Abstract
Dramatic morphogenetic processes underpin nearly every step of nervous system development, from initial neuronal migration and axon guidance to synaptogenesis. Underlying this morphogenesis are dynamic rearrangements of cytoskeletal architecture. Here we discuss the roles of the actin cytoskeleton in the development of presynaptic terminals, from the elaboration of terminal arbors to the recruitment of presynaptic vesicles and active zone components. The studies discussed here underscore the importance of actin regulation at every step in neuronal circuit assembly.
Introduction
Neural circuit assembly requires the coordination of multiple neurodevelopmental processes. Prior to the establishment of a functional circuit, neurons must migrate, polarize, and extend neurites. One of these neurites eventually forms the axon, which must interpret chemical cues to guide to its intended target. Once within the appropriate target field, axons must recognize and connect to postsynaptic partners. These distinct neurodevelopmental processes—cell migration, cell polarity, axon outgrowth, and synaptogenesis—all require organization of the actin cytoskeleton.Citation1,Citation2
Actin is an ancient and ubiquitous component of the cells that comprise all living organisms on Earth.Citation3 This protein performs multiple cellular functions, ranging from cellular movements to intracellular trafficking. Crucial to these cellular roles is the ability of actin to form filamentous polymers (F-actin). Polarized assembly of actin filaments (which grow more quickly at the barbed end) occurs spontaneously within our cells.Citation3 Filament assembly is promoted by actin nucleating, bundling and severing proteins and is terminated by actin capping proteins. These factors, together with a wider cast of actin regulatory proteins, determine the geometry and subcellular localization of F-actin structures.
Other reviews have highlighted the roles of the actin cytoskeleton in the coordination of neuronal growth cone movements toward or away from extracellular guidance cues,Citation4-Citation6 postsynaptic dendritic arbor assembly, and extension and plasticity of dendritic spines.Citation7,Citation8 Here we review recent findings that highlight functions for this versatile cellular component in the assembly of presynaptic terminals.
Roles for F-Actin in Specifying the Sites for Presynapse Differentiation
Actin polymerization produces the protrusive force necessary to drive advancement of neuronal growth cones.Citation4 Once the axon reaches its target, microfilament-comprised structures are also required for extension of the axonal arborizations and filopodia that can precede synapse formation. Axonal arborizations (referred to here as “terminal arbors”) are short branched structures containing presynapses (not to be confused with axonal branches resulting from growth cone bifurcationsCitation9). During neurodevelopment, terminal arbors precede synapse formation. Interestingly, however, axons may continue to arborize following synapse formation, and these secondary arbors often extend from existing presynaptic sites.Citation10-Citation12 One hypothesis that could explain this relationship is that terminal arborization and presynaptic assembly employ shared actin-regulatory components. For example, it has been observed that the Netrin receptor, UNC-40/DCC, and downstream actin regulatory molecules are required locally both for the clustering of synaptic vesicles and for the extension of terminal arbors.Citation12 These observations raise the possibility that actin regulatory components that are required locally for the assembly of presynaptic scaffolds may be adapted for subsequent actin organization, permitting local arbor extension from existing presynaptic sites.
Long-range axon guidance and short-range terminal arborization require distinct cellular pathways
Interestingly, the actin-regulatory molecules required for the initial, long-range guidance of axonal growth cones are not necessarily involved in short-range terminal arborization. For example, Ena/VASP proteins associate with the barbed ends of F-actin, precluding the binding of capping proteins. This activity promotes the formation of longer, unbranched filaments typical of filopodia.Citation13 Thus, one might expect that mis-targeting of such proteins would have catastrophic effects on growth cone guidance. To be sure, roles for these actin-regulatory proteins in modulating axon guidance are well documented.Citation14 However, in the case of axon guidance of retinal ganglion cells (RGCs), mis-targeting of Xena/VASP (Xenopus homologs of Ena/VASP) proteins to the mitochondrial surface does not affect initial axon outgrowth. Rather, axon guidance proceeds normally, but terminal arborizations do not form properly once these axons have reached their targets.Citation15 Similarly, the C. elegans Ena/VASP homolog, UNC-34, as well as the Lamellipodin homolog MIG-10, are largely dispensable for the guidance of the AVM, AIY and NSM axons.Citation12,Citation16-Citation19 Interestingly, however, UNC-34/Ena and MIG-10/Lamellipodin, while largely dispensable for NSM guidance, are required for NSM terminal arborization.Citation12
These findings indicate two important aspects about the role of actin organizing molecules such as UNC-34/Ena/VASP during neurodevelopment. First, they reveal a genetic requirement for actin organizing molecules during axon guidance and arborization. Importantly, these in vivo studies also reveal that axon guidance and arborization are genetically separable events. The significance of this second observation is that it suggests a mechanistic distinction between those processes underpinning the long distance migration of neuronal growth cones and the local assembly of presynaptic arbors. This mechanistic distinction could be related to the way in which actin is organized during these two neurodevelopmental processes. Understanding the ways in which actin regulatory mechanisms differ between growth cone guidance and terminal arborization is an outstanding goal within the field.
Actin nucleation: a double agent in terminal arborization
Actin nucleators permit the rapid assembly of actin filaments.Citation20 These new actin filaments can, in turn, promote the formation of terminal arbor structures. For example, Cordon-Bleu, an actin nucleator that produces unbranched actin filaments, promotes the formation of axon branches.Citation21 Arp2/3 also nucleates F-actin, but in a manner fundamentally different from that of Cordon-Bleu; Arp2/3 nucleates new actin branches on the sides of existing filaments. Although Cordon-Bleu and Arp2/3 nucleate actin through different mechanisms, both are associated with the formation of axon branches.Citation21-Citation23
However, actin nucleation does not necessarily promote the formation of terminal arbors. Indeed, the actin nucleating Arp2/3 pathway can also inhibit terminal arborization. Arp2/3 activity is promoted by members of the Wiskott-Aldrich syndrome protein (WASP) family. These Arp2/3 regulators are, in turn, localized to the membrane and disinhibited by F-BAR proteins such as Syndapins. Therefore Syndapins locally promote actin nucleation through WASP and the Arp2/3 complex. If actin nucleation were to exclusively promote terminal arbors, one would expect that elimination of Syndapin would result in fewer axon arbors. Instead, it was observed that knockdown of Syndapin in primary hippocampal neurons results in overgrowth of terminal arbors.Citation24 These results suggest that factors enhancing actin filament assembly can limit terminal arbor outgrowth.
Together, these studies show that actin nucleation can paradoxically promote or inhibit terminal arborization depending on the cellular context. One hypothesis to explain these results is that the position and structure of presynaptic F-actin determines its function. It has also been hypothesized that Arp2/3-dependent F-actin networks may retard the microtubule protrusion that promotes branch extension, thereby inhibiting arborization.Citation22,Citation24
Regardless of the mechanism, these findings indicate that F-actin nucleation per se is not predictive of axonal growth or arborization. Rather higher order organizers such as Syndapin and Ena/VASP proteins likely play a central role in selecting cellular outcomes arising from F-actin assembly. These findings are consistent with a model in which the geometry imposed by actin capping and bundling proteins plays a central role in determining whether actin nucleation results in axon branch extension or branch inhibition ().
