Abstract
Purpose: Recent modeling efforts, based on reported outcomes following salvage radiotherapy (SRT) for prostate cancer, predict the likelihood of biochemical control (tumor control probability, TCP) as a function of pre-treatment prostate specific antigen (PSA) and SRT dose. Similar instruments predict the risk of grade ≥ 3 late toxicity (normal tissue complication probability, NTCP) as a function of SRT dose. Here we explore how changes in the parameters of those models might affect the optimal SRT dose and clinical outcomes.
Materials and Methods: Baseline TCP and NTCP model parameters were established in a previous report. Pre-treatment PSA was set at 0.4 ng/mL. Model parameters were modified to explore four scenarios: (1) improving the safety of SRT, (2) increasing tumor cell radiosensitivity, (3) increasing the cure rate achievable with SRT and (4) adoption of hypofractionated SRT schedules. The “optimal” SRT dose, defined as the dose that maximized the likelihood of achieving biochemical control without causing late toxicity, was identified for each scenario.
Results: Improving the safety of SRT increased the optimal SRT dose, while radiosensitization decreased the optimal dose. Both changes were predicted to increase the probability of biochemical control and decrease late toxicity rates. Increasing the cure rate achievable with SRT (eg: improving patient selection or combining SRT with effective systemic therapy) provided the greatest gains in TCP. Adoption of a hypofractionated SRT schedule was predicted to improve both biochemical control and late toxicity.
Conclusions: Modeling exercises demonstrate the significant gains that may be achieved with improved implementation of SRT for prostate cancer. Strategies to realize the effects modeled in this report should be explored in clinical trials.
Keywords: :
Introduction
Each year, approximately 100,000 men in the United States undergo prostatectomy for localized prostate cancer. Approximately one in five patients will develop recurrence after prostatectomy,Citation1 and relapse rates are over 50% for patients found to have adverse pathologic features (extracapsular extension, seminal vesicle invasion or positive surgical margins) at the time of prostatectomy.Citation2 While adjuvant radiotherapy is recommended for patients with adverse pathologic features based on the results of three randomized trials,Citation3-Citation5 clinicians often opt to monitor postoperative prostate specific antigen (PSA) levels and initiate salvage radiotherapy (SRT) for cases of biochemical recurrence.Citation6
Unfortunately, SRT is not always effective in achieving biochemical or clinical disease control. Reported 5-y biochemical control rates following SRT range from 25–70%.Citation7 Retrospective analyses have identified a number of factors that may influence the efficacy of SRT. These include PSA at the time of SRT, SRT dose, PSA doubling time following prostatectomy, Gleason score, surgical margin status and use of androgen deprivation therapy.Citation8-Citation11 SRT can also cause significant toxicity. Reported rates of late grade ≥ 3 complications following SRT range from 1–13%.Citation7
We recently reviewed published SRT series and developed a tumor control probability (TCP) model to predict the likelihood of 5-y biochemical disease control following SRT as a function of radiotherapy dose and PSA at the time of SRT.Citation7 We also generated normal tissue complication probability (NTCP) models to predict the probability of severe (grade ≥ 3) gastrointestinal (GI) and/or genitourinary (GU) toxicity based on SRT dose. Those models can be used to predict outcomes when conventional approaches to patient selection and treatment are used. Advances in the implementation of SRT that increase treatment efficacy, decrease toxicity rates or improve patient selection could enhance the therapeutic ratio of SRT. Such advances could be regarded as changes in the parameters in our modeling exercises.
In this report we utilize TCP and NTCP modeling to explore how changes in model parameters may affect outcomes following SRT. We identify the parameters that most significantly modulate TCP in relation to NTCP. This may reveal the directions for future clinical trials that are most likely to provide meaningful gains for patients treated with SRT.
