Abstract
Previously, we proposed that cancer cells behave as metabolic parasites, as they use targeted oxidative stress as a “weapon” to extract recycled nutrients from adjacent stromal cells. Oxidative stress in cancer-associated fibroblasts triggers autophagy and mitophagy, resulting in compartmentalized cellular catabolism, loss of mitochondrial function, and the onset of aerobic glycolysis, in the tumor stroma. As such, cancer-associated fibroblasts produce high-energy nutrients (such as lactate and ketones) that fuel mitochondrial biogenesis, and oxidative metabolism in cancer cells. We have termed this new energy-transfer mechanism the “reverse Warburg effect.” To further test the validity of this hypothesis, here we used an in vitro MCF7-fibroblast co-culture system, and quantitatively measured a variety of metabolic parameters by FACS analysis (analogous to laser-capture micro-dissection). Mitochondrial activity, glucose uptake, and ROS production were measured with highly-sensitive fluorescent probes (MitoTracker, NBD-2-deoxy-glucose, and DCF-DA). Interestingly, using this approach, we directly show that cancer cells initially secrete hydrogen peroxide that then triggers oxidative stress in neighboring fibroblasts. Thus, oxidative stress is contagious (spreads like a virus) and is propagated laterally and vectorially from cancer cells to adjacent fibroblasts. Experimentally, we show that oxidative stress in cancer-associated fibroblasts quantitatively reduces mitochondrial activity, and increases glucose uptake, as the fibroblasts become more dependent on aerobic glycolysis. Conversely, co-cultured cancer cells show significant increases in mitochondrial activity, and corresponding reductions in both glucose uptake and GLUT1 expression. Pre-treatment of co-cultures with extracellular catalase (an anti-oxidant enzyme that detoxifies hydrogen peroxide) blocks the onset of oxidative stress, and potently induces the death of cancer cells, likely via starvation. Given that cancer-associated fibroblasts show the largest increases in glucose uptake, we suggest that PET imaging of human tumors, with Fluoro-2-deoxy-D-glucose (F-2-DG), may be specifically detecting the tumor stroma, rather than epithelial cancer cells.
Introduction
Recently, we proposed a new mechanism for understanding the Warburg effect in tumor metabolism.Citation1–Citation3 In this new paradigm, cancer cells induce oxidative stress in neighboring cancer-associated fibroblasts.Citation4,Citation5 Then, oxidative stress, in cancer-associated fibroblasts, triggers the activation of two main transcription factors, NFκB and HIF-1α, leading to the onset of inflammation, autophagy, mitophagy and aerobic glycolysis in the tumor microenvironment.Citation5–Citation7 This type of stromal metabolism then produces high-energy nutrients (lactate, ketones and glutamine), as well as recycled chemical building blocks (nucleotides, amino acids, fatty acids), to literally “feed” cancer cells.Citation4,Citation8 We also demonstrated that oxidative stress and ROS, produced in cancer-associated fibroblasts, has a “bystander effect” on adjacent cancer cells, leading to DNA damage, genomic instability and aneuploidy, which appears to be driving tumor-stroma co-evolution.Citation5,Citation9 However, it is not clear how tumor cells induce oxidative stress in cancer-associated fibroblasts, which we have demonstrated is the first step in the initiation of this cascade, that drives tumor-stroma metabolic coupling. Understanding this initiating step may lead to the development of new therapeutic strategies for cancer prevention. We have previously termed this new paradigm the “reverse Warburg effect,” as we see that aerobic glycolysis takes place in cancer-associated fibroblasts, rather than in tumor cells, as previously suspected.Citation1
To further validate this new hypothesis, here we used an established MCF7-fibroblast co-culture system to understand the metabolic processes underlying the cross-talk between fibroblasts and cancer cells.Citation10 These co-cultures were subjected to quantitative FACS analysis, to determine the levels of mitochondrial activity, glucose uptake and ROS production in tumor cells vs. cancer-associated fibroblasts.
Our results indicate that tumor cells produce and secrete hydrogen peroxide, thereby “fertilizing” the tumor microenvironment and driving the “reverse Warburg effect.” As a consequence, oxidative stress initiated in tumor cells is transferred to cancer-associated fibroblasts. Then, cancer-associated fibroblasts show quantitative reductions in mitochondrial activity and compensatory increases in glucose uptake, as well as high ROS production.
Interruption of this process, by addition of catalase (an enzyme that detoxifies hydrogen peroxide) to the tissue culture media, blocks ROS activity in cancer cells and leads to apoptotic cell death in cancer cells, likely due to their inability to extract recycled nutrients from cancer-associated fibroblasts.
Thus, reducing oxidative stress with powerful antioxidants, is an important strategy for cancer prevention, as it would suppress one of the key early initiating steps where DNA damage and tumor-stroma metabolic-coupling begins. This would prevent cancer cells from acting as metabolic “parasites.”Citation11 In addition, complementary therapies targeting active mitochondria in cancer cells, via mitophagy, would also be beneficial.Citation12
During wound healing, non-cancerous epithelial cells produce and secrete hydrogen peroxide, as part of the normal wound healing response, that alerts the immune system and surrounding fibroblasts.Citation13 Thus, cancer cells have “usurped” a known regulated normal physiological process, to physically generate a wound that does not heal. By secreting hydrogen peroxide, cancer cells may be masquerading as cells of the immune system (neutrophils and macrophages), which are the main producers of hydrogen peroxide, which further potentiates inflammation and DNA damage. In this regard, our results may also explain the “field effect” in cancer biology,Citation5 as hydrogen peroxide secreted by cancer cells, and the propagation of ROS production, from cancer cells to fibroblasts, would create an increasing “mutagenic field” of ROS production, due to the resulting DNA damage.
Our findings also have implications for the clinical imaging of human tumors, as medical oncologists and radiologists routinely use PET scanning to follow glucose uptake in human tumors.Citation14–Citation21 Here, we show that cancer-associated fibroblasts have the largest increases in glucose uptake, secondary to mitochondrial dysfunction. Thus, PET scanning with 2-[18F]-2-deoxy-D-glucose (18F-FDG) may be an ideal way to visualize the “reverse Warburg effect,” in cancer-associated fibroblasts, in human tumors in vivo.
Similarly, we show that a loss of stromal caveolin (Cav-1) is sufficient to drive mitochondrial dysfunction with increased glucose uptake in fibroblasts, mimicking the glycolytic phenotype of cancer-associated fibroblasts. These findings may explain the prognostic value of a loss of stromal Cav-1 as a marker of a “lethal” tumor microenvironment.Citation22–Citation30
For recent reviews on either Cav-1 or the conventional Warburg effect, please see the following references.Citation31–Citation33
Results
Measuring metabolic activity during tumor-stroma co-evolution.
We have previously documented, using antibody immuno-staining and confocal microscopy, that there are significant changes in cancer metabolism during tumor-stroma co-evolution.Citation5–Citation7,Citation10 To directly measure these metabolic changes in a functional and quantitative manner, we have now shifted our focus to FACS analysis with fluorescent probes (). For this purpose, MCF7 cells were co-cultured with fibroblasts for 5 d, and then incubated with specific well-established probes to measure mitochondrial activity, glucose uptake or ROS production. The two cell types were then separated by mild-trypsinization and FACS analysis, akin to laser-capture microdissection. MCF7 cancer cells were recombinantly-tagged with either GFP or RFP, allowing their recognition by FACS.
During co-culture, mitochondrial activity increases in cancer cells, and is reduced in stromal fibroblasts.
shows the FACS tracings for mitochondrial activity (MitoTracker red fluorescence) in fibroblasts as well as cancer cells, either cultured alone or in combination. Interestingly, during co-culture, MCF7 cells show a shift to the right, which corresponds to a >two-fold increase in mitochondrial activity ( and B). Conversely, during co-culture, stromal fibroblasts show just the opposite effect, with a shift to the left, resulting in a 1.7-fold reduction in mitochondrial activity ( and B).
To rule out that this metabolic behavior only occurred in MCF7 cancer cells, we also used MDA-MB-231 cells, another well-established human breast cancer cell line. shows that quantitatively similar results were obtained with MDA-MB-231-fibroblast co-cultures, with a 1.5-fold increase in mitochondrial activity in cancer cells, and a >five-fold reduction in mitochondrial activity in stromal fibroblasts ().
During co-culture, fibroblasts show increased glucose uptake, while cancer cells show less reliance on glucose.
shows the FACS tracings for NBD-2-deoxy-glucose uptake in fibroblasts or cancer cells, either cultured alone or in combination. During co-culture, stromal fibroblasts show a shift to the right, which corresponds to a 1.8-fold increase in glucose uptake. However, during co-culture, cancer cells show just the opposite phenotype, with a shift to the left, resulting in a 1.6-fold reduction in glucose uptake ( and B).