Figure 1. Synaptic arborization and the actin cytoskeleton. Cartoon diagram of the involvement of local actin structures in the decision to not extend (left) or extend (right) an axon arbor. Proteins and structures are not drawn to scale. On the left, an Arp2/3-dependent F-actin network retards the advance of microtubules, preventing the stabilization of nascent filopodia. Presence of the capping protein, Eps8 prevents the extension of actin filaments into long, unbranched bundles. Syndapin localizes N-WASP to the membrane, where it can activate the Arp2/3 complex to form a dendritic array of F-actin. On the right, a nascent axon branch is forming. Such nascent branches are promoted by actin nucleating factors such as Cobl and Arp2/3. Supporting the organization of actin into long, unbranched filaments are proteins of the Ena/VASP family. Myosin Va may support these branch extensions through the local trafficking of materials. Note that a microtubule successfully invaded this nascent branch. Note also the relationship between synaptic vesicle clusters and branch extension. This spatial correlation between presynapses and axon arbor extensions has been observed in numerous systems and may reveal the presence of share actin regulatory components at presynaptic sites and at synaptic arbors.
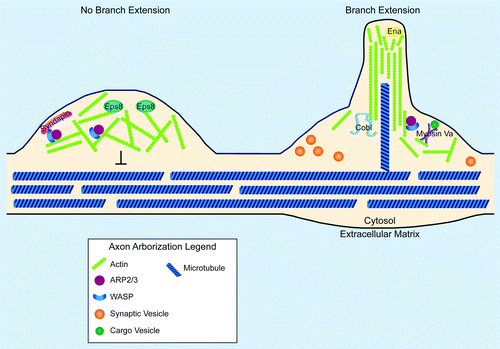
Capping and anti-capping proteins regulate presynaptic differentiation
Capping and anti-capping proteins represent a second group of proteins involved in the regulation of actin dynamics within axons.Citation25 Capping proteins terminate actin filament extension by binding barbed endsCitation26 (). On the other hand, proteins such as Ena/VASP promote actin filament extension in part by preventing the binding of capping proteins, such as Eps8, to actin.Citation13,Citation25 Cultured hippocampal neurons derived from Eps8 knockout mice form ectopic filopodia. The formation of these filopodia is dependent on the presence of VASP.Citation27 These results highlight the crucial role of capping proteins in instructing the morphological development of terminal axon structures. Perhaps even more exciting is the finding that the ectopic filopodia (that result when these capping proteins are missing) contain synaptobrevin clusters, suggesting the presence of ectopic presynaptic sites. These data from studies in neuronal culture could suggest the possibility that actin-capping proteins such as Eps8 indirectly limit presynaptic assembly by preventing the formation of ectopic filopodia. Examining the in vivo function of actin capping proteins such as Eps8 is critical, particularly in light of recent studies in neuronal culture, which demonstrate that Eps8 promotes, rather than inhibits the formation of spine synapses.Citation28 Although the function of Eps8 in vivo remains to be determined, these studies reveal a central role for capping proteins like Eps8 in organizing the actin cytoskeleton during synaptogenesis.
Actin plays non-structural roles in the assembly of terminal arbors
In addition to its structural role as an organizer of the presynaptic compartment, F-actin is also implicated in the transport mechanisms that support synaptic arborization. Whereas microtubules function as “highways” for the long-distance trafficking of proteins, organelles and membrane, actin provides a substrate for local transport in association with motor proteins such as myosin Va.Citation29 This role for F-actin has recently been linked to the growth of terminal arbors in the mouse cerebellum. Here, climbing fibers do not arborize properly to innervate Purkinje cells in myosin Va mutant mice.Citation30 While the specific cargo of myosin Va is not known in this developmental context, these data support a role for F-actin accumulations not only for the generation of protrusive force (as discussed in previous sections) but also for the assembly of a matrix for cellular transport.
Actin provides a scaffold for developing presynapses
Terminal arborization is followed by the assembly of presynaptic terminals. Both of these processes are dependent on the organization of F-actin. F-actin acts as a major scaffold for organization of the presynaptic compartment as evidenced by the fact that treatment of developing neurons with actin polymerization inhibitors results in the dispersal of synaptic vesicles and other presynaptic components. This structural dependence on F-actin is temporally restricted to the time during which synapses are forming, suggesting a specific and crucial involvement of F-actin in the building of a synapse.Citation31
In cell culture systems, synapse formation is remarkably promiscuous. Synapses form at points of contact between pairs of neurons, between neurons and their substrates, or even between a neuron and itself.Citation32 It has been hypothesized that in culture systems, local adhesion triggers local synapse assembly. Supporting this model is the finding that PDL-coated beads can induce synaptic vesicle clusters at sites of contact with axons of dissociated rat hippocampal neurons. Extracellularly, the heparan sulfate proteoglycan Syndecan-2 is hypothesized to mediate the neuronal interaction with the beads. Intracellularly, this interaction results in the local clustering of F-actin and synaptic vesicles. Synaptic vesicle clustering is diminished at bead contact sites in the presence of the actin-depolymerizing agent latrunculin, suggesting that F-actin clustering is required downstream from neuronal adhesion for the clustering of vesicles. This requirement for actin polymerization emphasizes the importance of cytoskeletal rearrangements as a link between local adhesion and local presynapse induction.Citation33
In contrast to the promiscuity of synapse formation in vitro, in vivo synapses form with remarkable specificity. This specificity is, in part, conferred by transmembrane molecules that mediate cell-cell contact and specify the sites for presynaptic assembly.Citation34 Like the presynaptic sites induced by PDL-coated beads, presynaptic sites specified by these transmembrane molecules are also dependent on F-actin reorganization. Recent studies have now identified molecular mechanisms that link these extracellular signaling and adhesion events with F-actin organization and synaptogenesis. In particular, the pathways directing cytoskeletal rearrangements downstream from immunoglobulin receptor- and cadherin-mediated synaptic adhesion, discussed below, are beginning to be elucidated.
IG adhesion receptors influence synapse formation through cytoskeletal interactions
Immunoglobulin (IG) superfamily adhesion receptors are crucial mediators of in vivo synapse formation.Citation16,Citation34-Citation42 For example, the interaction between the L1 cell adhesion molecule (L1CAM) and the Spectrin/actin adaptor protein Ankyrin has been shown to instruct GABAergic presynapse formation in the mouse cortex.Citation37 These data provide evidence that transmembrane IG proteins may influence synaptogenesis through local cytoskeletal interactions in vivo.
Similarly, the IG superfamily protein Basigin is required in the Drosophila neuromuscular junction (NMJ) for presynaptic formation. Mutants lacking functional Basigin display aberrant NMJ bouton number, size, terminal branching, and synaptic vesicle distribution. Interestingly, these mutants also display inappropriate actin and spectrin cytoskeletal organization; F-actin and spectrin markers form aggregates within synaptic boutons rather than localizing exclusively to the synaptic plasma membrane.Citation38 These data suggest that like L1CAM, Basigin may influence presynaptic organization in part through the local organization of spectrin and F-actin.
In the nematode C. elegans, IG molecules also act through F-actin to influence presynaptic differentiation. For instance, Netrin signals through the IG receptor UNC-40/DCC to instruct local clustering of F-actin and synaptic vesicles.Citation17 At a different C. elegans synapse, the IG transmembrane adhesion molecule SYG-1 is necessary and sufficient for presynaptic F-actin assembly. Here, as in the case of artificial presynapse assembly on PDL beads, the disruption of F-actin results in defective synapse assembly, and ectopic localization of SYG-1 induces ectopic F-actin accumulations.Citation43 These findings in both vertebrate and invertebrate systems emphasize the importance of F-actin as a link between IG signaling and adhesion molecules and presynaptic differentiation.