Results
depicts TCP and NTCP (as functions of SRT dose) using baseline parameter values. The optimal “success” rate was found with an SRT dose of 66 Gy. This provided a 61% chance of tumor control, a 5% chance of severe toxicity and a 58% chance of tumor control without toxicity
Figure 1. TCP and NTCP models using baseline parameters. The optimal SRT dose in this scenario is 66 Gy. This provides a 61% chance of tumor control, 5% chance of severe toxicity and a 58% chance of “success” (tumor control without toxicity).
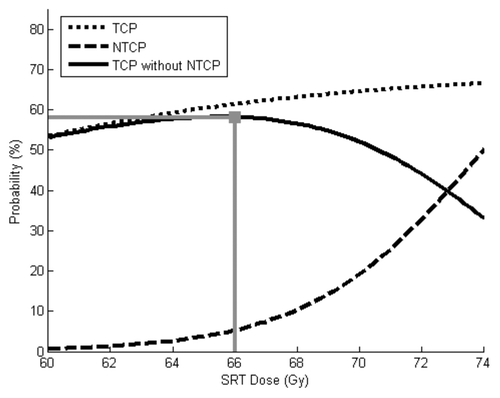
The effects of increasing the TD50 from 74 Gy to 80 Gy are shown in . The optimal SRT dose in that scenario was 70 Gy. Rates of TCP, NTCP, and “success” with that dose were 65%, 3% and 63%, respectively. Thus, increasing TD50 allowed SRT dose escalation such that both disease control rates and toxicity rates were improved.
Figure 2. TCP and NTCP models using baseline parameters, except TCD50 is increased from 74 Gy to 80 Gy. The optimal SRT dose in this scenario is 70 Gy. This provides a 65% chance of tumor control, 3% chance of severe toxicity and a 63% chance of “success.”
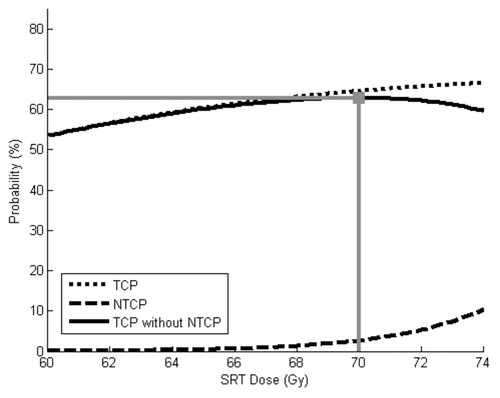
Increasing α from 0.12 Gy−1 by 25% to 0.15 Gy−1 led to different results. The optimal “success” rate was seen with a lower SRT dose of 62 Gy. This provided a 68% chance of tumor control, a 1% chance of severe toxicity and a 67% chance of tumor control without toxicity. Again, adjustment of a single model parameter (cancer cell radiosensitivity) led to improvements in both TCP and NTCP.
The effects of increasing K from 0.70 to 0.85 are shown in . 66 Gy maximized the “success” rate in that scenario, with rates of TCP, NTCP and “success” of 75%, 5% and 71%, respectively. Of note, this scenario provided the greatest likelihood of biochemical disease control without toxicity.
Figure 3. TCP and NTCP models using baseline parameters, except K is increased from 0.70 to 0.85. The optimal SRT dose in this scenario is 66 Gy. This provides a 75% chance of tumor control, 5% chance of severe toxicity and a 71% chance of “success.”
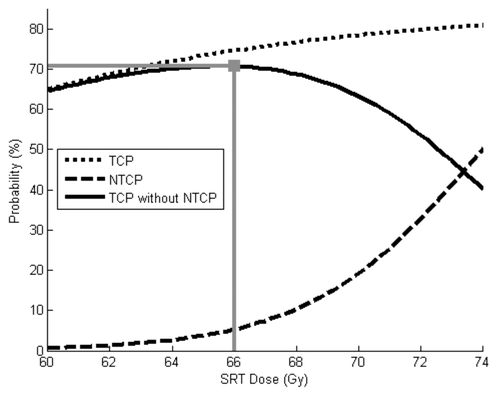
TCP and NTCP modeling results using hypofractionated SRT are shown in . Using fraction sizes of 2.5 Gy, the optimal SRT dose was 60 Gy. This provided a 64% chance of tumor control, a 5% chance of severe toxicity and a 60% chance of tumor control without toxicity. () Results of more drastic hypofractionation using 5-fraction SRT schedules are shown in . The optimal 5-fraction dose was 32.5 Gy (6.5 Gy x 5). This yielded a 67% chance of tumor control, a 1% chance of severe toxicity and a 66% chance of tumor control without toxicity. Thus, both TCP and NTCP improved as radiotherapy fraction size was increased.
