TGFβ family members in HyStem-C constructs was also performed. For example, the hEP cell line E15 at passage 19 was differentiated for 14 d in HyStem constructs in the presence of 100 ng/ml of BMP7 and 10 ng/ml of TGFβ3. The results show hematoxylin and eosin (H&E) and Safranin-O staining as expected for cartilage differentiation ( and B respectively, previously unpublished data).
Figure 4. Representative histology of differentiated HyStem-C constructs in vitro and tracking of human hEP-derived differentiated cells in HyStem-C in tissue sections. The hEP cell line E15 (P19) was differentiated for 14 d in HyStem-C in vitro supplemented with TGFβ3 and BMP7. (A) H&E staining of differentiated E15/HyStem-C construct, (B) Safranin-O staining of differentiated E15/HyStem-C construct, (C) The cell line 4D20.8 was formulated in HyStem-C and implanted in vivo into a rat femoral defect for four weeks and transplanted cells were localized with anti-human mitochondrial antibody and H&E staining (red arrows).
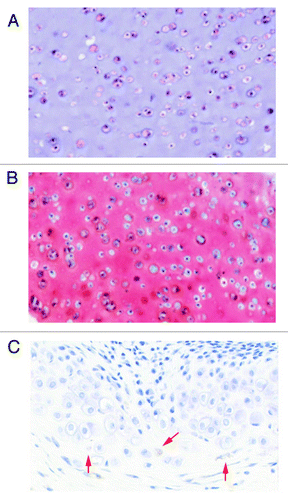
While we observed that hEP cell lines can be successfully differentiated in alternative injectable matrices such as calcium alginate beads, we have observed that transplantation of the cells in vivo in alginate is problematic in that alginate stains brightly with multiple reagents including Safranin-O. While HyStem-C stains slightly with Safranin-O stain, we found that cells transplanted in vivo in HyStem-C were easily stained by H&E and Safranin-O, and could be localized in the tissue with human-specific antibody. For example, as shown in , the chondrogenic progenitor 4D20.8 transplanted in HyStem-C in vivo into femoral trochlear defects, could be easily tracked in tissue sections using anti-human mitochondrial antibody.
To determine whether this cell-matrix combination can also be used to generate tissue engineered cartilage constructs useful in measuring mechanical strength parameters, 5 x 107 cells/ml were incorporated into 4mm HyStem discs with and without collagen for three or six weeks.Citation6 In the presence of TGFβ3 and BMP7, for example, the hEP cells designated 4D20.8 at six weeks showed an equilibrium modulus of 9.6 kPa while the cells in the presence of TGFβ3 and GDF5 at the same time point showed an equilibrium modulus of 29.2 kPa. The dynamic modulus observed in the presence of TGFβ3 and BMP7 was 824 kPa and that in TGFβ3 and GDF5 at the same time point was 1311 kPa. These observations replicated in other chondrogenic hEP cell lines suggest that various in vitro modeling can also be performed in the clonal hEP cell lines in the same matrix planned for in vivo application.
HyStem-4D bead arrays not only allowed for improved definition of the fate space of these novel clonal hEP cell lines, it documented their ability to differentiate in HyStem-C, and enabled the accumulation of a large amount of data on the biological influence of HyStem-C on diverse cell types. With Illumina gene expression microarray data from more than 3,000 differentiation experiments, we searched for genes frequently up- and downregulated in HyStem-4D beads and compared those profiles to those obtained under micromass conditions. For example, we observed that cells cultured in HyStem-4D beads with BMP4 frequently exhibited a marked decrease in myofibroblast markers such as MYH11, and increased expression of adipocyte markers such as FABP4 and anti-inflammatory markers such as TIMP4. A representative experiment is shown in . The cell line E15, which in other conditions was shown to have chondrogenic potentialCitation19 and the line W10Citation27 strongly induced MYH11 in micromass conditions supplemented with 10 ng/mL BMP4, but this induction was essentially ablated in HyStem-4D culture supplemented with BMP4. Instead, in HyStem-4D beads, the line markedly upregulated expression of DCN, a marker of meninges.Citation28 This physiological effect on myofibroblastic differentiation seen in many lines cultured in HyStem-4D beads (i.e., the strong reduction in MYH11 expression) may have therapeutic implications in vivo, such as in inhibiting fibrosis or adhesions.Citation29 This may also be of benefit in surgical settings where cells could be transplanted to regenerate tissue function while inhibiting adhesions and related fibrotic process at the surgical site.