Cadherins assist in presynapse scaffold assembly
Cadherins are adhesion molecules that form trans-synaptic homophilic dimers, engaging downstream F-actin rearrangements through catenins. These adhesion molecules are critical both pre- and post-synaptically for appropriate synaptic differentiation.Citation44 Presynaptically, elimination of cadherin function results in impairments of synapsin and synaptic vesicle localization and recycling.Citation45 In vitro studies suggest that cadherin-mediated adhesion directly triggers presynaptic actin polymerization and subsequent synaptic vesicle trapping through the Rac GEF β-Pix.Citation46 Here again, transmembrane molecules specify the sites of F-actin accumulation, and in effect the sites of downstream presynapse differentiation.
Cadherins also play an intriguing role in synaptic maturation. In young synapses, depolymerization of F-actin results in the dispersion not only of synaptic vesicles, but also of synaptic N-cadherin clusters.Citation31 Thus, in the early stages of presynapse assembly, adhesion molecules both recruit and are stabilized by F-actin. Mature synapses, on the other hand, are resistant to actin depolymerizing agents. While immature synapses are disassembled by latrunculin, more mature synapses are stable upon challenge with this depolymerizing agent.Citation31 Interestingly, if N-cadherin is blocked, the synapses that still form remain sensitive to F-actin depolymerization during their second week in culture.Citation47 Thus, N-cadherin may contribute to the assembly of secondary scaffolds that assist in the maturation of synapses. Collectively, these data show that N-cadherin-mediated adhesion can directly influence cytoskeletal rearrangements associated with presynapse assembly. In the early synapse, these structures serve both as a temporary scaffold for maintaining synaptic structural integrity and as a matrix to trap and tether additional synaptic components and stabilize adhesion molecules.
In conclusion, extracellular cues, whether specific as in the case of synaptic adhesion molecules, or non-specific as in the case of PDL-coated beads, can designate the positions of nascent synapses. Multiple groups have shown that these synaptogenic cues often converge on pathways that regulate the local assembly of F-actin. Local extracellular cues thus become translated into localized intracellular accumulations of actin. In the early stages of synaptogenesis, F-actin and cell adhesion matrices are critical and interdependent. As the synapse matures, these networks permit the recruitment of materials integral to synaptic functioning, including synaptic vesicles and components of the active zone.
F-Actin is Required for Proper Development of the Presynaptic Active Zone and Synaptic Vesicle Pools
Presynaptic sites contain synaptic vesicles and active zones (). Actin is essential for the formation and function of these two presynaptic structures. This requirement for F-actin is best demonstrated by the finding that removal of F-actin early in synapse assembly results in disruption of synaptic structure.Citation31 The roles of F-actin in the functioning of mature synapses, including endocytosis, exocytosis, and plasticity, have been reviewed previously and will not be addressed here.Citation48-Citation51 Instead, we will focus on the role of F-actin in the assembly of the presynaptic active zone and synaptic vesicle clustering.
Figure 2. F-actin in synaptogenesis. Cartoon diagram of the molecules and pathways that interact with F-actin in synaptic vesicle clustering (left panel) and active zone assembly (right panel) in an en passant presynapse. The postsynaptic cell is not depicted. Proteins and structures are not drawn to scale. In both panels, the green gradient away from the active zone represents presynaptic actin. Also, green circles (actin) are present to demonstrate direct actin binding by specific molecules (profilin, NAB-1/Neurabin, Arp2/3-WASP complex, and WAVE complex). In the left panel, only one pathway downstream of cadherin is represented, given space constraints. Arrows denote positive regulation; dashed lines indicate indirect regulation (left panel) or uncertain regulation (right panel). Arrows to the synaptic vesicle cluster in the left panel illustrate instruction of synaptic vesicle clustering through F-actin. Arrows to the developing active zone (gray density at the membrane) in the right panel indicate instruction of active zone assembly through F-actin (for profilin and Arp2/3) or addition of active zone components to the growing active zone (for Liprin-α, SYD-1, and ELKS).
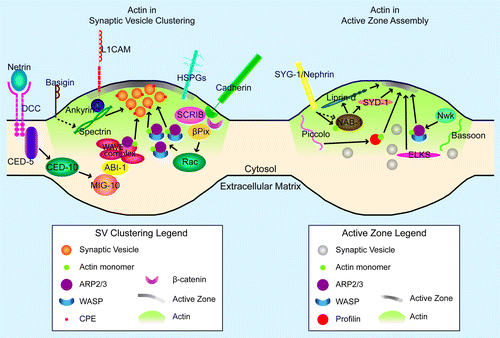
F-actin organization at the presynapse
The presynaptic compartment contains hundreds of proteins, including those associated with synaptic vesicles, channels, SNAREs, active zone cytomatrix components, endocytic machinery, and adhesion molecules.Citation52-Citation55 While studies have demonstrated that F-actin is required for the proper development and organization of the presynaptic components of synapses, as we will discuss in the ensuing sections,Citation31,Citation33,Citation43 there is disagreement over the localization of F-actin in the presynaptic compartment.
Some studies have found that presynaptic F-actin associates with and surrounds the active zone and synaptic vesicle pool.Citation56-Citation61 In C. elegans, F-actin also partially colocalizes with synaptic vesicle markers. In addition, several mutant backgrounds simultaneously disrupt F-actin and synaptic vesicle clusters. These findings suggest that F-actin functions within or near synaptic vesicle pools.Citation17,Citation43,Citation62 At the Drosophila NMJ, actin is observed as distinct puncta in synaptic boutons.Citation63 Similarly, several proteomic studies have identified actin as a component of the synaptic vesicle and active zone proteomes.Citation52,Citation54,Citation55,Citation64-Citation67 Interestingly, in lamprey reticulospinal neurons, two functionally and structurally separate pools of presynaptic actin have been identified: one associated with the active zone and the other with synaptic vesicle pools. These two pools are differentially susceptible to pharmacological agents that disrupt F-actin structures.Citation68 Together, these studies provide ultrastructural, cell biological, and biochemical evidence that indicates that actin is present near synaptic vesicle pools and at the active zone.
Curiously, cryoelectron tomography of mammalian central nervous system synapses demonstrated that presynaptic compartments only occasionally contained actin filaments, and actin bundles were observed 300 nm away from the active zone.Citation69 In addition, actin filaments were rarely observed in cerebellar slices that were either fixed with aldehyde or rapidly frozen.Citation70 The discrepancies in F-actin localization between the EM tomography studies and the cell biological studies discussed in the previous paragraph may be due to differences between specific synapses, including the stage of development of the observed synapse. It has been demonstrated that the presence of F-actin at the synapse is most critical during early development and that disruption of actin in mature synapses does not disrupt clustering of synaptic proteins.Citation31 Therefore, it is possible that F-actin localization at the synapse changes during development. In addition, actin cytoskeleton structures are fragile and susceptible to deformation during certain preparations for electron microscopy.Citation71 Taking advantage of the latest technologies, such as super-resolution light microscopy and systematically analyzing nascent and developing synapses across different species will provide a more comprehensive understanding of F-actin localization in the presynaptic compartment.
F-actin regulation of synaptic vesicle clustering
Over the past few decades, several groups have worked to understand how synaptic vesicles remain clustered in several different pools near the presynaptic active zone. A significant effort has gone into clarifying the role of synapsins as links between F-actin and synaptic vesicles to provide a molecular mechanism for synaptic vesicle clustering. These findings have been previously reviewed.Citation72-Citation76 Here we will discuss the mechanisms that act upstream of synapsins during initial synaptic vesicle clustering and the synapsin-independent pathways that maintain these organized synaptic vesicle pools after preliminary presynaptic assembly (, left panel).