Figure 4. (A) TCP and NTCP models using baseline parameters and mildly hypofractionated SRT schedules (2.5 Gy per fraction). The optimal SRT dose in this scenario 60 Gy (gray boxes). This provides a 64% chance of tumor control, a 5% chance of severe toxicity and a 60% chance of “success.” (B) TCP and NTCP models using baseline parameters and 5-fraction SRT schedules. The optimal SRT dose this scenario is 32.5 Gy (6.5 Gy x 5, gray boxes). This provides a 67% chance of tumor control, a 1% chance of severe toxicity and a 66% chance of “success.”
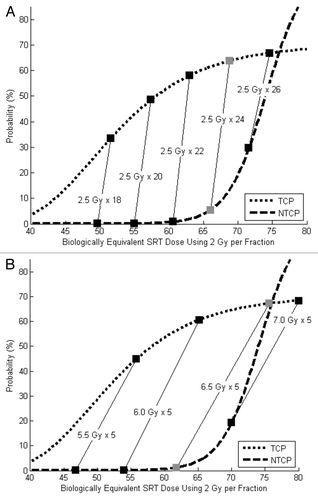
Discussion
In this exercise, we demonstrated how changes in the parameters of TCP and NTCP models for SRT for prostate cancer can affect the optimal SRT dose, the likelihood of biochemical disease control and the risk of severe toxicity. The four changes that we modeled were: (1) improving the safety of SRT, (2) increasing the tumor cell radiosensitivity, (3) increasing the cure rate achievable with SRT and (4) adoption of hypofractionated SRT schedules. While the modifications that we applied to our baseline model parameters are inherently theoretical, real-world improvements in the implementation of SRT might be expected to have similar effects. Many such advancements are the subject of preclinical and clinical study.
The first change we examined was an increase in the TD50 (). Graphically, this can be viewed as a rightward shift of the NTCP curve. Our findings indicate that this should allow safe dose escalation, leading to improvements in both disease control and expected toxicity. Since the baseline model parameters were based on toxicity rates following 3-dimensional conformal radiotherapy, a clinical correlate to the scenario described in might be the delivery of SRT using dose-escalated intensity-modulated radiotherapy (IMRT). This strategy has already been adopted by many clinicians. In a recent survey of United States radiation oncologists, the majority of respondents indicated that they use IMRT for postoperative radiotherapy and most utilize SRT doses of 70 Gy or higher.Citation6 Several groups have recently published results of dose-escalated SRT using IMRT, and their toxicity rates are consistent with those predicted in this analysis.Citation12-Citation14 Other changes in treatment strategy that may effectively increase the TD50 are the adoption of image-guided radiotherapy (IGRT),Citation15 or the administration of radioprotective agents.Citation16
Next, we modeled the effects of increasing tumor cell radiosensitivity. This allowed SRT dose de-escalation, again providing improvements in both TCP and NTCP. One way to achieve such gains in a clinical setting might be with the addition of a radiosensitizing agent to SRT. Some groups have reported early-phase results with the combination of taxane-based chemotherapy and radiotherapy as definitive treatment for intermediate or high-risk prostate cancer.Citation17 Several similar trials for the salvage setting are already underway. Of course, our modeling exercise assumed that radiosensitization of tumor cells could be achieved without increasing toxicity. Clinical study is required to identify agents that radiosensitize tumor cells preferentially over normal tissues in the setting of SRT.