These observations are consistent with our results obtained with MitoTracker, indicating that fibroblasts have reduced mitochondrial activity and an increased avidity for glucose, indicative of a shift toward aerobic glycolysis. Conversely, MCF7 cancer cells show increased mitochondrial activity and a reduced avidity for glucose, due to a shift toward oxidative mitochondrial metabolism.
Monitoring ROS during tumor-stroma co-evolution: ROS production occurs mainly in stromal fibroblasts.
We have previously hypothesized that the “root cause” for changes in cancer metabolism can be traced to ROS production, and the resulting loss of mitochondrial function in stromal fibroblasts.Citation2–Citation5,Citation34 To further test this hypothesis, MCF7 cancer cells and stromal fibroblasts were co-cultured for 5 d, and then subjected to FACS analysis, with the fluorescent probe DCF-DA, which measures ROS production.
shows the FACS tracings for ROS production. Interestingly, at day 5 of co-culture, we see that stromal fibroblasts show a shift to the right, which corresponds to a >two-fold increase in ROS production ( and B). In contrast, cancer cells show only a 1.2-fold increase in ROS production, under the same conditions.
Thus, during co-culture, stromal fibroblasts show increases in ROS production and glucose uptake, and a corresponding decrease in mitochondrial activity.
Cancer cells initially secrete hydrogen peroxide, transferring ROS production from cancer cells to adjacent stromal fibroblasts.
Because cancer cells can secrete hydrogen peroxide,Citation35–Citation37 we wondered if ROS production in cancer cells occurs earlier during co-culture, and then spreads laterally and vectorially to fibroblasts. To test this hypothesis, we measured ROS production in 2-d co-cultures.
shows that after 2 d of co-culture, MCF7 cancer cells show a near three-fold induction in ROS production, while stromal fibroblasts have only minor increases in ROS production. Consistent with the hypothesis that this indeed represents the secretion of hydrogen peroxide into the culture media,Citation38 addition of extracellular catalase (an enzyme that detoxifies hydrogen peroxide to oxygen and water) was sufficient to normalize ROS associated with co-cultured cancer cells. Under these conditions, an irrelevant protein (namely BSA) had no effect ( and B).
Thus, in this co-culture system, it appears that ROS production starts in cancer cells (at day 2 or earlier), via the generation and secretion of hydrogen peroxide, and then spreads laterally to adjacent stromal fibroblasts (as observed at day 5 co-culture).
Catalase induces apoptosis in co-cultured MCF7 cells.
Our working hypothesis is that cancer cells use oxidative stress in cancer-associated fibroblasts to maintain access to a steady-stream of high-energy nutrients, via stromal autophagy and aerobic glycolysis. Thus, it follows that antioxidants might cut off a cancer cell's fuel supply, likely resulting in the death of cancer cells via apoptosis.
To test this hypothesis directly, we assessed apoptosis in MCF7 cells after addition of extracellular catalase to the co-culture, to detoxify hydrogen peroxide and prevent oxidative stress. shows that the simple addition of catalase to the culture media induces a five-fold increase in apoptotic cell death in co-cultured cancer cells; however, an irrelevant protein (namely BSA) had no effect ( and B).
Thus, we believe that catalase may induce apoptosis in co-cultured cancer cells, by detoxifying extracellular hydrogen peroxide, effectively cutting off the fuel supply for cancer cells.
Comparison of metabolic parameters in fibroblasts vs. cancer cells.
We also calculated fold-changes in metabolism for fibroblasts vs. MCF7 cells (Fibro/MCF7), under conditions of single cell type (homotypic) cultures and after co-culture. These results are summarized in . This type of quantitative analysis allows a direct comparison of relative metabolic activity in cancer cells and fibroblasts, under the same conditions.
Five days co-culture: fibroblasts vs. MCF7 cells. Mitochondrial activity. In single cell cultures, fibroblasts have roughly twice the mitochondrial activity of MCF7 cells. However, in co-cultures, fibroblasts have nearly half the mitochondrial activity of MCF7 cells. This represents a large change in relative mitochondrial activity in fibroblasts (a near four-fold reduction).
Glucose uptake. In single cell cultures, fibroblasts and MCF7 cells show the same relative glucose uptake. However, in co-cultures, fibroblasts show a near three-fold increase in glucose uptake, relative to MCF7 cells.
ROS production. In single cell cultures, fibroblasts and MCF7 cells show the same relative ROS production. However, in co-cultures, fibroblasts show a two-fold increase in ROS production, relative to MCF7 cells.
Thus, relative to MCF7 cells, co-cultured fibroblasts show a significant decrease in mitochondrial activity, with a compensatory increase in glucose uptake and increased ROS production.
Two days co-culture: fibroblasts vs. MCF7 cells. ROS production. In single cell cultures, fibroblasts and MCF7 cells show the same relative ROS production. However, in co-cultures, MCF7 cells show a two-fold increase in ROS production, relative to fibroblasts cells. Thus, increased ROS production starts in cancer cells (at 2 d of co-culture) and is then transferred to fibroblast (by 5 d of co-culture).
Five days co-culture: fibroblasts vs. MDA-MB-231 cells. Mitochondrial activity. In single cell cultures, fibroblasts have roughly twice the mitochondrial activity of MDA-MB-231 cells. However, in co-cultures, fibroblasts have nearly one quarter of the mitochondrial activity of MDA-MB-231 cells. This represents a large change in relative mitochondrial activity in fibroblasts (a near eight-fold reduction). These results are very similar to our observations with MCF7 cells.
In summary, during the long-term co-culture of fibroblasts with epithelial cancer cells, fibroblasts show increased ROS production, decreased mitochondrial activity and increased glucose uptake. These findings are consistent with the idea that aerobic glycolysis (i.e., the Warburg effect) actually occurs in cancer-associated fibroblasts.
Co-culture of MCF7 cells with fibroblasts leads to GLUT1 downregulation.
Given that MCF7 cells show a significant reduction in functional glucose uptake during co-culture with fibroblasts (), we also monitored the expression of GLUT1 (a major glucose transporter), using specific antibody probes.
shows the expression of GLUT1 in MCF7 cells alone, and in MCF7 cells co-cultured with fibroblasts. Note that GLUT1 expression is dramatically downregulated when MCF7 cells are co-cultured with fibroblasts, consistent with our functional results obtained by FACS analysis. However, stromal fibroblasts do not upregulate GLUT1 under these conditions. Thus, the increase we observe in glucose uptake in co-cultured fibroblasts must be due to the upregulation of another GLUT family member.
During co-culture, fibroblasts undergo aerobic glycolysis and produce high-energy metabolites, such as L-Lactate and 3-Hydroxy-Butyrate (a ketone body), due to mitochondrial dysfunction. Thus, we next assessed the ability of these high-energy metabolites, which are converted to Acetyl-CoA and can enter the TCA cycle, to affect GLUT1 expression in MCF7 cells cultured alone.
shows that treatment of MCF7 cells with either L-Lactate and 3-Hydroxy-Butyrate (10 mM for 2 d) is indeed sufficient to induce a loss of GLUT1 expression in MCF7 cells alone. Thus, treatment with either L-Lactate and 3-Hydroxy-Butyrate is sufficient to pheno-copy the effects of fibroblasts on GLUT1 expression in MCF7 cells.
Loss of caveolin-1 is sufficient to drive mitochondrial dysfunction, with increased glucose uptake, in stromal fibroblasts.
We and others have previously shown that a loss of stromal Cav-1 is associated with early tumor recurrence, metastasis, tamoxifen-resistance and poor clinical outcome in human breast cancer patients.Citation22–Citation30 Similarly, Cav-1 knock-down fibroblasts increase tumor growth, up to ∼four-fold, when they are co-injected with human breast cancer cells in a xenograft model.Citation39 Most importantly, a loss of stromal Cav-1 occurs when MCF7 cells are co-cultured with stromal fibroblasts, indicating that these co-culture conditions mimic a “lethal” tumor micro-environment.Citation10 Under these co-culture conditions, Cav-1 in fibroblasts is degraded by lysosomes in response to oxidative stress, and this can be prevented by treatment with antioxidants or autophagy inhibitors.Citation5,Citation6,Citation10 Interestingly, a loss of Cav-1 is also sufficient to induce autophagy and mitophagy,Citation2,Citation4–Citation6,Citation10 providing a feed-forward mechanism for maintaining an autophagic tumor micro-environment, driving the “reverse Warburg effect.”Citation1
To further test this hypothesis, here we examined mitochondrial activity and glucose uptake in Cav-1 knock-down fibroblasts, by FACS analysis with fluorescent probes. A prediction of our hypothesis is that a loss of Cav-1 should be sufficient to induce a reduction in mitochondrial activity, with a compensatory increase in glucose uptake, as we observe in stromal fibroblasts that are co-cultured with cancer cells.
shows that we successfully created stable Cav-1 knock-down fibroblasts, using an siRNA-based approach. As predicted, Cav-1 knock-down fibroblasts show a ∼1.8-fold reduction in mitochondrial activity, as measured with the MitoTracker probe. Conversely, Cav-1 knock-down fibroblasts show a ∼1.4-fold increase in glucose uptake, using NBD-2-deoxy-glucose.