During presynaptic development, actin is required for the growth and maintenance of synaptic vesicle clusters.Citation31 Formins are actin-interacting proteins that nucleate and elongate unbranched filaments through interactions at the barbed end of actin.Citation77-Citation80 In Drosophila NMJs, a member of a formin subfamily, Diaphanous (Dia), is required at the presynapse for synaptic growth and synaptic vesicle localization.Citation63 Diaphanous acts downstream of DLar, a receptor tyrosine phosphatase, in NMJ synaptic growth. The decrease in synaptic vesicle pools in dlar;dia double mutants was not significantly different from either single mutant, suggesting that DLar and Dia function in the same pathway to cluster vesicles. In addition, both mutants disrupt actin organization at synaptic boutons.Citation63 Together, these results suggest that DLar acts upstream of Diaphanous to regulate the actin cytoskeleton and synaptic vesicle pool organization at synaptic boutons.
Rac and Cdc42 are also well-known regulators of the actin cytoskeleton.Citation81 In developing neurons, Rac GTPases can regulate axon outgrowth, guidance and branching by influencing actin cytoskeleton organization.Citation82 Rac GTPase pathways are also important regulators of synaptic vesicle organization at the presynapse. For example, knockdown of β-Pix, a Rac/Cdc42 GEF, causes a decrease in actin puncta at synapses and mislocalization of synaptic vesicles. Interestingly, overexpression of cortactin, a protein that polymerizes actin, rescued β-Pix knockdown and increased synaptic vesicle colocalization with actin in presynaptic regions.Citation46 These results demonstrate that Rac GTPase signaling is required for actin polymerization at presynapses, which is, in turn, required for proper synaptic vesicle localization and clustering. In addition, a cell adhesion molecule cadherin/ β-catenin/scribble complex interacts with and recruits β-Pix to cell–cell contact sites, providing a link between extracellular signals, actin and synaptic vesicle clustering.Citation46 Together, these studies demonstrate that clusters of F-actin at presynaptic sites recruit and stabilize synaptic vesicles during presynaptic development.
Similarly, in C. elegans, Netrin can instruct synaptic vesicle clustering at nascent synapses via mechanisms that depend on Rac and the WAVE regulatory complex.Citation17,Citation62 Interestingly, the RacGEF involved in this pathway, CED-5/DOCK180, while required for vesicle clustering, is not required for proper localization of active zone proteins to presynaptic sites. These findings suggest that active zone assembly and synaptic vesicle clustering are instructed by two different signaling pathways downstream from Netrin.Citation17 Thus, while actin plays a role in synaptic vesicle clustering and (as we will discuss in the next section) in active zone assembly, these processes can depend on distinct actin regulatory proteins.
After presynaptic development, a primary role of presynaptic F-actin is to contain synaptic vesicles at the presynaptic active zone and prevent synaptic vesicle fusion.Citation56,Citation83 Carboxypeptidase E (CPE), a transmembrane protein present on synaptic vesicles, is required for maintaining vesicle clustering. Studies in vertebrates and invertebrates have demonstrated that CPE is required for neurotransmission.Citation84-Citation86 Cell biological studies in CPE knockout mice demonstrated that loss of CPE leads to significant reductions of synaptic vesicles docked at active zones. This phenotype, which is similar to that of synapsin knockouts, was not the result of decreased synaptic protein levels or of defects in synaptic vesicle biogenesis.Citation75,Citation87 Instead, CPE mediates an interaction with the actin cytoskeleton to regulate synaptic vesicle clustering. CPE interacts with γ-adducin.Citation87 In turn, γ-adducins cap actin near the plasma membrane and regulate presynaptic shape and growth.Citation87,Citation88 CPE can also interact with the active zone protein RIM (Rab3-interacting molecule), which directs synaptic vesicle docking at the membrane.Citation87,Citation89 Therefore, carboxypeptidase E provides a link between F-actin and synaptic vesicles. This connection, which is important for vesicle clustering, is distinct from the link provided by synapsins. Together, these findings indicate that F-actin directs synaptic vesicle clustering through vesicle-associated proteins such as CPE and synapsin. F-actin regulates synaptic vesicle clustering both through pathways independent of synapsins and pathways that signal through synapsins, suggesting that multiple signaling mechanisms interpret the F-actin presynaptic structure to mediate presynaptic assembly. Collectively, these studies indicate that F-actin is required during synapse formation for the growth and maintenance of synaptic vesicle clusters.
F-actin at the active zone
Active zones provide the protein framework that supports docking and priming of synaptic vesicles, localization of voltage-gated Ca2+ channels, exact apposition of pre- and postsynaptic specializations, and facilitation of short- and long-term synaptic plasticity.Citation89 Despite the importance of the presynaptic active zone in synaptic function, the molecular mechanisms of active zone assembly during synaptogenesis are poorly understood. Several protein components of the active zone have been identified, such as Bruchpilot/ELKS-1, RIMs (Rab3-interacting molecules), SYD-2/Liprin-α, SYD-1, Piccolo, and Bassoon.Citation89,Citation90 While the presence of actin at the active zone is often reported, the role of actin and other protein constituents in active zone development are not fully understood.
Recent studies in invertebrates have provided new molecular links between actin and active zone assembly (). Specifically, studies have identified distinct signaling pathways that control F-actin organization to influence active zone development. For example, the C. elegans homolog of neurabin, NAB-1, binds to and colocalizes with F-actin in presynaptic terminals. This interaction is necessary for NAB-1 participation in active zone assembly. Although F-actin remains synaptically localized in nab-1 mutants, NAB-1 becomes mislocalized when F-actin assembly is disrupted with latrunculin. These data suggest that F-actin directs the synaptic localization of NAB-1. NAB-1 also forms a complex with active zone proteins SYD-1 and SYD-2. Therefore, NAB-1 acts as a molecular bridge between F-actin and the active zone during synapse assembly.Citation43
The evolutionarily conserved adaptor protein, Nervous Wreck (Nwk), directs active zone formation in Drosophila by regulating actin. nwk mutants display fewer active zones and exhibit decreased synaptic transmission compared with wild-type flies. Nwk directly interacts with WASP, an Arp2/3 complex actin regulator, and both proteins, Nwk and WASP, colocalize at the periactive zone. wsp mutants phenocopy nwk mutants, suggesting that Nwk and Wsp are members of a pathway that modulates synaptogenesis through regulation of actin dynamics.Citation58 These results suggest that Nwk regulates active zone assembly by regulating actin dynamics, again placing actin dynamics upstream of active zone assembly. Consistent with actin playing a role upstream of active zone assembly, studies in vertebrates demonstrate that F-actin depolymerization in young synapses results in a loss of clusters of the active zone protein Bassoon. Interestingly, the dependence of Bassoon localization on F-actin content disappears with synaptic age, emphasizing the importance of actin organization during synaptogenesis.Citation31 Together, these studies suggest that diverse signaling pathways converge to influence F-actin organization at the presynapse, and that active zone formation is downstream from presynaptic F-actin organization.
Interestingly, recent studies demonstrate that active zone proteins can also modulate F-actin organization in the presynapse. Piccolo is a large, conserved protein associated with the active zone.Citation89 Originally thought to be unique to vertebrates, a Piccolo homolog, Fife, was identified in invertebrates.Citation91 Knockdown of Piccolo with shRNA causes dispersion of Synapsin1a and F-actin at the presynaptic terminal and causes a loss of tight association between Synapsin1a, actin, and synaptic vesicles.Citation56,Citation92 Piccolo also interacts with actin regulators such as profilin.Citation56 Thus, the active zone protein Piccolo can regulate presynaptic F-actin organization. Collectively, these studies suggest two important conceptual points. First, F-actin is necessary for proper active zone formation. These studies also indicate that active zone proteins, such as Piccolo, can play important roles in organizing F-actin, thereby providing a feedback loop during active zone assembly.