The third scenario we explored was an increase in the term K, which represents the maximum achievable biochemical control rate following SRT. Others have noted that this value seems to peak around 75%.Citation8 In our previous modeling study, we found a similar value of approximately 70%.Citation7 Presumably, this is because approximately 30% of seemingly appropriate candidates for SRT have occult extrapelvic disease that cannot be detected with standard workup (CT scan, bone scan) and cannot be cured with SRT. Thus, the value of K might be increased if patients with occult metastatic disease could be identified and spared from unnecessary local therapy. This may one day be achieved with the use of novel functional imaging modalities such as 11C-choline PET.Citation18 Alternatively, addition of effective systemic therapy to SRT might increase the proportion of patients who can be cured in the salvage setting. In our opinion, both of these strategies are worthy of clinical study, as our modeling exercises showed that increasing K provided the greatest gains in TCP and overall treatment “success.”
The final situation we examined was a shift to hypofractionated SRT. Based on a wide body of literature indicating that prostate cancer cells are extremely sensitive to radiotherapy fraction size,Citation19,Citation20 hypofractionation of radiotherapy may yield therapeutic gains for prostate cancer patients. As one might expect, our modeling revealed that the gains from gentle hypofractionation using fraction sizes of 2.5 Gy were modest. More aggressive hypofractionation, using a regimen of 6.5 Gy x 5, however, provided significant improvements in both tumor control and expected toxicity. Trials of gentle hypofractionation for SRT have already been reported.Citation21,Citation22 Based on our findings, careful clinical study of more aggressive hypofractionated schedules may be warranted. We find this strategy especially appealing because it requires no technologic or scientific advancement per se and may lead to more efficient management of this patient population.
summarizes the advances we have modeled in this paper and the effects they are predicted to have on optimal SRT dose, disease control probability and toxicity rates. We also provide some examples of how those changes might be achieved in a clinical setting. Technological advances in SRT delivery, including adoption of IGRT and IMRT, have already been implemented by many clinicians to decrease toxicity rates. Other strategies, such as the combination of systemic therapy and SRT, are the subject of preclinical and clinical study.
Table 1. Summary of modeling results and possible clinical correlates
There are several limitations to the applicability of our study that should be mentioned. One is that our definition of treatment “success” as achievement of biochemical disease control without causing severe late toxicity was somewhat arbitrary. Some may believe, for example, that failure to achieve disease control is more impactful to a patient than a transient late side effect of treatment. Others may argue that biochemical control is of little importance to a patient, and SRT toxicity should be avoided at all costs. Another major limitation of this study is that it was predicated on a pair of models whose parameters are by no means set in stone; use of different baseline assumptions would clearly alter the results. While the baseline model parameters were extracted from published salvage radiotherapy data, the improved parameter values are largely theoretical. This limits the validity of comparing outcomes between scenarios. Modeling NTCP as a function of prescription dose rather than partial volume doses to relevant organs at risk is not optimalCitation23 but is required based on the available data. Finally, our analysis of hypofractionated SRT schedules did not account for changes in overall treatment time. Clinical study is needed to determine how shortening the course of SRT from 6–7 weeks to as little as 5 d affects treatment efficacy and tolerability.
Conclusion
We have used TCP and NTCP models to describe the clinical gains that may be achieved with improved implementation of SRT for prostate cancer. Our findings suggest that improved identification of patients who may be cured by SRT or effective treatment of patients with occult metastatic disease may yield significant gains for this patient population. Hypofractionation of SRT may be a simple way to improve the risk-benefit ratio of SRT and should be studied clinically.