Thus, it appears that a loss of stromal Cav-1 is sufficient to induce mitochondrial dysfunction, with increased glucose uptake, mimicking the phenotype of cancer-associated fibroblasts in co-culture.
Discussion
Here, we have systematically monitored a number of metabolic parameters using a co-culture system that mimics tumor-stroma co-evolution.Citation9,Citation10 First, we observed that cancer cells secrete hydrogen peroxide, which induces ROS production in cancer-associated fibroblasts (summarized in ). Then, oxidative stress in cancer-associated fibroblast leads to decreases in functional mitochondrial activity, and a corresponding increase in glucose uptake, to fuel aerobic glycolysis. Conversely, we observed that MCF7 cancer cells show significant increases in mitochondrial activity, and decreases in glucose uptake. Taken together, these results suggest that fibroblasts and cancer cells in co-culture become metabolically coupled, resulting in the development of a “symbiotic” or “parasitic” relationship.Citation11 In this scenario, cancer-associated fibroblasts undergo aerobic glycolysis (producing lactate), while cancer cells use oxidative mitochondrial metabolism.Citation1 These new observations provide direct experimental support for this hypothesis. We have termed this “symbiotic” or “parasitic” model of cancer metabolism, the “reverse Warburg effect.”Citation1
We have previously shown that oxidative stress in cancer-associated fibroblasts drives a loss of stromal Cav-1, due to its destruction via autophagy/lysosomal degradation.Citation5,Citation10 Interestingly, a loss of stromal Cav-1 is sufficient to induce further oxidative stress, DNA damage and autophagy, essentially mimicking pseudo-hypoxia and driving mitochondrial dysfunction.Citation1–Citation6,Citation10 Importantly, we and others have previously shown that a loss of stromal Cav-1 is a powerful biomarker for identifying breast cancer patients with early tumor recurrence, lymph-node metastasis, drug-resistance and poor clinical outcome.Citation22–Citation27 Thus, this type of metabolism (aerobic glycolysis and autophagy in the tumor stroma) is characteristic of a lethal tumor micro-environment, as it fuels anabolic growth in cancer cells, via the production of high-energy nutrients (such as lactate, ketones and glutamine) and other chemical building blocks (fatty acids, nucleotides, amino acids).Citation4,Citation11,Citation40
Interestingly, we show here that the upstream tumor-initiating event appears to be the secretion of hydrogen peroxide by MCF7 cancer cells under co-culture conditions. This effectively shifts oxidative stress from cancer cells to adjacent stromal fibroblasts, essentially driving autophagy/mitophagy and aerobic glycolysis in tumor stroma. Interestingly, in the immune system, neutrophils and macrophages produce the most hydrogen peroxide, providing another link between how inflammation may provide “fertile ground,” by inducing DNA damage and metabolic changes in the tumor microenvironment via oxidative stress.Citation26 Consistent with this idea, tumors drive the systemic onset of DNA damage,Citation41,Citation42 likely via the secretion of hydrogen peroxide and the resulting inflammatory phenotype,Citation35 which has now been observed experimentally in pre-clinical animal models.
Should we use antioxidants for cancer prevention and therapy?
If cancer cells maintain their “fuel supply” by secreting hydrogen peroxide into the tumor stroma, this provides a novel therapeutic strategy for cancer prevention and therapy with powerful antioxidants. In this regard, one such enzymatically-active protein anti-oxidant that may be of therapeutic use is catalase, as it detoxifies hydrogen peroxide to water. In direct support of this notion, numerous studies show that “catalase therapy” in pre-clinical animal models is indeed sufficient to almost completely block tumor recurrence and metastasis.Citation43–Citation49 Here, we show that extracellular administration of catalase blocks the production of ROS in MCF7-fibroblasts co-cultures and induces apoptotic cell death in co-cultured MCF7 cancer cells, providing a new mechanism for the anti-metastatic action of catalase. Similarly, Ladiges and colleagues used transgenic expression of catalase (targeted to mitochondria) to reduce metastatic tumor burden nearly 13-fold in MMTV-PyMT mice (a mouse model of breast cancer).Citation50–Citation52
What are the possible implications of anti-oxidant therapies for breast cancer patients? Ambrosone and colleagues have shown that a particular allele of the catalase gene in humans (the CC genotype) is associated with higher catalase activity, and a near 20% reduction in breast cancer risk. This reduction in breast cancer risk was nearly doubled to 40%, if patients with the CC genotype had a high consumption of fruits and vegetables, which are rich in antioxidants.Citation53 Similarly, in a large study of nearly 5,000 women with breast cancer, if these patients used antioxidants (such as Vitamins C and E), they achieved ∼20% reductions in both recurrence and mortality.Citation54 However, this effect was only observed in patients that did not undergo radiation therapy. If breast cancer patients that underwent radiation therapy were excluded, then the reductions in both recurrence and mortality approached ∼40–50%, with anti-oxidant usage.Citation54 Thus, by eliminating oxidative stress in cancer cells and the tumor microenvironment,Citation55 we may be able to effectively cut off the tumor's fuel supply, by blocking stromal autophagy and aerobic glycolysis.Citation52,Citation55
In further support of the above clinical studies, female catalase-deficient mice spontaneously develop aggressive mammary tumors (likely due to increased hydrogen peroxide and oxidative stress) Citation56 by 9 months of age, and this can be prevented by dietary supplementation with Vitamin E, an anti-oxidant.Citation56 Similarly, male catalase-deficient mice also develop mammary tumors.Citation56 Importantly, control wild-type mice did not develop any mammary tumors in this time frame.
Consistent with the idea that excess hydrogen peroxide has potent stromal effects, catalase-deficient mice also show an increased tendency toward fibrosis and collagen deposition.Citation57–Citation60 Conversely, catalase or other antioxidants can also be administered therapeutically, in various pre-clincal models, which then prevents the onset of fibrosis.Citation61–Citation66 In this context, it is interesting to note that activated myofibroblasts are known to produce and secrete hydrogen peroxide, which can have deleterious paracrine effects on adjacent normal epithelial cells.Citation67
Furthermore, breast cancer patients show systemic evidence of increased oxidative stress and a decreased anti-oxidant defense, which increases with aging and tumor progression.Citation68–Citation70 Chemotherapy and radiation therapy then promote further oxidative stress.Citation69 Unfortunately, “sub-lethal” doses of oxidative stress during cancer therapy may contribute to tumor recurrence and metastasis, via the activation of myofibroblasts.Citation71
Explaining the onset of DNA damage in the tumor stroma.
Our current results also have implications for understanding the onset of ROS and DNA damage in the tumor microenvironment, which provides a “mutagenic motor” for cancer cell evolution. We show here that most of the ROS production eventually occurs in adjacent stromal fibroblasts. ROS production also induces DNA damage. So, it would not be unreasonable to observe extensive DNA damage in the tumor stroma. In support of these predictions, Charis Eng and colleagues have clearly documented that extensive DNA damage and genomic instability occurs in the tumor stroma, and that this stromal DNA damage phenotype is predictive of metastasis and poor clinical outcome in breast and head and neck cancer patients.Citation72–Citation74
Further independent validation comes from transcriptional profiling of the tumor stroma isolated from Cav-1(+) and Cav-1(-) breast cancer patients, via laser-capture microdissection.Citation26 In these patients, a loss of stromal Cav-1 is associated with the increased expression of gene profiles associated with normal aging, oxidative stress, DNA damage, HIF1/hypoxia, NFκB/inflammation, glycolysis and mitochondrial dysfunction.Citation26 These in vivo molecular profiling data are consistent with the metabolic observations we have made here, using the MCF7-fibroblast co-culture system, which shows the onset of ROS production, mitochondrial dysfunction and increased glucose uptake, specifically in the stromal fibroblast compartment.
Using PET scanning to detect the “reverse Warburg effect” in cancer-associated fibroblasts.
Our findings also have important implications for re-interpreting the PET scans of human tumors using Fluoro-2-deoxy-D-glucose (F-2-DG), as glucose uptake was previously thought to be solely the domain of cancer cells. Instead, here we see that cancer-associated fibroblasts show the largest increases in glucose uptake, while cancer cells show corresponding decreases in glucose uptake, under identical co-culture conditions. Thus, increased PET glucose avidity may actually be a surrogate marker for a loss of stromal Cav-1 in human tumors, allowing the rapid detection of a lethal tumor microenvironment.