Figure 5. Relative expression levels of MYH11, FABP4, DCN, and TIMP4 in hEP cell lines differentiated in BMP4 in both micromass and HyStem-4D bead arrays. Expression levels of MYH11 (solid black), FABP4 (stippled), DCN (gray) and TIMP4 (cross-hatched) are shown for the hEP cell lines E15, E69, T42, and W10 in both micromass and HyStem-4D bead arrays both being supplemented with BMP4. (RFUs, relative fluorescence units; RFU values of < 100 considered as background signal). Cntl, Control; MM, micromass; HS, HyStem-C constructs.
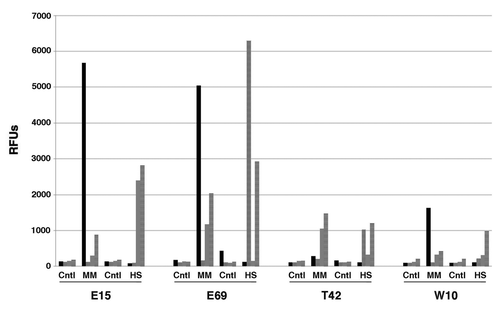
In addition to decreasing myofibroblast gene expression, the culture of hEP cells in HyStem-4D beads and BMP4, as opposed to micromasses and BMP4, frequently showed an upregulation of adipocyte differentiation markers as shown for the cell line E69 in . These observations support the view that co-development of hPS-derived cells and matrices in combination early in the discovery process may provide important information on gene expression, dosage, and viability that would be useful in designing optimal animal preclinical models, thereby streamlining product development.
Summary
The increasingly rigorous standards imposed by regulatory agencies for the approval of cellular therapeutics, and the requisite financial costs involved, demands a fresh perspective on the process development strategies of manufacturing products from hPS cell sources. The combination of in vivo crosslinkable hydrogels such as HyStem-C together with clonally-purified hPS-derived EP cell lines may simplify and improve the economics of the scalable manufacture of highly identified and purified cell types with improved standards of viability and immobilization post-implant.
While hPS cells possess the advantage of indefinite scalability, hPS-derived clonal EP cells have suitable proliferative lifespan for widespread therapeutic applications. The use of a single culture of scaled clonal EP cells may allow, for example, the manufacture of very large product lot sizes capable of delivering, for instance, millions of doses of 100 million cells. Since it is also possible to generate additional clonal lines from the frozen stocks of the heterogeneous cultures from which the clones were initially isolated, very large numbers of cells from expanded hEP cell lines is easily achievable.
In contrast to the use of clonal hEP cells, the process of heterogeneous differentiation results in impure populations of cells with contaminating and uncharacterized embryonic progenitor cells. Because such lots of cells vary from lot-to-lot, it is only feasible to test the behavior and gene expression profiles of these cells in candidate matrices after the fact. This may lead to uncertainty regarding the behavior of the cells in the novel matrix, and the potential clonal expansion of the contaminating progenitor cells to form ectopic tissues.
In summary, there appear to be at least two advantages the use of clonal hEP cell lines in HyStem-4D bead arrays. First, stable and expandable clonal hEP cell lines may provide an improved method of reliably generating diverse, purified, and scalable human embryonic cell types. Second, HyStem-4D bead arrays may provide a more uniform high density system compared with micromass differentiation. In addition, discoveries in HyStem-C may lead to a more direct translation into formulations suited for human transplantation since HyStem-C is capable of safely crosslinking with cells in vivo. Together, clonal hEP cell lines formulated in HyStem-C may accelerate the pace of translation from discovery to the use of the combined products in preclinical and clinical testing.