Materials and Methods
Baseline model parameters
We have previously described the formulation of TCP and NTCP models for the setting of SRT for prostate cancer.Citation7 These models are: TCP = K * (1 – e-α*D)C*PSA and NTCP = e([D-TD50]/k) ÷ [1 + e([D-TD50]/k)], where K is the maximum achievable TCP using SRT (70%), α is prostate cancer cell radiosensitivity (0.12 Gy−1), D is SRT dose (in 2 Gy fractions, variable), C is the number of prostate cancer cells per unit PSA at the time of SRT (900 per ng/mL), TD50 is the SRT dose that would yield a 50% complication rate (74 Gy), and k is a fitting constant for the NTCP curve (3Gy). TCP in this setting refers to the probability of biochemical disease control at five years. For this exercise, we assumed that SRT is initiated at a PSA level of 0.4 ng/mL. NTCP model parameters for combined (GI+GU) grade ≥ 3 toxicity were used. For each tested SRT dose, we calculated the probability of achieving biochemical disease control, the probability of causing grade ≥ 3 toxicity, and the probability of treatment “success,” defined as achieving biochemical disease control without causing severe toxicity (see below). The SRT dose that maximized that probability was identified. “Success” Rate = TCP * (1-NTCP)
Modeling improvements in SRT delivery
We repeated the above exercise following three modifications of the baseline model parameters. In each case, one variable was altered from its original value. The changes we tested were:
• Increasing TD50 from 74 Gy to 80 Gy (decreasing toxicity of SRT)
• Increasing α from 0.12 Gy−1 to 0.15 Gy−1 (increasing tumor cell radiosensitivity)
• Increasing K from 0.70 to 0.85 (increasing achievable cure rate using SRT)
The fourth scenario that we examined was a shift to hypofractionated SRT. We tested two types of hypofractionation. First, we examined mildly hypofractionated SRT schedules using daily doses of 2.5 Gy, as this fraction size has been utilized in recent clinical trials.Citation21,Citation22 We tested total doses of 45, 50, 55, 60 and 65 Gy (18 to 26 fractions). Next, we tested more aggressive hypofractionated schema using a total of only five fractions, as this strategy is being tested by numerous groups as primary treatment for localized prostate cancer.Citation24 Fraction sizes of 5.5 Gy, 6.0 Gy, 6.5 Gy and 7.0 Gy were used (total doses of 27.5 to 35 Gy). Since our TCP and NTCP models are based on experiences using conventional fraction sizes of approximately 2.0 Gy, we utilized the Linear Quadratic Model to calculate biologically equivalent doses for each hypofractionated schedule.Citation25 Biologically Equivalent Dose = nominal dose x (fraction size + α/β) ÷ (2 Gy + α/β)
An α/β ratio of 1.4 Gy was used for prostate cancer cells,Citation19 and a value of 3.0 Gy was used for normal tissues. Thus, each hypofractionated schedule yielded two values of “D” – one for the TCP calculation and a second (lower) value for the NTCP calculation. No adjustments were made for changes in overall treatment duration in this analysis.
Following each modification, we recalculated the probability of TCP, NTCP and treatment “success” over a range of SRT doses. The maximum achievable “success” rate and the corresponding SRT dose in each scenario were identified. We compared the relative effects of each parameter change on optimal SRT dosing, the likelihood of achieving biochemical disease control, the chance of causing severe toxicity and the probability of achieving disease control without toxicity (“success”).
Disclosure of Potential Conflicts of Interest
No potential conflicts of interest were disclosed.