Similarly, PET glucose avidity in the brain was initially attributed to glucose uptake in neurons. However, now it appears that astrocytes are actually the cell type responsible for the glucose avidity.Citation75–Citation77 In the brain, astrocytes are glycolytic and undergo aerobic glycolysis. Thus, astrocytes take up and metabolically process glucose to lactate.Citation75–Citation77 Then, lactate is secreted via a mono-carboxylate transporter, namely MCT4. As a consequence, neurons use lactate as their preferred energy substrate. This hierarchy or division of labor has been termed “neuron-glia metabolic coupling,”Citation75–Citation77 and is analogous to the “reverse Warburg effect” in human tumors. Thus, it is not far-fetched to propose that the tumor PET glucose avidity may be due to the presence of glycolytic cancer-associated fibroblasts. Interestingly, both astrocytes and cancer-associated fibroblasts express MCT4 (which extrudes lactate) and MCT4 is upregulated by oxidative stress in stromal fibroblasts.Citation34
In accordance with the idea that cancer-associated fibroblasts take up the bulk of glucose, PET glucose avidity is also now routinely used to measure the extent of fibrosis in a number of human diseases, including interstitial pulmonary fibrosis, postsurgical scars, keloids, arthritis and a variety of collagen-vascular diseases.Citation14–Citation21
Similarly, PET is also now used to detect inflammationCitation78 that is due to both infectious diseases, such as fever of unknown origin (FUO) and bacteremic foci,Citation79–Citation81 and non-infectious diseases, such as graft rejection in liver and renal transplants.Citation82–Citation85 We have recently shown that inflammatory cytokines induce autophagy in cancer-associated fibroblasts, consistent with a shift toward aerobic glycolysis under inflammatory conditions.Citation7 Interestingly, PET glucose avidity and elevated serum inflammatory markers both correlate with poor prognosis in breast cancers.Citation86–Citation90
PET glucose avidity also persists for up to 8 weeks after radiation therapy or surgical removal of the tumorCitation91 and the PET signal over-estimates the actual anatomical size of the tumor, consistent with the idea that PET glucose avidity is really measuring fibrosis and inflammation in the tumor microenvironment.
Hydrogen peroxide: environmental exposure and DNA damage.
Consistent with the idea that oxidative stress can spread systemically, hair dye use (which involves hydrogen peroxide) is associated with an ∼11-fold increased risk for DNA damage in mammary epithelial cells isolated from the milk of lactating mothers.Citation92 Similarly, wasabi (a condiment; Japanese Horseradish) contains a peroxidase enzymeCitation93 that generates high levels of hydrogen peroxide and Japanese men have the highest rates of gastric cancer in the world (∼7–10 times higher than the United States).Citation94 This possible association undoubtedly merits further study.
Hydrogen peroxide: implications for cancer diagnosis and therapy.
The idea that cancer cells produce and secrete hydrogen peroxide also has important implications for cancer imaging, diagnosis and therapy.Citation47,Citation95–Citation97 For example, new fluorescent probes have been developed to visualize xenografted tumors, based on their ability to produce hydrogen peroxide.Citation98 Similarly, human breast and lung cancer patients can be positively identified by examining their exhaled breath for the presence of hydrogen peroxide.Citation99–Citation101 In accordance with their idea that hydrogen peroxide may be produced by the tumor stroma, the exhaled breath of patients with idiopathic pulmonary fibrosis also shows increased levels of hydrogen peroxide.Citation102
Finally, tumor cell production of hydrogen peroxide drives NFκB-activation in adjacent normal cells in cultureCitation6 and during metastasis,Citation103 directly implicating the use of antioxidants, NFκB-inhibitors and anti-inflammatory agents, in the treatment of aggressive human cancers.
Materials and Methods
Cell cultures.
Human skin fibroblasts immortalized with human telomerase reverse transcriptase (hTERT-BJ1) were purchased originally from Clontech, Inc. The human breast cancer cell lines, MCF7 and MDA-MB-231, were from ATCC. All cells were maintained in DMEM, with 10% Fetal Bovine Serum (FBS) and Penicillin 100 units/mL-Streptomycin 100 µg/mL (Invitrogen). Co-culture experiments were performed as previously described with minor modifications.Citation10 Fibroblasts and cancer cells were co-plated or plated as a homotypic cell culture in 12-well plates in complete media. Fibroblasts were plated first and cancer cells were plated within 2 h. The total number of cells per well in coculture was 1 × 105 cells, with a 5:1 fibroblast-to-epithelial cell ratio. As controls, homotypic cultures of fibroblasts and cancer cells were plated in parallel, using the same number of a given cell population as the corresponding co-cultures. The day after plating, the media was changed to DMEM with 10% NuSerum I (a low protein alternative to FBS; BD Biosciences) and Pen-Strep. Cells were maintained in this media for 2 to 5 d, until further analysis. Both mono-cultures and co-cultures were maintained at 37°C in a humidified atmosphere containing 5% CO2.
Mitochondrial activity.
To measure mitochondrial activity, cells were stained with MitoTracker Orange (CMTMRos cat #M7510, Invitrogen), whose accumulation in mitochondria is dependent upon membrane potential. Briefly, GFP-MCF7 cells or GFP-MDA-MB-231 cells were co-cultured with fibroblasts for 5 d in DMEM with 10% NuSerum. After 5 d, cells were incubated with pre-warmed MitoTracker staining solution (diluted in serum free DMEM to a final concentration of 10–35 nM) for 10 min at 37°C. For MDA-MB-231 cells, the MitoTracker was used at a final concentration of 35 nM for 10 min at 37°C. All subsequent steps were performed in the dark. Cells were washed in PBS, harvested and re-suspended in 500 µL of Binding Buffer (BD Biosciences). Cells were then analyzed by flow cytometry using a GFP signal detector with excitation wavelength of 488 nm and emission of 530 nm (to detect GFP-positive MCF7 or MDA-MB-231 cells), and a PE Texas Red signal detector with excitation wavelength of 496 nm and emission of 615 nm (to detect MitoTracker). Thus, fibroblasts in co-culture were the GFP-negative population. Data analysis was performed using FlowJo 8.8 software.
Glucose uptake.
The glucose uptake assay was performed using 2-NBDG {2-[N-(7-nitrobenz-2-oxa-1,3-diazol-4-yl) amino]-2-deoxy-D-glucose, cat #N13195, Invitrogen}. MCF7-RFP cells were co-cultured with fibroblasts for 5 d in DMEM with 10% NuSerum I. After 5 d, cells were incubated with pre-warmed 2-NBDG solution (diluted in serum free DMEM to a final concentration of 200 µM) for 15 min at 37°C. All subsequent steps were performed in the dark. Cells were washed in PBS, harvested and re-suspended in 500 µL of Binding Buffer (BD Biosciences). Cells were then analyzed by flow cytometry using a GFP signal detector with excitation wavelength of 488 nm and emission of 530 nm (to detect 2-NBDG), and a PE Texas Red signal detector with excitation wavelength of 496 nm and emission of 615 nm (to detect RFP-positive MCF7 cells). Thus, fibroblasts in co-culture were the RFP-negative population. Data analysis was performed using FlowJo 8.8 software.
ROS measurement.
CM-H2DCFDA [5-(and 6-) carboxy-2′,7′-dichlorodihydrofluorescein diacetate, cat #C369, Invitrogen] is a cell-permeable molecule used to measure intracellular ROS. CM-H2DCFDA is a non-fluorescent molecule (DCFH) that, when taken up by cells, is rapidly oxidized to a highly fluorescent derivative (DCF) that is a measure of intracellular oxidant production. The CM-H2DCFDA fluorescence method was used according to the manufacturer's instructions. Briefly, MCF7-RFP cells were co-cultured with fibroblasts for 5 d in DMEM with 10% NuSerum I. After 5 d, cells were washed twice with HBSS buffer, incubated with 10 µM CM-H2DCFDA for 15 min at 37°C. All subsequent steps were performed in the dark. Cells were washed and incubated with Phenol-red free media supplemented with 10% FBS for 15 min at 37°C. Cells were then washed in PBS, harvested and re-suspended in 500 µL of Binding Buffer (BD Biosciences). Cells were then analyzed by flow cytometry using a GFP signal detector with excitation wavelength of 488 nm and emission of 530 nm (to detect DCF-DA), and a PE Texas Red signal detector with excitation wavelength of 496 nm and emission of 615 and (to detect RFP-positive MCF7 cells). Thus, fibroblasts in co-culture were the RFP-negative population. Data analysis was performed using FlowJo 8.8 software.
Catalase treatment.
Co-cultures and corresponding homotypic cultures were established. The next day, the media was changed to DMEM with 10% NuSerum I, supplemented with bovine catalase (cat # C1345; 2 µM dissolved in media, Sigma; powder, suitable for cell culture, 2,000–5,000 units/mg protein). After 48 h, the cells were subjected to either ROS staining or apoptosis analysis. As control, cells were incubated in parallel with BSA-fraction-V (2 mM, Invitrogen) or left untreated.
Apoptosis assay.