References
- zur Nieden NI. Embryonic stem cells for osteo-degenerative diseases. Methods Mol Biol 2011; 690:1 - 30; http://dx.doi.org/10.1007/978-1-60761-962-8_1; PMID: 21042982
- Funk WD, Labat I, Sampathkumar J, Gourraud PA, Oksenberg JR, Rosler E, et al. Evaluating the genomic and sequence integrity of human ES cell lines; comparison to normal genomes. Stem Cell Res 2012; 8:154 - 64; http://dx.doi.org/10.1016/j.scr.2011.10.001; PMID: 22265736
- Thomson JA, Itskovitz-Eldor J, Shapiro SS, Waknitz MA, Swiergiel JJ, Marshall VS, et al. Embryonic stem cell lines derived from human blastocysts. Science 1998; 282:1145 - 7; http://dx.doi.org/10.1126/science.282.5391.1145; PMID: 9804556
- Wagers AJ, Weissman IL. Plasticity of adult stem cells. Cell 2004; 116:639 - 48; http://dx.doi.org/10.1016/S0092-8674(04)00208-9; PMID: 15006347
- West MD, Sargent RG, Long J, Brown C, Chu JS, Kessler S, et al. The ACTCellerate initiative: large-scale combinatorial cloning of novel human embryonic stem cell derivatives. Regen Med 2008; 3:287 - 308; http://dx.doi.org/10.2217/17460751.3.3.287; PMID: 18462054
- Sternberg H, Murai JT, Erickson IE, Funk WD, Das S, Wang Q, et al. A human embryonic stem cell-derived clonal progenitor cell line with chondrogenic potential and markers of craniofacial mesenchyme. Regen Med 2012; 7:481 - 501; http://dx.doi.org/10.2217/rme.12.29; PMID: 22519755
- Estes JM, Adzick NS, Harrison MR, Longaker MT, Stern R. Hyaluronate metabolism undergoes an ontogenic transition during fetal development: implications for scar-free wound healing. J Pediatr Surg 1993; 28:1227 - 31; http://dx.doi.org/10.1016/S0022-3468(05)80303-3; PMID: 8263679
- Matsumoto K, Li Y, Jakuba C, Sugiyama Y, Sayo T, Okuno M, et al. Conditional inactivation of Has2 reveals a crucial role for hyaluronan in skeletal growth, patterning, chondrocyte maturation and joint formation in the developing limb. Development 2009; 136:2825 - 35; http://dx.doi.org/10.1242/dev.038505; PMID: 19633173
- Zheng Shu X, Liu Y, Palumbo FS, Luo Y, Prestwich GD. In situ crosslinkable hyaluronan hydrogels for tissue engineering. Biomaterials 2004; 25:1339 - 48; http://dx.doi.org/10.1016/j.biomaterials.2003.08.014; PMID: 14643608
- Prestwich GD. Hyaluronic acid-based clinical biomaterials derived for cell and molecule delivery in regenerative medicine. J Control Release 2011; 155:193 - 9; http://dx.doi.org/10.1016/j.jconrel.2011.04.007; PMID: 21513749
- Cheng K, Blusztajn A, Shen D, Li TS, Sun B, Galang G, et al. Functional performance of human cardiosphere-derived cells delivered in an in situ polymerizable hyaluronan-gelatin hydrogel. Biomaterials 2012; 33:5317 - 24; http://dx.doi.org/10.1016/j.biomaterials.2012.04.006; PMID: 22560668
- Zhong J, Chan A, Morad L, Kornblum HI, Fan G, Carmichael ST. Hydrogel matrix to support stem cell survival after brain transplantation in stroke. Neurorehabil Neural Repair 2010; 24:636 - 44; http://dx.doi.org/10.1177/1545968310361958; PMID: 20424193
- Bartlett RS, Thibeault SL, Prestwich GD. Therapeutic potential of gel-based injectables for vocal fold regeneration. Biomed Mater 2012; 7:024103; http://dx.doi.org/10.1088/1748-6041/7/2/024103; PMID: 22456756
- Flynn L, Prestwich GD, Semple JL, Woodhouse KA. Adipose tissue engineering in vivo with adipose-derived stem cells on naturally derived scaffolds. J Biomed Mater Res A 2009; 89:929 - 41; http://dx.doi.org/10.1002/jbm.a.32044; PMID: 18465826