References
- Han M, Partin AW, Pound CR, Epstein JI, Walsh PC. Long-term biochemical disease-free and cancer-specific survival following anatomic radical retropubic prostatectomy. The 15-year Johns Hopkins experience. Urol Clin North Am 2001; 28:555 - 65; http://dx.doi.org/10.1016/S0094-0143(05)70163-4; PMID: 11590814
- Swanson GP, Riggs M, Hermans M. Pathologic findings at radical prostatectomy: risk factors for failure and death. Urol Oncol 2007; 25:110 - 4; http://dx.doi.org/10.1016/j.urolonc.2006.06.003; PMID: 17349524
- Thompson IM, Tangen CM, Paradelo J, Lucia MS, Miller G, Troyer D, et al. Adjuvant radiotherapy for pathological T3N0M0 prostate cancer significantly reduces risk of metastases and improves survival: long-term followup of a randomized clinical trial. J Urol 2009; 181:956 - 62; http://dx.doi.org/10.1016/j.juro.2008.11.032; PMID: 19167731
- Bolla M, van Poppel H, Collette L, van Cangh P, Vekemans K, Da Pozzo L, et al, European Organization for Research and Treatment of Cancer. Postoperative radiotherapy after radical prostatectomy: a randomised controlled trial (EORTC trial 22911). Lancet 2005; 366:572 - 8; http://dx.doi.org/10.1016/S0140-6736(05)67101-2; PMID: 16099293
- Wiegel T, Bottke D, Steiner U, Siegmann A, Golz R, Störkel S, et al. Phase III postoperative adjuvant radiotherapy after radical prostatectomy compared with radical prostatectomy alone in pT3 prostate cancer with postoperative undetectable prostate-specific antigen: ARO 96-02/AUO AP 09/95. J Clin Oncol 2009; 27:2924 - 30; http://dx.doi.org/10.1200/JCO.2008.18.9563; PMID: 19433689
- Showalter TN, Ohri N, Teti KG, Foley KA, Keith SW, Trabulsi EJ, et al. Physician Beliefs and Practices for Adjuvant and Salvage Radiation Therapy After Prostatectomy. Int J Radiat Oncol Biol Phys 2012; 82:e233 - 8; http://dx.doi.org/10.1016/j.ijrobp.2011.04.003; PMID: 21605945
- Ohri N, Dicker AP, Trabulsi EJ, Showalter TN. Can early implementation of salvage radiotherapy for prostate cancer improve the therapeutic ratio? A systematic review and regression meta-analysis with radiobiological modelling. Eur J Cancer 2012; 48:837 - 44; http://dx.doi.org/10.1016/j.ejca.2011.08.013; PMID: 21945099
- King CR. Adjuvant radiotherapy after prostatectomy: does waiting for a detectable prostate-specific antigen level make sense?. Int J Radiat Oncol Biol Phys 2011; 80:1 - 3; http://dx.doi.org/10.1016/j.ijrobp.2010.10.073; PMID: 21277112
- King CR, Spiotto MT. Improved outcomes with higher doses for salvage radiotherapy after prostatectomy. Int J Radiat Oncol Biol Phys 2008; 71:23 - 7; http://dx.doi.org/10.1016/j.ijrobp.2007.09.047; PMID: 18207668
- Stephenson AJ, Scardino PT, Kattan MW, Pisansky TM, Slawin KM, Klein EA, et al. Predicting the outcome of salvage radiation therapy for recurrent prostate cancer after radical prostatectomy. J Clin Oncol 2007; 25:2035 - 41; http://dx.doi.org/10.1200/JCO.2006.08.9607; PMID: 17513807
- Valicenti RK, Gomella LG, Ismail M, Mulholland SG, Strup S, Petersen RO, et al. Durable efficacy of early postoperative radiation therapy for high-risk pT3N0 prostate cancer: the importance of radiation dose. Urology 1998; 52:1034 - 40; http://dx.doi.org/10.1016/S0090-4295(98)00405-1; PMID: 9836551
- Ost P, Lumen N, Goessaert AS, Fonteyne V, De Troyer B, Jacobs F, et al. High-dose salvage intensity-modulated radiotherapy with or without androgen deprivation after radical prostatectomy for rising or persisting prostate-specific antigen: 5-year results. Eur Urol 2011; 60:842 - 9; http://dx.doi.org/10.1016/j.eururo.2011.04.021; PMID: 21514039