Together, these studies demonstrate that presynaptic F-actin directs the formation of two critical presynaptic elements: the active zone and synaptic vesicle clusters. Interestingly, disruption of actin-organizing molecules sometimes affects only one of these two presynaptic elements. In C. elegans, mutations that alter active zone localization do not necessarily affect synaptic vesicle localization.Citation93 In Drosophila, ghost boutons contain synaptic vesicles, but lack active zones.Citation94 As we previously discussed, C. elegans CED-5/DOCK180 regulates both synaptic vesicle clustering and presynaptic F-actin localization, but is dispensable for active zone assembly.Citation17 These observations support the hypothesis that while presynaptic F-actin organization is a shared requirement for both synaptic vesicle clustering and active zone assembly, different actin-organizing modules could dictate the development of these two presynaptic elements. These results are also consistent with observations in the lamprey reticulospinal neurons that demonstrated the existence of separate pools of presynaptic actin in the active zone and in synaptic vesicle pools.Citation68 It would be interesting to determine whether different presynaptic F-actin structures could separately direct either active zone formation or synaptic vesicle clustering.
Concluding Remarks
We have discussed the roles of F-actin and its regulators in the assembly of presynaptic terminal structures. Although we still have a great deal to learn, it is clear that the actin cytoskeleton, which is important for growth cone dynamics during axon guidance, can be reorganized for the regulation of terminal arborization and presynaptic assembly. There is exquisite regulation of F-actin dynamics in the presynaptic compartment. While various pathways converge on F-actin regulators such as Wasp and Arp2/3 to organize the F-actin cytoskeleton, the neurodevelopmental outcomes resulting from actin polymerization can be distinct, depending on where and how actin is organized.
Much progress has been made toward understanding the role of the actin cytoskeleton in presynaptic assembly, but many outstanding questions remain. What is the geometry of actin structures within arborizing axons? How do these structures compare with those within growth cones? It is possible that the underlying differences between actin organization in these two structures may explain the selection of a particular morphogenic program. What is the relationship between actin and other cytoskeletal elements during the specification of presynaptic terminals? Similarly, in each cellular context, it will be critical to differentiate between the structural roles of actin and its transport and synaptic vesicle clustering roles. It will also be important to elucidate the subcellular localization of actin at all stages of presynaptic development and after presynaptic maturation. Determining where actin is in the presynaptic compartment will facilitate a better understanding of the roles of actin in the presynapse. Can actin direct multiple aspects of presynaptic assembly within the same cell? For example, it will be interesting to ascertain how F-actin can separately regulate synaptic vesicle clustering and active zone assembly. Furthermore, unraveling the feedback loops and nonlinear relationships between actin and active zone proteins may clarify how the organization of the cytoarchitecture influences the development of the neural architecture.
Acknowledgments
We apologize to colleagues whose work we did not cite due to lack of space or unintentional oversight. We thank A. Koleske, T. Biederer and K. Shen for thoughtful comments on the manuscript. This work was supported by a March of Dimes Research Grant and an R01 from the National Institutes of Health (NS076558).
Disclosure of Potential Conflicts of Interest
No potential conflicts of interest were disclosed.
References
- Benson DL, Colman DR, Huntley GW. Molecules, maps and synapse specificity. Nat Rev Neurosci 2001; 2:899 - 909; http://dx.doi.org/10.1038/35104078; PMID: 11733797
- Holt CE, Harris WA. Target selection: invasion, mapping and cell choice. Curr Opin Neurobiol 1998; 8:98 - 105; http://dx.doi.org/10.1016/S0959-4388(98)80013-5; PMID: 9568397
- Pollard TD, Cooper JA. Actin, a central player in cell shape and movement. Science 2009; 326:1208 - 12; http://dx.doi.org/10.1126/science.1175862; PMID: 19965462
- Dent EW, Gupton SL, Gertler FB. The growth cone cytoskeleton in axon outgrowth and guidance. Cold Spring Harb Perspect Biol 2011; 3:a001800; http://dx.doi.org/10.1101/cshperspect.a001800; PMID: 21106647
- Kessels MM, Schwintzer L, Schlobinski D, Qualmann B. Controlling actin cytoskeletal organization and dynamics during neuronal morphogenesis. Eur J Cell Biol 2011; 90:926 - 33; http://dx.doi.org/10.1016/j.ejcb.2010.08.011; PMID: 20965607
- Geraldo S, Gordon-Weeks PR. Cytoskeletal dynamics in growth-cone steering. J Cell Sci 2009; 122:3595 - 604; http://dx.doi.org/10.1242/jcs.042309; PMID: 19812305
- Svitkina T, Lin WH, Webb DJ, Yasuda R, Wayman GA, Van Aelst L, et al. Regulation of the postsynaptic cytoskeleton: roles in development, plasticity, and disorders. J Neurosci 2010; 30:14937 - 42; http://dx.doi.org/10.1523/JNEUROSCI.4276-10.2010; PMID: 21068295
- Frost NA, Kerr JM, Lu HE, Blanpied TA. A network of networks: cytoskeletal control of compartmentalized function within dendritic spines. Curr Opin Neurobiol 2010; 20:578 - 87; http://dx.doi.org/10.1016/j.conb.2010.06.009; PMID: 20667710
- Gallo G. The cytoskeletal and signaling mechanisms of axon collateral branching. Dev Neurobiol 2011; 71:201 - 20; http://dx.doi.org/10.1002/dneu.20852; PMID: 21308993
- Meyer MP, Smith SJ. Evidence from in vivo imaging that synaptogenesis guides the growth and branching of axonal arbors by two distinct mechanisms. J Neurosci 2006; 26:3604 - 14; http://dx.doi.org/10.1523/JNEUROSCI.0223-06.2006; PMID: 16571769
- Manitt C, Nikolakopoulou AM, Almario DR, Nguyen SA, Cohen-Cory S. Netrin participates in the development of retinotectal synaptic connectivity by modulating axon arborization and synapse formation in the developing brain. J Neurosci 2009; 29:11065 - 77; http://dx.doi.org/10.1523/JNEUROSCI.0947-09.2009; PMID: 19741113