- Prestwich GD, Erickson IE, Zarembinski TI, West M, Tew WP. The translational imperative: making cell therapy simple and effective. Acta Biomater 2012; 8:4200 - 7; http://dx.doi.org/10.1016/j.actbio.2012.06.043; PMID: 22776825
- Johnstone B, Hering TM, Caplan AI, Goldberg VM, Yoo JU. In vitro chondrogenesis of bone marrow-derived mesenchymal progenitor cells. Exp Cell Res 1998; 238:265 - 72; http://dx.doi.org/10.1006/excr.1997.3858; PMID: 9457080
- Steck E, Benz K, Lorenz H, Loew M, Gress T, Richter W. Chondrocyte expressed protein-68 (CEP-68), a novel human marker gene for cultured chondrocytes. Biochem J 2001; 353:169 - 74; http://dx.doi.org/10.1042/0264-6021:3530169; PMID: 11139377
- Brandau O, Meindl A, Fässler R, Aszódi A. A novel gene, tendin, is strongly expressed in tendons and ligaments and shows high homology with chondromodulin-I. Dev Dyn 2001; 221:72 - 80; http://dx.doi.org/10.1002/dvdy.1126; PMID: 11357195
- Sternberg H, et al. Seven diverse human embryonic stem cell-derived chondrogenic clonal embryonic progenitor cell lines display site-specific cell fates. Regen Med 2012; http://dx.doi.org/10.2217/rme.12.29; PMID: 23249126
- Grigoriou M, Tucker AS, Sharpe PT, Pachnis V. Expression and regulation of Lhx6 and Lhx7, a novel subfamily of LIM homeodomain encoding genes, suggests a role in mammalian head development. Development 1998; 125:2063 - 74; PMID: 9570771
- Zhang Y, Mori T, Takaki H, Takeuch M, Iseki K, Hagino S, et al. Comparison of the expression patterns of two LIM-homeodomain genes, Lhx6 and L3/Lhx8, in the developing palate. Orthod Craniofac Res 2002; 5:65 - 70; http://dx.doi.org/10.1034/j.1600-0544.2002.02198.x; PMID: 12086327
- Grant WT, Wang GJ, Balian G. Type X collagen synthesis during endochondral ossification in fracture repair. J Biol Chem 1987; 262:9844 - 9; PMID: 3597444
- Gebhard S, Hattori T, Bauer E, Bösl MR, Schlund B, Pöschl E, et al. BAC constructs in transgenic reporter mouse lines control efficient and specific LacZ expression in hypertrophic chondrocytes under the complete Col10a1 promoter. Histochem Cell Biol 2007; 127:183 - 94; http://dx.doi.org/10.1007/s00418-006-0236-8; PMID: 17051351
- Topping RE, Bolander ME, Balian G. Type X collagen in fracture callus and the effects of experimental diabetes. Clin Orthop Relat Res 1994; 220 - 8; PMID: 7955687
- Storm EE, Huynh TV, Copeland NG, Jenkins NA, Kingsley DM, Lee SJ. Limb alterations in brachypodism mice due to mutations in a new member of the TGF beta-superfamily. Nature 1994; 368:639 - 43; http://dx.doi.org/10.1038/368639a0; PMID: 8145850
- Koyama E, Shibukawa Y, Nagayama M, Sugito H, Young B, Yuasa T, et al. A distinct cohort of progenitor cells participates in synovial joint and articular cartilage formation during mouse limb skeletogenesis. Dev Biol 2008; 316:62 - 73; http://dx.doi.org/10.1016/j.ydbio.2008.01.012; PMID: 18295755
- Bignone PA, Krupa RA, Sternberg H, Funk WD, Snyder EY, West MD, et al. Identification of human embryonic progenitor cell targeting peptides using phage display. PLoS One 2013; 8:e58200; http://dx.doi.org/10.1371/journal.pone.0058200; PMID: 23483995
- Scholzen T, Solursh M, Suzuki S, Reiter R, Morgan JL, Buchberg AM, et al. The murine decorin. Complete cDNA cloning, genomic organization, chromosomal assignment, and expression during organogenesis and tissue differentiation. J Biol Chem 1994; 269:28270 - 81; PMID: 7961765
- Micallef L, Vedrenne N, Billet F, Coulomb B, Darby IA, Desmoulière A. The myofibroblast, multiple origins for major roles in normal and pathological tissue repair. Fibrogenesis Tissue Repair 2012; 5:Suppl 1 S5; PMID: 23259712