Cell death was quantified by flow cytometry using propidium iodide (PI) and Annexin-V-APC (Apoptosis Detection Kit, BD Biosciences), as we previously described with minor modifications.Citation5,Citation6 Briefly, MCF7-GFP cells were co-cultured with fibroblasts in DMEM with 10% NuSerum I. After 2 d, cells were collected by centrifugation and re-suspended in 500 µL of Annexin-V Binding Buffer. Then, the annexin V-APC conjugate (BD Biosciences 550474) (4 µL) and PI (1 µL) was added and incubated in the dark at room temperature for 5 min. Cells were then analyzed by flow cytometry using a GFP signal detector with excitation wavelength of 488 nm and emission of 530 nm (to detect MCF7-GFP cells), a PE Texas Red signal detector with excitation wavelength of 496 nm and emission of 615 (to detect PI) and an APC signal detector with excitation wavelength of 650 nm and emission of 660 nm. Apoptosis analysis was performed using FlowJo 8.8 software.
Statistical analysis.
Data were analyzed using the Student's t-test. p values lower than 0.05 were considered statistically significant.
Immunocytochemistry.
Cells were fixed after 5 d of culture and immunostained as we have previously described, with minor modifications.Citation34 Briefly, cells were fixed for 30 min at room temperature in 2% paraformaldehyde diluted in PBS, after which they were permeabilized with cold methanol at −20°C for 5 min. All subsequent steps were performed at room temperature. After rinsing with PBS with 0.1 mM Calcium chloride and 1 mM Magnesium chloride (PBS/CM), cells were incubated with NH4Cl in PBS to quench free aldehyde groups. Cells were then blocked with immunofluorescence (IF) buffer (PBS, 1% BSA, 0.1% Tween 20) for 1 h. Anti-GLUT1 antibodies (AB1340, Chemicon) were incubated in IF buffer for 1 h. After washing with IF buffer (3x, 10 min each), cells were incubated for 30 min with fluorochrome-conjugated secondary antibodies diluted in IF buffer. Finally, slides were washed with IF buffer (3x, 10 min each), rinsed with PBS/CM, counter-stained with DAPI (10 µg/ml) in PBS and mounted with Prolong Gold anti-fade Reagent (Invitrogen).
Analysis of Cav-1 knock-down fibroblasts.
GFP-positive hTERT-BJ1 Cav-1 knock-down (KD) fibroblasts (and vector controls) were as we previously described in reference Citation39, or were generated using siRNA vectors (Santa Cruz Biotech; sc29241-V; sc-44202-V and sc-108080). The latter hTERT-BJ1 fibroblasts are GFP-negative. For the mitochondrial activity studies, GFP-positive Cav-1 KD fibroblasts and control fibroblasts were seeded in DMEM-10% FBS. The next day, the media was changed to DMEM-10% NuSerum I. After 5 d, cells were stained with 35 nM MitoTracker and analyzed by flow cytometry. For the glucose uptake studies, GFP-negative Cav-1 KD fibroblasts and control fibroblasts were seeded in DMEM-10% FBS. The next day, the media was changed to DMEM-10% NuSerum I. After 48 h, the cells were subjected to the glucose uptake assay with 200 µM 2-NBDG and analyzed by flow cytometry.
Figures and Tables
Figure 1 Overall strategy for quantitating cancer metabolism in MCF7-fibroblast co-cultures. MCF7 cells are co-cultured with stromal fibroblasts for 5 d. Then, the co-cultures are briefly incubated with different fluorescent probes to measure mitochondrial activity (Mito-Tracker), glucose uptake (NBD-2-deoxy-glucose) or ROS production (DCF-DA). Afterwards, co-cultures are mildy-trypsinized and subjected to FACS analysis to physically separate MCF7 cancer cells from stromal fibroblasts which is analogous to laser-capture micro-dissection (LCM). This strategy allows for compartment-specific metabolic analysis. For all of our experiments, we used MCF7 cells recombinantly tagged with either GFP (green fluorescent protein) or RFP (red fluorescent protein) and unlabelled stromal fibroblasts.
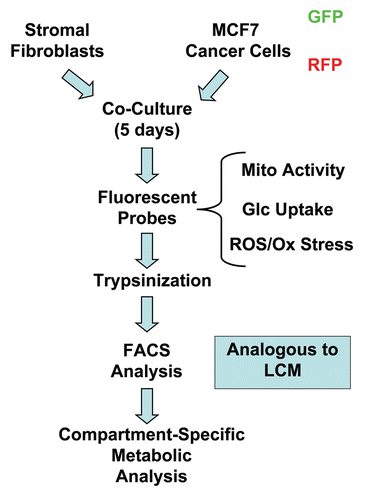
Figure 2 Changes in mitochondrial activity in MCF7 cells and fibroblasts in co-culture. FACS tracings for mitochondrial activity (MitoTracker red fluorescence) in fibroblasts or cancer cells, either cultured alone or in combination, are shown. Interestingly, during co-culture, MCF7 cells show a shift to the right, as indicated by the blue arrow. Conversely, during co-culture, stromal fibroblasts show just the opposite effect, with a shift to the left.
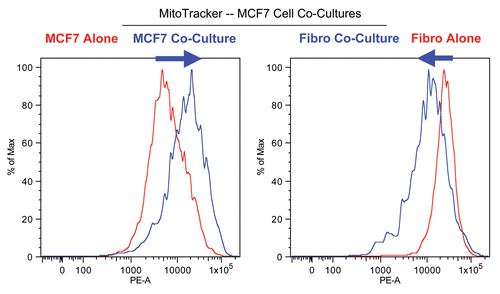
Figure 3 Increased mitochondrial activity in MCF7 cells in co-culture: quantitative analysis. After 5 d of co-culture, MCF7 cells show a >2-fold increase in mitochondrial activity (A). Similar results were obtained using a range (10–35 nM) of Mitotracker concentrations (B).
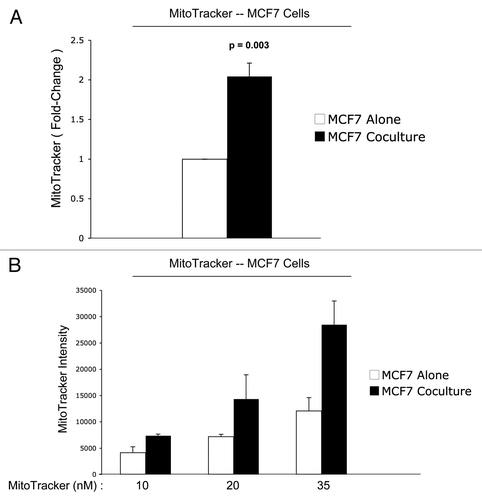
Figure 4 Reduced mitochondrial activity in fibroblasts in co-culture: quantitative analysis. After 5 d of co-culture, stromal fibroblasts show a 1.7-fold reduction in mitochondrial activity (A). Similar results were obtained using a range (10–35 nM) of MitoTracker concentrations (B).
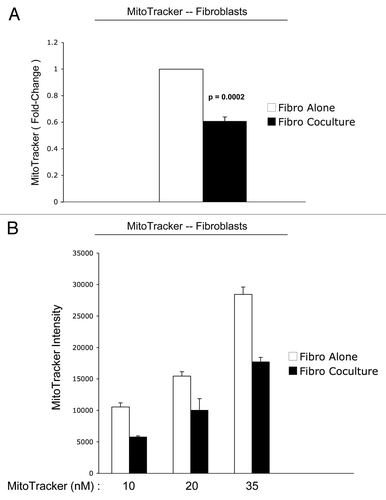
Figure 5 Mitochondrial activity in MDA-MB-231 cells and fibroblasts in co-culture. FACS tracings for mitochondrial activity (MitoTracker red fluorescence) in fibroblasts or MDA-MB-231 cancer cells, either cultured alone or in combination, are shown (A). The direction of change in co-culture is indicated by blue arrows. Interestingly, after 5 d of co-culture, MDA-MB-231 cells show a shift to the right, which corresponds to a 1.5-fold increase in mitochondrial activity (A and B). Conversely, after 5 d of co-culture, stromal fibroblasts show just the opposite effect, with a shift to the left, resulting in a >5-fold reduction in mitochondrial activity (A and C).
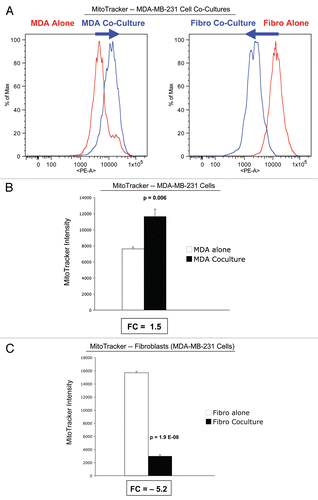
Figure 6 Glucose uptake in MCF7 cells and fibroblasts in co-culture. FACS tracings for glucose uptake (NBD-2-deoxy-glucose) in fibroblasts or MCF7 cancer cells, either cultured alone or in combination, are shown (A). The direction of change in co-culture is indicated by blue arrows. Note that after 5 d of co-culture, stromal fibroblasts show a shift to the right, which corresponds to a 1.8-fold increase in glucose uptake (A and B). Remarkably, after 5 d of co-culture, MCF7 cancer cells show just the opposite phenotype, with a shift to the left, resulting in a 1.6-fold reduction in glucose uptake (A and B).