- Goenka A, Magsanoc JM, Pei X, Schechter M, Kollmeier M, Cox B, et al. Improved toxicity profile following high-dose postprostatectomy salvage radiation therapy with intensity-modulated radiation therapy. Eur Urol 2011; 60:1142 - 8; http://dx.doi.org/10.1016/j.eururo.2011.08.006; PMID: 21855208
- Ohri N, Dicker AP, Showalter TN. Reply letter to: Salvage radiotherapy: A plea for dose-escalation with intensity modulated radiotherapy. Eur J Cancer 2012; 48:837 - 44; http://dx.doi.org/10.1016/j.ejca.2011.08.013; PMID: 21945099
- Sandhu A, Sethi R, Rice R, Wang JZ, Marcus L, Salem C, et al. Prostate bed localization with image-guided approach using on-board imaging: reporting acute toxicity and implications for radiation therapy planning following prostatectomy. Radiother Oncol 2008; 88:20 - 5; http://dx.doi.org/10.1016/j.radonc.2008.05.009; PMID: 18524399
- Singh AK, Ménard C, Guion P, Simone NL, Smith S, Crouse NS, et al. Intrarectal amifostine suspension may protect against acute proctitis during radiation therapy for prostate cancer: a pilot study. Int J Radiat Oncol Biol Phys 2006; 65:1008 - 13; http://dx.doi.org/10.1016/j.ijrobp.2006.02.030; PMID: 16730138
- Perrotti M, Doyle T, Kumar P, McLeod D, Badger W, Prater S, et al. Phase I/II trial of docetaxel and concurrent radiation therapy in localized high risk prostate cancer (AGUSG 03-10). Urol Oncol 2008; 26:276 - 80; http://dx.doi.org/10.1016/j.urolonc.2007.04.003; PMID: 18452819
- Martini T, Mayr R, Trenti E, Palermo S, Comploj E, Pycha A, et al. The Role of C-Choline-PET/CT-Guided Secondary Lymphadenectomy in Patients with PSA Failure after Radical Prostatectomy: Lessons Learned from Eight Cases. Adv Urol 2012; 2012:601572; http://dx.doi.org/10.1155/2012/601572; PMID: 21822429
- Miralbell R, Roberts SA, Zubizarreta E, Hendry JH. Dose-Fractionation Sensitivity of Prostate Cancer Deduced from Radiotherapy Outcomes of 5,969 Patients in Seven International Institutional Datasets: alpha/beta = 1.4 (0.9-2.2) Gy. Int J Radiat Oncol Biol Phys 2012; 82:e17 - 24; http://dx.doi.org/10.1016/j.ijrobp.2010.10.075; PMID: 21324610
- Brenner DJ, Hall EJ. Fractionation and protraction for radiotherapy of prostate carcinoma. Int J Radiat Oncol Biol Phys 1999; 43:1095 - 101; http://dx.doi.org/10.1016/S0360-3016(98)00438-6; PMID: 10192361
- Kruser TJ, Jarrard DF, Graf AK, Hedican SP, Paolone DR, Wegenke JD, et al. Early hypofractionated salvage radiotherapy for postprostatectomy biochemical recurrence. Cancer 2011; 117:2629 - 36; http://dx.doi.org/10.1002/cncr.25824; PMID: 21656740
- Lee LW, McBain CA, Swindell R, Wylie JP, Cowan RA, Logue JP. Hypofractionated radiotherapy as salvage for rising prostate-specific antigen after radical prostatectomy. Clin Oncol (R Coll Radiol) 2004; 16:517 - 22; http://dx.doi.org/10.1016/j.clon.2004.07.006; PMID: 15630843
- Niemierko A, Goitein M. Calculation of normal tissue complication probability and dose-volume histogram reduction schemes for tissues with a critical element architecture. Radiother Oncol 1991; 20:166 - 76; http://dx.doi.org/10.1016/0167-8140(91)90093-V; PMID: 1852908
- King C. Stereotactic body radiotherapy for prostate cancer: current results of a phase II trial. Front Radiat Ther Oncol 2011; 43:428 - 37; http://dx.doi.org/10.1159/000322507; PMID: 21625167
- Fowler JF. The linear-quadratic formula and progress in fractionated radiotherapy. Br J Radiol 1989; 62:679 - 94; http://dx.doi.org/10.1259/0007-1285-62-740-679; PMID: 2670032