- Nelson JC, Colón-Ramos DA. Serotonergic neurosecretory synapse targeting is controlled by netrin-releasing guidepost neurons in Caenorhabditis elegans. J Neurosci 2013; 33:1366 - 76; http://dx.doi.org/10.1523/JNEUROSCI.3471-12.2012; PMID: 23345213
- Bear JE, Gertler FB. Ena/VASP: towards resolving a pointed controversy at the barbed end. J Cell Sci 2009; 122:1947 - 53; http://dx.doi.org/10.1242/jcs.038125; PMID: 19494122
- Drees F, Gertler FB. Ena/VASP: proteins at the tip of the nervous system. Curr Opin Neurobiol 2008; 18:53 - 9; http://dx.doi.org/10.1016/j.conb.2008.05.007; PMID: 18508258
- Dwivedy A, Gertler FB, Miller J, Holt CE, Lebrand C. Ena/VASP function in retinal axons is required for terminal arborization but not pathway navigation. Development 2007; 134:2137 - 46; http://dx.doi.org/10.1242/dev.002345; PMID: 17507414
- Colón-Ramos DA, Margeta MA, Shen K. Glia promote local synaptogenesis through UNC-6 (netrin) signaling in C. elegans. Science 2007; 318:103 - 6; http://dx.doi.org/10.1126/science.1143762; PMID: 17916735
- Stavoe AKH, Colón-Ramos DA. Netrin instructs synaptic vesicle clustering through Rac GTPase, MIG-10, and the actin cytoskeleton. J Cell Biol 2012; 197:75 - 88; http://dx.doi.org/10.1083/jcb.201110127; PMID: 22451697
- Chang C, Adler CE, Krause M, Clark SG, Gertler FB, Tessier-Lavigne M, et al. MIG-10/lamellipodin and AGE-1/PI3K promote axon guidance and outgrowth in response to slit and netrin. Curr Biol 2006; 16:854 - 62; http://dx.doi.org/10.1016/j.cub.2006.03.083; PMID: 16618541
- Axäng C, Rauthan M, Hall DH, Pilon M. Developmental genetics of the C. elegans pharyngeal neurons NSML and NSMR. BMC Dev Biol 2008; 8:38; http://dx.doi.org/10.1186/1471-213X-8-38; PMID: 18400083
- Chesarone MA, Goode BL. Actin nucleation and elongation factors: mechanisms and interplay. Curr Opin Cell Biol 2009; 21:28 - 37; http://dx.doi.org/10.1016/j.ceb.2008.12.001; PMID: 19168341
- Ahuja R, Pinyol R, Reichenbach N, Custer L, Klingensmith J, Kessels MM, et al. Cordon-bleu is an actin nucleation factor and controls neuronal morphology. Cell 2007; 131:337 - 50; http://dx.doi.org/10.1016/j.cell.2007.08.030; PMID: 17956734
- Strasser GA, Rahim NA, VanderWaal KE, Gertler FB, Lanier LM. Arp2/3 is a negative regulator of growth cone translocation. Neuron 2004; 43:81 - 94; http://dx.doi.org/10.1016/j.neuron.2004.05.015; PMID: 15233919
- Spillane M, Ketschek A, Donnelly CJ, Pacheco A, Twiss JL, Gallo G. Nerve growth factor-induced formation of axonal filopodia and collateral branches involves the intra-axonal synthesis of regulators of the actin-nucleating Arp2/3 complex. J Neurosci 2012; 32:17671 - 89; http://dx.doi.org/10.1523/JNEUROSCI.1079-12.2012; PMID: 23223289
- Dharmalingam E, Haeckel A, Pinyol R, Schwintzer L, Koch D, Kessels MM, et al. F-BAR proteins of the syndapin family shape the plasma membrane and are crucial for neuromorphogenesis. J Neurosci 2009; 29:13315 - 27; http://dx.doi.org/10.1523/JNEUROSCI.3973-09.2009; PMID: 19846719
- Menna E, Fossati G, Scita G, Matteoli M. From filopodia to synapses: the role of actin-capping and anti-capping proteins. Eur J Neurosci 2011; 34:1655 - 62; http://dx.doi.org/10.1111/j.1460-9568.2011.07897.x; PMID: 22103422
- Cooper JA, Schafer DA. Control of actin assembly and disassembly at filament ends. Curr Opin Cell Biol 2000; 12:97 - 103; http://dx.doi.org/10.1016/S0955-0674(99)00062-9; PMID: 10679358
- Menna E, Disanza A, Cagnoli C, Schenk U, Gelsomino G, Frittoli E, et al. Eps8 regulates axonal filopodia in hippocampal neurons in response to brain-derived neurotrophic factor (BDNF). PLoS Biol 2009; 7:e1000138; http://dx.doi.org/10.1371/journal.pbio.1000138; PMID: 19564905
- Stamatakou E, Marzo A, Gibb A, Salinas PC. Activity-dependent spine morphogenesis: a role for the actin-capping protein Eps8. J Neurosci 2013; 33:2661 - 70; http://dx.doi.org/10.1523/JNEUROSCI.0998-12.2013; PMID: 23392693
- Desnos C, Huet S, Darchen F.. 'Should I stay or should I go?': myosin V function in organelle trafficking. Biology of the cell / under the auspices of the European Cell Biology Organization 2007; 99:411 - 23; http://dx.doi.org/10.1042/BC20070021
- Takagishi Y, Hashimoto K, Kayahara T, Watanabe M, Otsuka H, Mizoguchi A, et al. Diminished climbing fiber innervation of Purkinje cells in the cerebellum of myosin Va mutant mice and rats. Dev Neurobiol 2007; 67:909 - 23; http://dx.doi.org/10.1002/dneu.20375; PMID: 17506494
- Zhang W, Benson DL. Stages of synapse development defined by dependence on F-actin. J Neurosci 2001; 21:5169 - 81; PMID: 11438592
- Vaughn JE. Fine structure of synaptogenesis in the vertebrate central nervous system. Synapse 1989; 3:255 - 85; http://dx.doi.org/10.1002/syn.890030312; PMID: 2655146
- Lucido AL, Suarez Sanchez F, Thostrup P, Kwiatkowski AV, Leal-Ortiz S, Gopalakrishnan G, et al. Rapid assembly of functional presynaptic boutons triggered by adhesive contacts. J Neurosci 2009; 29:12449 - 66; http://dx.doi.org/10.1523/JNEUROSCI.1381-09.2009; PMID: 19812321
- Maeder CI, Shen K. Genetic dissection of synaptic specificity. Curr Opin Neurobiol 2011; 21:93 - 9; http://dx.doi.org/10.1016/j.conb.2010.10.004; PMID: 21087855
- Ango F, di Cristo G, Higashiyama H, Bennett V, Wu P, Huang ZJ. Ankyrin-based subcellular gradient of neurofascin, an immunoglobulin family protein, directs GABAergic innervation at purkinje axon initial segment. Cell 2004; 119:257 - 72; http://dx.doi.org/10.1016/j.cell.2004.10.004; PMID: 15479642
- Ango F, Wu C, Van der Want JJ, Wu P, Schachner M, Huang ZJ. Bergmann glia and the recognition molecule CHL1 organize GABAergic axons and direct innervation of Purkinje cell dendrites. PLoS Biol 2008; 6:e103; http://dx.doi.org/10.1371/journal.pbio.0060103; PMID: 18447583
- Guan H, Maness PF. Perisomatic GABAergic innervation in prefrontal cortex is regulated by ankyrin interaction with the L1 cell adhesion molecule. Cereb Cortex 2010; 20:2684 - 93; http://dx.doi.org/10.1093/cercor/bhq016; PMID: 20156840
- Besse F, Mertel S, Kittel RJ, Wichmann C, Rasse TM, Sigrist SJ, et al. The Ig cell adhesion molecule Basigin controls compartmentalization and vesicle release at Drosophila melanogaster synapses. J Cell Biol 2007; 177:843 - 55; http://dx.doi.org/10.1083/jcb.200701111; PMID: 17548512