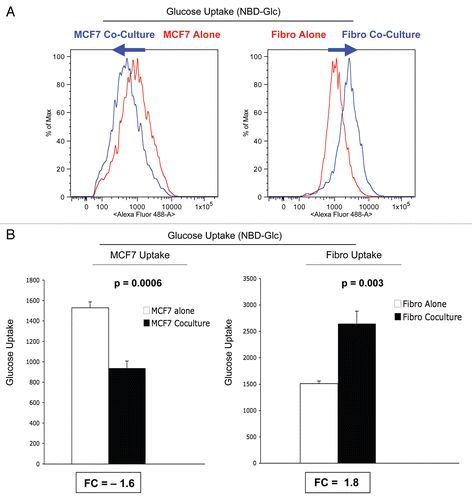
Figure 7 ROS production in MCF7 cells and fibroblasts at 5-days of co-culture. MCF7 cancer cells and stromal fibroblasts were co-cultured for 5 d, and then subjected to FACS analysis, with the fluorescent probe DCF-DA, which measures ROS production. FACS tracings for ROS production are shown in (A). At day 5 of co-culture, stromal fibroblasts show a shift to the right, which corresponds to a >two-fold increase in ROS production (B). In contrast, cancer cells show only a 1.2-fold increase in ROS production, under the same conditions (B). Thus, after 5 d of co-culture, stromal fibroblasts show increases in ROS production and glucose uptake, and a corresponding decrease in mitochondrial activity.
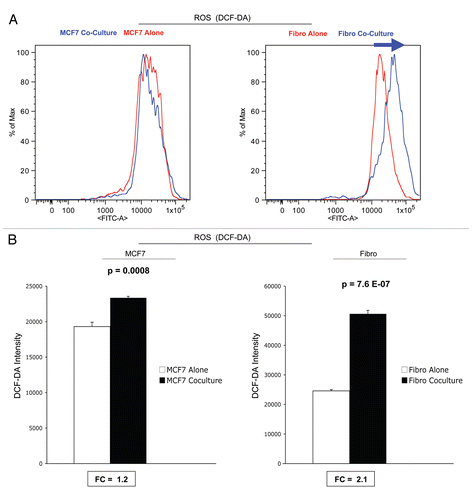
Figure 8 ROS production in MCF7 cells and fibroblasts at 2-days of co-culture: effects of catalase. At 2 d of co-culture, MCF7 cancer cells show a near three-fold induction of ROS production (A), while stromal fibroblasts have only minor increases in ROS production (B). Note that addition of extracellular catalase (an enzyme that detoxifies hydrogen peroxide to water) was sufficient to normalize ROS associated with co-cultured cancer cells (A). Under these conditions, an irrelevant protein (namely BSA) had no effect (A). Thus, it appear that ROS production starts in cancer cells, via the generation and secretion of hydrogen peroxide, and then spreads to adjacent stromal fibroblasts.
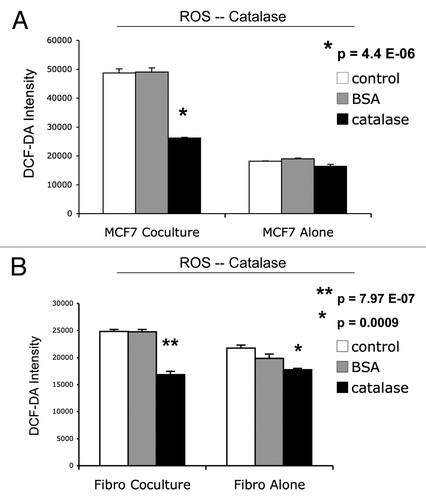
Figure 9 Catalase induces apoptosis in MCF7 cells in co-culture. Next, we added catalase to MCF7-fibroblas co-culture to detoxify hydrogen peroxide and prevent oxidative stress. Note that the simple addition of extracellular catalase to the culture media induced a five-fold increase in apoptotic cell death in co-cultured cancer cells (A); however, an irrelevant protein (namely BSA) had no effect (B). Catalase may induce apoptosis in co-cultured cancer cells, by detoxifying extracellular hydrogen peroxide, thereby cutting off the fuel supply for cancer cells. Cell death was defined as the Annexin V(+)/PI(−) population of cells (“early apoptosis”).
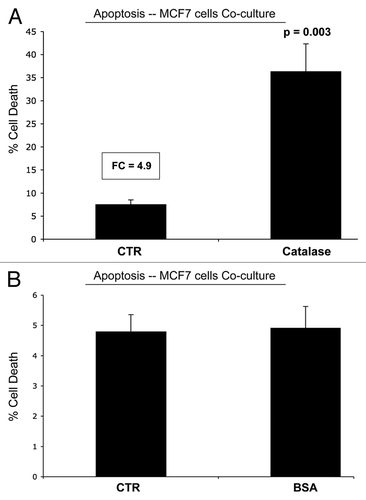
Figure 10 GLUT1 expression in MCF7 cells is downregulated by co-culture with stromal fibroblasts. MCF7 cell-fibroblast co-cultures were immuno-stained with a specific antibody probe directed against GLUT1. MCF7 cells cultured alone were processed in parallel, under identical culture conditions. Note that GLUT1 expression is dramatically downregulated when MCF7 cells are co-cultured with fibroblasts, consistent with our functional results obtained by FACS analysis. MCF7 cells were visualized by immuno-staining with keratin-8/18 antibodies. Nuclei are counter-stained with DAPI and appear blue.
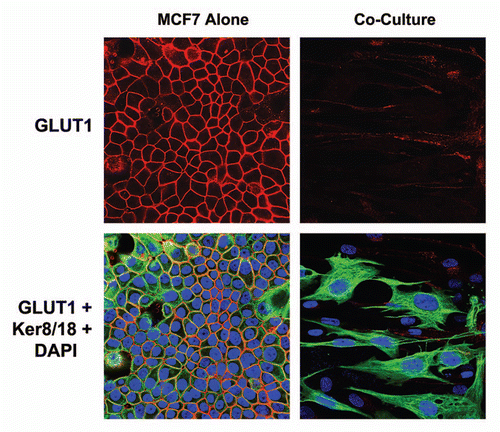
Figure 11 GLUT1 expression in MCF7 cells is downregulated by high energy metabolites. MCF7 cells alone were treated with L-Lactate (10 mM) and 3-Hydroxy-Butyrate (10 mM) for 2 d or left untreated. Note that treatment with either L-Lactate and 3-Hydroxy-Butyrate is sufficient to phenocopy the effects of fibroblasts on GLUT1 expression in co-cultured MCF7 cells, resulting in a loss of GLUT1 expression.
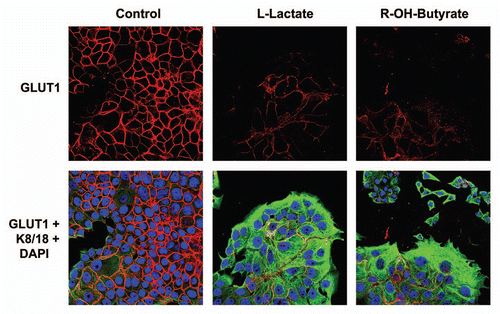
Figure 12 A loss of Cav-1 drives mitochondrial dysfunction and increased glucose uptake in stromal fibroblasts. (A) Western blot analysis shows the targeted stable knock-down of Cav-1 in human stromal fibroblasts, using an siRNA-based approach. Immuno-blotting with β-actin is shown as a control for equal loading. (B) Cav-1 knock-down fibroblasts show a ∼1.8 fold reduction in mitochondrial activity, as measured with the MitoTracker probe, relative to control vector fibroblasts. (C) Cav-1 knock-down fibroblasts show a ∼1.4-fold increase in glucose uptake, using NBD-2-deoxy-glucose, relative to control vector fibroblasts.
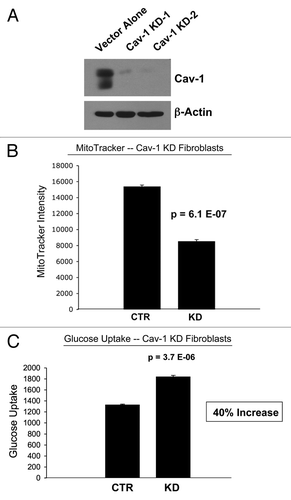
Figure 13 Hydrogen peroxide and ROS production in the tumor micro-environment fuels the anabolic growth of cancer cells. Recently, we proposed a new mechanism for understanding the Warburg effect in tumor metabolism. In this new paradigm, cancer cells induce oxidative stress in neighboring cancer-associated fibroblasts. Then, oxidative stress, in cancer-associated fibroblasts, triggers the activation of two main transcription factors, NFκB and HIF-1α, leading to the onset of inflammation, autophagy, mitophagy and aerobic glycolysis in the tumor micro-environment. this type of stromal metabolism then produces high-energy nutrients (lactate, ketones and glutamine), as well as recycled chemical building blocks (nucleotides, amino acids, fatty acids), to literally “feed” cancer cells. these nutrients (such as lactate) stimulate mitochondrial biogenesis in adjacent cancer cells. In addition, the oxidative stress and ROS, produced in cancer-associated fibroblasts, has a “bystander effect” on adjacent cancer cells, leading to DNA damage, genomic instability and aneuploidy, driving tumor-stroma co-evolution. We have previously termed this new paradigm the “reverse Warburg effect,” as we see that aerobic glycolysis takes place in cancer-associated fibroblasts, rather than in tumor cells, as previously suspected. Here, we showed that tumor cells produce and secrete hydrogen peroxide, thereby “fertilizing” the tumor microenvironment and driving the “reverse Warburg effect.” As a consequence, oxidative stress initiated in tumor cells is transferred to cancer-associated fibroblasts, via hydrogen peroxide. Modified and reproduced with permission from reference Citation6.
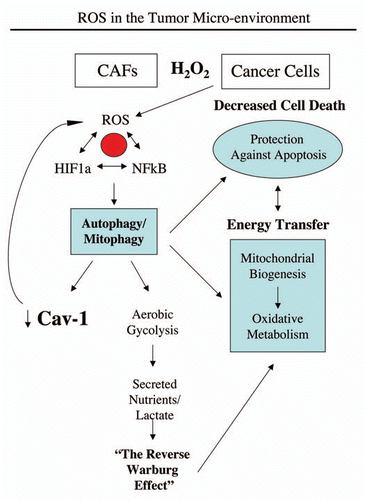
Table 1 Relative mitochondrial activity, glucose uptake and ROS production in single cell cultures vs. co-cultures
Acknowledgments
M.P.L. and his laboratory were supported by grants from the NIH/NCI (R01-CA-080250; R01-CA-098779; R01-CA-120876; R01-AR-055660), and the Susan G. Komen Breast Cancer Foundation. F.S. was supported by grants from the W.W. Smith Charitable Trust, the Breast Cancer Alliance (BCA), and a Research Scholar Grant from the American Cancer Society (ACS). Funds were also contributed by the Margaret Q. Landenberger Research Foundation (to M.P.L.). R.G.P. was supported by grants from the NIH/NCI (R01-CA-70896, R01-CA-75503, R01-CA-86072 and R01-CA-107382) and the Dr. Ralph and Marian C. Falk Medical Research Trust. The Kimmel Cancer Center was supported by the NIH/NCI Cancer Center Core grant P30-CA-56036 (to R.G.P.).
This project is funded, in part, under a grant with the Pennsylvania Department of Health (to M.P.L. and F.S.). The Department specifically disclaims responsibility for any analyses, interpretations or conclusions. This work was also supported, in part, by a Centre grant in Manchester from Breakthrough Breast Cancer in the UK (to A.H.) and an Advanced ERC Grant from the European Research Council.
References
- Pavlides S, Whitaker-Menezes D, Castello-Cros R, Flomenberg N, Witkiewicz AK, Frank PG, et al. The reverse Warburg effect: Aerobic glycolysis in cancer associated fibroblasts and the tumor stroma. Cell Cycle 2009; 8:3984 - 4001; PMID: 19923890; http://dx.doi.org/10.4161/cc.8.23.10238
- Pavlides S, Tsirigos A, Vera I, Flomenberg N, Frank PG, Casimiro MC, et al. Loss of stromal caveolin-1 leads to oxidative stress, mimics hypoxia and drives inflammation in the tumor microenvironment, conferring the “reverse Warburg effect”: A transcriptional informatics analysis with validation. Cell Cycle 2010; 9:2201 - 2219; PMID: 20519932; http://dx.doi.org/10.4161/cc.9.11.11848
- Pavlides S, Tsirigos A, Vera I, Flomenberg N, Frank PG, Casimiro MC, et al. Transcriptional evidence for the “Reverse Warburg Effect” in human breast cancer tumor stroma and metastasis: similarities with oxidative stress, inflammation, Alzheimer's disease and “Neuron-Glia Metabolic Coupling”. Aging (Albany NY) 2010; 2:185 - 199; PMID: 20442453
- Pavlides S, Tsirigos A, Migneco G, Whitaker-Menezes D, Chiavarina B, Flomenberg N, et al. The autophagic tumor stroma model of cancer: Role of oxidative stress and ketone production in fueling tumor cell metabolism. Cell Cycle 2010; 9:3485 - 505; PMID: 20861672; http://dx.doi.org/10.4161/cc.9.17.12721
- Martinez-Outschoorn UE, Balliet RM, Rivadeneira DB, Chiavarina B, Pavlides S, Wang C, et al. Oxidative stress in cancer associated fibroblasts drives tumor-stroma co-evolution: A new paradigm for understanding tumor metabolism, the field effect and genomic instability in cancer cells. Cell Cycle 2010; 9:3256 - 3276; PMID: 20814239; http://dx.doi.org/10.4161/cc.9.16.12553
- Martinez-Outschoorn UE, Trimmer C, Lin Z, Whitaker-Menezes D, Chiavarina B, Zhou J, et al. Autophagy in cancer associated fibroblasts promotes tumor cell survival: Role of hypoxia, HIF1 induction and NFkappaB activation in the tumor stromal microenvironment. Cell Cycle 2010; 9:3515 - 3533; PMID: 20855962; http://dx.doi.org/10.4161/cc.9.17.12928
- Martinez-Outschoorn UE, Whitaker-Menezes D, Lin Z, Flomenberg N, Howell A, Pestell RG, et al. Cytokine production and inflammation drive autophagy in the tumor microenvironment: Role of stromal caveolin-1 as a key regulator. Cell Cycle 2011; 10:1784 - 1793; PMID: 21566463
- Martinez-Outschoorn UE, Prisco M, Ertel A, Tsirigos A, Lin Z, Pavlides S, et al. Ketones and lactate increase cancer cell “stemness,” driving recurrence, metastasis and poor clinical outcome in breast cancer: Achieving personalized medicine via Metabolo-Genomics. Cell Cycle 2011; 10:1271 - 1286; PMID: 21512313; http://dx.doi.org/10.4161/cc.10.8.15330
- Lisanti MP, Martinez-Outschoorn UE, Chiavarina B, Pavlides S, Whitaker-Menezes D, Tsirigos A, et al. Understanding the “lethal” drivers of tumor-stroma co-evolution: emerging role(s) for hypoxia, oxidative stress and autophagy/mitophagy in the tumor micro-environment. Cancer Biol Ther 2010; 10:537 - 542; PMID: 20861671; http://dx.doi.org/10.4161/cbt.10.6.13370
- Martinez-Outschoorn UE, Pavlides S, Whitaker-Menezes D, Daumer KM, Milliman JN, Chiavarina B, et al. Tumor cells induce the cancer associated fibroblast phenotype via caveolin-1 degradation: Implications for breast cancer and DCIS therapy with autophagy inhibitors. Cell Cycle 2010; 9:2423 - 2433; PMID: 20562526; http://dx.doi.org/10.4161/cc.9.12.12048
- Martinez-Outschoorn UE, Pavlides S, Howell A, Pestell RG, Tanowitz HB, Sotgia F, Lisanti MP. Stromal-epithelial metabolic coupling in cancer: Integrating autophagy and metabolism in the tumor microenvironment. Int J Biochem Cell Biol 2011; 43:1045 - 1051
- Gargini R, Garcia-Escudero V, Izquierdo M. Therapy mediated by mitophagy abrogates tumor progression. Autophagy 2011; 7:466 - 476; PMID: 21270513; http://dx.doi.org/10.4161/auto.7.5.14731
- Niethammer P, Grabher C, Look AT, Mitchison TJ. A tissue-scale gradient of hydrogen peroxide mediates rapid wound detection in zebrafish. Nature 2009; 459:996 - 999; PMID: 19494811; http://dx.doi.org/10.1038/nature08119