- Shen K, Fetter RD, Bargmann CI. Synaptic specificity is generated by the synaptic guidepost protein SYG-2 and its receptor, SYG-1. Cell 2004; 116:869 - 81; http://dx.doi.org/10.1016/S0092-8674(04)00251-X; PMID: 15035988
- Yamagata M, Sanes JR. Dscam and Sidekick proteins direct lamina-specific synaptic connections in vertebrate retina. Nature 2008; 451:465 - 9; http://dx.doi.org/10.1038/nature06469; PMID: 18216854
- Yamagata M, Weiner JA, Sanes JR. Sidekicks: synaptic adhesion molecules that promote lamina-specific connectivity in the retina. Cell 2002; 110:649 - 60; http://dx.doi.org/10.1016/S0092-8674(02)00910-8; PMID: 12230981
- Biederer T, Sara Y, Mozhayeva M, Atasoy D, Liu X, Kavalali ET, et al. SynCAM, a synaptic adhesion molecule that drives synapse assembly. Science 2002; 297:1525 - 31; http://dx.doi.org/10.1126/science.1072356; PMID: 12202822
- Chia PH, Patel MR, Shen K. NAB-1 instructs synapse assembly by linking adhesion molecules and F-actin to active zone proteins. Nat Neurosci 2012; 15:234 - 42; http://dx.doi.org/10.1038/nn.2991; PMID: 22231427
- Benson DL, Huntley GW. Synapse adhesion: a dynamic equilibrium conferring stability and flexibility. Curr Opin Neurobiol 2012; 22:397 - 404; http://dx.doi.org/10.1016/j.conb.2011.09.011; PMID: 22019151
- Togashi H, Abe K, Mizoguchi A, Takaoka K, Chisaka O, Takeichi M. Cadherin regulates dendritic spine morphogenesis. Neuron 2002; 35:77 - 89; http://dx.doi.org/10.1016/S0896-6273(02)00748-1; PMID: 12123610
- Sun Y, Bamji SX. β-Pix modulates actin-mediated recruitment of synaptic vesicles to synapses. J Neurosci 2011; 31:17123 - 33; http://dx.doi.org/10.1523/JNEUROSCI.2359-11.2011; PMID: 22114281
- Bozdagi O, Valcin M, Poskanzer K, Tanaka H, Benson DL. Temporally distinct demands for classic cadherins in synapse formation and maturation. Mol Cell Neurosci 2004; 27:509 - 21; http://dx.doi.org/10.1016/j.mcn.2004.08.008; PMID: 15555928
- Doussau F, Augustine GJ. The actin cytoskeleton and neurotransmitter release: an overview. Biochimie 2000; 82:353 - 63; http://dx.doi.org/10.1016/S0300-9084(00)00217-0; PMID: 10865123
- Dillon C, Goda Y. The actin cytoskeleton: integrating form and function at the synapse. Annu Rev Neurosci 2005; 28:25 - 55; http://dx.doi.org/10.1146/annurev.neuro.28.061604.135757; PMID: 16029114
- Saheki Y, De Camilli P. Synaptic vesicle endocytosis. Cold Spring Harb Perspect Biol 2012; 4:a005645; http://dx.doi.org/10.1101/cshperspect.a005645; PMID: 22763746
- Cingolani LA, Goda Y. Actin in action: the interplay between the actin cytoskeleton and synaptic efficacy. Nat Rev Neurosci 2008; 9:344 - 56; http://dx.doi.org/10.1038/nrn2373; PMID: 18425089
- Morciano M, Beckhaus T, Karas M, Zimmermann H, Volknandt W. The proteome of the presynaptic active zone: from docked synaptic vesicles to adhesion molecules and maxi-channels. J Neurochem 2009; 108:662 - 75; http://dx.doi.org/10.1111/j.1471-4159.2008.05824.x; PMID: 19187093
- Yan D, Noma K, Jin Y. Expanding views of presynaptic terminals: new findings from Caenorhabditis elegans. Curr Opin Neurobiol 2012; 22:431 - 7; http://dx.doi.org/10.1016/j.conb.2011.10.002; PMID: 22036768
- Stevens SM Jr., Zharikova AD, Prokai L. Proteomic analysis of the synaptic plasma membrane fraction isolated from rat forebrain. Brain Res Mol Brain Res 2003; 117:116 - 28; http://dx.doi.org/10.1016/S0169-328X(03)00282-1; PMID: 14559145
- Volknandt W, Karas M. Proteomic analysis of the presynaptic active zone. Exp Brain Res 2012; 217:449 - 61; http://dx.doi.org/10.1007/s00221-012-3031-x; PMID: 22354101
- Waites CL, Leal-Ortiz SA, Andlauer TF, Sigrist SJ, Garner CC. Piccolo regulates the dynamic assembly of presynaptic F-actin. J Neurosci 2011; 31:14250 - 63; http://dx.doi.org/10.1523/JNEUROSCI.1835-11.2011; PMID: 21976510
- Sankaranarayanan S, Atluri PP, Ryan TA. Actin has a molecular scaffolding, not propulsive, role in presynaptic function. Nat Neurosci 2003; 6:127 - 35; http://dx.doi.org/10.1038/nn1002; PMID: 12536209
- Coyle IP, Koh YH, Lee WC, Slind J, Fergestad T, Littleton JT, et al. Nervous wreck, an SH3 adaptor protein that interacts with Wsp, regulates synaptic growth in Drosophila. Neuron 2004; 41:521 - 34; http://dx.doi.org/10.1016/S0896-6273(04)00016-9; PMID: 14980202
- Bloom O, Evergren E, Tomilin N, Kjaerulff O, Löw P, Brodin L, et al. Colocalization of synapsin and actin during synaptic vesicle recycling. J Cell Biol 2003; 161:737 - 47; http://dx.doi.org/10.1083/jcb.200212140; PMID: 12756235
- Morales M, Colicos MA, Goda Y. Actin-dependent regulation of neurotransmitter release at central synapses. Neuron 2000; 27:539 - 50; http://dx.doi.org/10.1016/S0896-6273(00)00064-7; PMID: 11055436
- Colicos MA, Collins BE, Sailor MJ, Goda Y. Remodeling of synaptic actin induced by photoconductive stimulation. Cell 2001; 107:605 - 16; http://dx.doi.org/10.1016/S0092-8674(01)00579-7; PMID: 11733060
- Stavoe AK, Nelson JC, Martínez-Velázquez LA, Klein M, Samuel AD, Colón-Ramos DA. Synaptic vesicle clustering requires a distinct MIG-10/Lamellipodin isoform and ABI-1 downstream from Netrin. Genes Dev 2012; 26:2206 - 21; http://dx.doi.org/10.1101/gad.193409.112; PMID: 23028145
- Pawson C, Eaton BA, Davis GW. Formin-dependent synaptic growth: evidence that Dlar signals via Diaphanous to modulate synaptic actin and dynamic pioneer microtubules. J Neurosci 2008; 28:11111 - 23; http://dx.doi.org/10.1523/JNEUROSCI.0833-08.2008; PMID: 18971454
- Burré J, Beckhaus T, Schägger H, Corvey C, Hofmann S, Karas M, et al. Analysis of the synaptic vesicle proteome using three gel-based protein separation techniques. Proteomics 2006; 6:6250 - 62; http://dx.doi.org/10.1002/pmic.200600357; PMID: 17080482
- Burré J, Volknandt W. The synaptic vesicle proteome. J Neurochem 2007; 101:1448 - 62; http://dx.doi.org/10.1111/j.1471-4159.2007.04453.x; PMID: 17355250
- Takamori S, Holt M, Stenius K, Lemke EA, Grønborg M, Riedel D, et al. Molecular anatomy of a trafficking organelle. Cell 2006; 127:831 - 46; http://dx.doi.org/10.1016/j.cell.2006.10.030; PMID: 17110340
- Butko MT, Savas JN, Friedman B, Delahunty C, Ebner F, Yates JR 3rd, et al. In vivo quantitative proteomics of somatosensory cortical synapses shows which protein levels are modulated by sensory deprivation. Proc Natl Acad Sci U S A 2013; 110:E726 - 35; http://dx.doi.org/10.1073/pnas.1300424110; PMID: 23382246
- Bleckert A, Photowala H, Alford S. Dual pools of actin at presynaptic terminals. J Neurophysiol 2012; 107:3479 - 92; http://dx.doi.org/10.1152/jn.00789.2011; PMID: 22457456
- Fernández-Busnadiego R, Zuber B, Maurer UE, Cyrklaff M, Baumeister W, Lucic V. Quantitative analysis of the native presynaptic cytomatrix by cryoelectron tomography. J Cell Biol 2010; 188:145 - 56; http://dx.doi.org/10.1083/jcb.200908082; PMID: 20065095
- Landis DM, Hall AK, Weinstein LA, Reese TS. The organization of cytoplasm at the presynaptic active zone of a central nervous system synapse. Neuron 1988; 1:201 - 9; http://dx.doi.org/10.1016/0896-6273(88)90140-7; PMID: 3152289
- Urban E, Jacob S, Nemethova M, Resch GP, Small JV. Electron tomography reveals unbranched networks of actin filaments in lamellipodia. Nat Cell Biol 2010; 12:429 - 35; http://dx.doi.org/10.1038/ncb2044; PMID: 20418872
- Humeau Y, Candiani S, Ghirardi M, Poulain B, Montarolo P. Functional roles of synapsin: lessons from invertebrates. Semin Cell Dev Biol 2011; 22:425 - 33; http://dx.doi.org/10.1016/j.semcdb.2011.07.018; PMID: 21843652
- Fornasiero EF, Bonanomi D, Benfenati F, Valtorta F. The role of synapsins in neuronal development. Cell Mol Life Sci 2010; 67:1383 - 96; http://dx.doi.org/10.1007/s00018-009-0227-8; PMID: 20035364
- Shupliakov O, Haucke V, Pechstein A. How synapsin I may cluster synaptic vesicles. Semin Cell Dev Biol 2011; 22:393 - 9; http://dx.doi.org/10.1016/j.semcdb.2011.07.006; PMID: 21798362
- Bykhovskaia M. Synapsin regulation of vesicle organization and functional pools. Semin Cell Dev Biol 2011; 22:387 - 92; http://dx.doi.org/10.1016/j.semcdb.2011.07.003; PMID: 21827866
- Cesca F, Baldelli P, Valtorta F, Benfenati F. The synapsins: key actors of synapse function and plasticity. Prog Neurobiol 2010; 91:313 - 48; http://dx.doi.org/10.1016/j.pneurobio.2010.04.006; PMID: 20438797
- Kovar DR, Harris ES, Mahaffy R, Higgs HN, Pollard TD. Control of the assembly of ATP- and ADP-actin by formins and profilin. Cell 2006; 124:423 - 35; http://dx.doi.org/10.1016/j.cell.2005.11.038; PMID: 16439214
- Pruyne D, Evangelista M, Yang C, Bi E, Zigmond S, Bretscher A, et al. Role of formins in actin assembly: nucleation and barbed-end association. Science 2002; 297:612 - 5; http://dx.doi.org/10.1126/science.1072309; PMID: 12052901
- Zigmond SH, Evangelista M, Boone C, Yang C, Dar AC, Sicheri F, et al. Formin leaky cap allows elongation in the presence of tight capping proteins. Curr Biol 2003; 13:1820 - 3; http://dx.doi.org/10.1016/j.cub.2003.09.057; PMID: 14561409
- Romero S, Le Clainche C, Didry D, Egile C, Pantaloni D, Carlier MF. Formin is a processive motor that requires profilin to accelerate actin assembly and associated ATP hydrolysis. Cell 2004; 119:419 - 29; http://dx.doi.org/10.1016/j.cell.2004.09.039; PMID: 15507212
- Hall A. Rho GTPases and the actin cytoskeleton. Science 1998; 279:509 - 14; http://dx.doi.org/10.1126/science.279.5350.509; PMID: 9438836
- Lundquist EA. Rac proteins and the control of axon development. Curr Opin Neurobiol 2003; 13:384 - 90; http://dx.doi.org/10.1016/S0959-4388(03)00071-0; PMID: 12850224
- Murthy VN, De Camilli P. Cell biology of the presynaptic terminal. Annu Rev Neurosci 2003; 26:701 - 28; http://dx.doi.org/10.1146/annurev.neuro.26.041002.131445; PMID: 14527272
- Dhanvantari S, Arnaoutova I, Snell CR, Steinbach PJ, Hammond K, Caputo GA, et al. Carboxypeptidase E, a prohormone sorting receptor, is anchored to secretory granules via a C-terminal transmembrane insertion. Biochemistry 2002; 41:52 - 60; http://dx.doi.org/10.1021/bi015698n; PMID: 11772002
- Jacob TC, Kaplan JM. The EGL-21 carboxypeptidase E facilitates acetylcholine release at Caenorhabditis elegans neuromuscular junctions. J Neurosci 2003; 23:2122 - 30; PMID: 12657671
- Zhu X, Wu K, Rife L, Cawley NX, Brown B, Adams T, et al. Carboxypeptidase E is required for normal synaptic transmission from photoreceptors to the inner retina. J Neurochem 2005; 95:1351 - 62; http://dx.doi.org/10.1111/j.1471-4159.2005.03460.x; PMID: 16219026
- Lou H, Park JJ, Cawley NX, Sarcon A, Sun L, Adams T, et al. Carboxypeptidase E cytoplasmic tail mediates localization of synaptic vesicles to the pre-active zone in hypothalamic pre-synaptic terminals. J Neurochem 2010; 114:886 - 96; http://dx.doi.org/10.1111/j.1471-4159.2010.06820.x; PMID: 20492353
- Pielage J, Bulat V, Zuchero JB, Fetter RD, Davis GW. Hts/Adducin controls synaptic elaboration and elimination. Neuron 2011; 69:1114 - 31; http://dx.doi.org/10.1016/j.neuron.2011.02.007; PMID: 21435557
- Südhof TC. The presynaptic active zone. Neuron 2012; 75:11 - 25; http://dx.doi.org/10.1016/j.neuron.2012.06.012; PMID: 22794257
- Gundelfinger ED, Fejtova A. Molecular organization and plasticity of the cytomatrix at the active zone. Curr Opin Neurobiol 2012; 22:423 - 30; http://dx.doi.org/10.1016/j.conb.2011.10.005; PMID: 22030346
- Bruckner JJ, Gratz SJ, Slind JK, Geske RR, Cummings AM, Galindo SE, et al. Fife, a Drosophila Piccolo-RIM homolog, promotes active zone organization and neurotransmitter release. J Neurosci 2012; 32:17048 - 58; http://dx.doi.org/10.1523/JNEUROSCI.3267-12.2012; PMID: 23197698
- Leal-Ortiz S, Waites CL, Terry-Lorenzo R, Zamorano P, Gundelfinger ED, Garner CC. Piccolo modulation of Synapsin1a dynamics regulates synaptic vesicle exocytosis. J Cell Biol 2008; 181:831 - 46; http://dx.doi.org/10.1083/jcb.200711167; PMID: 18519737
- Yeh E, Kawano T, Weimer RM, Bessereau JL, Zhen M. Identification of genes involved in synaptogenesis using a fluorescent active zone marker in Caenorhabditis elegans. J Neurosci 2005; 25:3833 - 41; http://dx.doi.org/10.1523/JNEUROSCI.4978-04.2005; PMID: 15829635
- Ataman B, Ashley J, Gorczyca D, Gorczyca M, Mathew D, Wichmann C, et al. Nuclear trafficking of Drosophila Frizzled-2 during synapse development requires the PDZ protein dGRIP. Proc Natl Acad Sci U S A 2006; 103:7841 - 6; http://dx.doi.org/10.1073/pnas.0600387103; PMID: 16682643