- Cheng YM, Ho CL, Chiu NT, Hsu KF. Cesarean section scar mimicking uterine malignant neoplasm at positron emission tomography/computed tomography. J Minim Invasive Gynecol 2009; 16:372 - 374; PMID: 19423072; http://dx.doi.org/10.1016/j.jmig.2009.02.005
- Gorenberg M, Bar-Shalom R, Israel O. Patterns of FDG uptake in post-thoracotomy surgical scars in patients with lung cancer. Br J Radiol 2008; 81:821 - 825; PMID: 18796557; http://dx.doi.org/10.1259/bjr/26210052
- Ozawa T, Okamura T, Harada T, Muraoka M, Ozawa N, Koyama K, et al. Accumulation of glucose in keloids with FDG-PET. Ann Nucl Med 2006; 20:41 - 44; PMID: 16485573; http://dx.doi.org/10.1007/BF02985589
- Vaglio A, Greco P, Versari A, Filice A, Cobelli R, Manenti L, et al. Post-treatment residual tissue in idiopathic retroperitoneal fibrosis: active residual disease or silent “scar”? A study using 18F-fluorodeoxyglucose positron emission tomography. Clin Exp Rheumatol 2005; 23:231 - 234; PMID: 15895895
- Hofer C, Laubenbacher C, Block T, Breul J, Hartung R, Schwaiger M. Fluorine-18-fluorodeoxyglucose positron emission tomography is useless for the detection of local recurrence after radical prostatectomy. Eur Urol 1999; 36:31 - 35; PMID: 10364652; http://dx.doi.org/10.1159/000019923
- Groves AM, Win T, Screaton NJ, Berovic M, Endozo R, Booth H, et al. Idiopathic pulmonary fibrosis and diffuse parenchymal lung disease: implications from initial experience with 18F-FDG PET/CT. J Nucl Med 2009; 50:538 - 545; PMID: 19289428; http://dx.doi.org/10.2967/jnumed.108.057901
- Nishiyama Y, Yamamoto Y, Dobashi H, Kameda T. Clinical value of 18F-fluorodeoxyglucose positron emission tomography in patients with connective tissue disease. Jpn J Radiol 2010; 28:405 - 413; PMID: 20661690; http://dx.doi.org/10.1007/s11604-010-0445-x
- Okabe T, Shibata H, Shizukuishi K, Yoneyama T, Inoue T, Tateishi U. F-18 FDG Uptake Patterns and Disease Activity of Collagen Vascular Diseases-Associated Arthritis. Clin Nucl Med 2011; 36:350 - 354; PMID: 21467850; http://dx.doi.org/10.1097/RLU.0b013e318212c858
- Witkiewicz AK, Casimiro MC, Dasgupta A, Mercier I, Wang C, Bonuccelli G, et al. Towards a new “stromal-based” classification system for human breast cancer prognosis and therapy. Cell Cycle 2009; 8:1654 - 1658; PMID: 19448435; http://dx.doi.org/10.4161/cc.8.11.8544
- Witkiewicz AK, Dasgupta A, Nguyen KH, Liu C, Kovatich AJ, Schwartz GF, et al. Stromal caveolin-1 levels predict early DCIS progression to invasive breast cancer. Cancer Biol Ther 2009; 8:1071 - 1079; PMID: 19502809; http://dx.doi.org/10.4161/cbt.8.11.8874
- Witkiewicz AK, Dasgupta A, Sammons S, Er O, Potoczek MB, Guiles F, et al. Loss of stromal caveolin-1 expression predicts poor clinical outcome in triple negative and basal-like breast cancers. Cancer Biol Ther 2010; 10:135 - 143; PMID: 20431349; http://dx.doi.org/10.4161/cbt.10.2.11983
- Witkiewicz AK, Dasgupta A, Sotgia F, Mercier I, Pestell RG, Sabel M, et al. An absence of stromal caveolin-1 expression predicts early tumor recurrence and poor clinical outcome in human breast cancers. Am J Pathol 2009; 174:2023 - 2034; PMID: 19411448; http://dx.doi.org/10.2353/ajpath.2009.080873
- Witkiewicz AK, Kline J, Queenan M, Brody JR, Tsirigos A, Bilal E, et al. Molecular profiling of a lethal tumor microenvironment, as defined by stromal caveolin-1 status in breast cancers. Cell Cycle 2011; 10:1794 - 1809; PMID: 21521946
- Sloan EK, Ciocca DR, Pouliot N, Natoli A, Restall C, Henderson MA, et al. Stromal cell expression of caveolin-1 predicts outcome in breast cancer. Am J Pathol 2009; 174:2035 - 2043; PMID: 19411449; http://dx.doi.org/10.2353/ajpath.2009.080924
- Koo JS, Park S, Kim SI, Lee S, Park BW. The impact of caveolin protein expression in tumor stroma on prognosis of breast cancer. Tumour Biol 2011; 32:787 - 799; PMID: 21584795; http://dx.doi.org/10.1007/s13277-011-0181-6
- Qian N, Ueno T, Kawaguchi-Sakita N, Kawashima M, Yoshida N, Mikami Y, et al. Prognostic significance of tumor/stromal caveolin-1 expression in breast cancer patients. Cancer Sci 2011; 102:1590 - 1596; PMID: 21585620; http://dx.doi.org/10.1111/j.1349-7006.2011.01985.x
- Ghajar CM, Meier R, Bissell MJ. Quis custodiet ipsos custodies: who watches the watchmen?. Am J Pathol 2009; 174:1996 - 1999; PMID: 19465642; http://dx.doi.org/10.2353/ajpath.2009.090363
- Volonte D, Galbiati F. Caveolin-1, cellular senescence and pulmonary emphysema. Aging (Albany NY) 2009; 1:831 - 835; PMID: 20157570
- Sáinz-Jaspeado M, Martin-Liberal J, Lagares-Tena L, Mateo-Lozano S, Garcia De, Muro X, Tirado OM. Caveolin-1 in sarcomas: friend or foe?. Oncotarget 2011; 2:305 - 312; PMID: 21471610
- Darnell JE Jr. STAT3, HIF-1, glucose addiction and Warburg effect. Aging (Albany NY) 2010; 2:890 - 891; PMID: 21149895
- Whitaker-Menezes D, Martinez-Outschoorn UE, Lin Z, Ertel A, Flomenberg N, Witkiewicz AK, et al. Evidence for a stromal-epithelial “lactate shuttle” in human tumors: MCT4 is a marker of oxidative stress in cancer-associated fibroblasts. Cell Cycle 2011; 10:1772 - 1783; PMID: 21558814
- Szatrowski TP, Nathan CF. Production of large amounts of hydrogen peroxide by human tumor cells. Cancer Res 1991; 51:794 - 798; PMID: 1846317
- Burdon RH. Superoxide and hydrogen peroxide in relation to mammalian cell proliferation. Free Radic Biol Med 1995; 18:775 - 794; PMID: 7750801; http://dx.doi.org/10.1016/0891-5849(94)00198-S
- Feng Y, Santoriello C, Mione M, Hurlstone A, Martin P. Live imaging of innate immune cell sensing of transformed cells in zebrafish larvae: parallels between tumor initiation and wound inflammation. PLoS Biol 2010; 8:1000562; PMID: 21179501; http://dx.doi.org/10.1371/journal.pbio.1000562
- Ubezio P, Civoli F. Flow cytometric detection of hydrogen peroxide production induced by doxorubicin in cancer cells. Free Radic Biol Med 1994; 16:509 - 516; PMID: 8005536; http://dx.doi.org/10.1016/0891-5849(94)90129-5
- Trimmer C, Sotgia F, Whitaker-Menezes D, Balliet RM, Eaton G, Martinez-Outschoorn UE, et al. Caveolin-1 and mitochondrial SOD2 (MnSOD) function as tumor suppressors in the stromal microenvironment: a new genetically tractable model for human cancer associated fibroblasts. Cancer Biol Ther 2011; 11:383 - 394; PMID: 21150282; http://dx.doi.org/10.4161/cbt.11.4.14101
- Martinez-Outschoorn UE, Whitaker-Menezes D, Pavlides S, Chiavarina B, Bonuccelli G, Casey T, et al. The Autophagic Tumor Stroma Model of Cancer or “Battery-Operated Tumor Growth”: A Simple Solution to the Autophagy Paradox. Cell Cycle 2010; 9:4297 - 4306; PMID: 21051947; http://dx.doi.org/10.4161/cc.9.21.13817
- Martin OA, Redon CE, Dickey JS, Nakamura AJ, Bonner WM. Para-inflammation mediates systemic DNA damage in response to tumor growth. Commun Integr Biol 2011; 4:78 - 81; PMID: 21509186
- Redon CE, Dickey JS, Nakamura AJ, Kareva IG, Naf D, Nowsheen S, et al. Tumors induce complex DNA damage in distant proliferative tissues in vivo. Proc Natl Acad Sci USA 2010; 107:17992 - 17997; PMID: 20855610; http://dx.doi.org/10.1073/pnas.1008260107
- Hyoudou K, Nishikawa M, Ikemura M, Kobayashi Y, Mendelsohn A, Miyazaki N, et al. Prevention of pulmonary metastasis from subcutaneous tumors by binary system-based sustained delivery of catalase. J Control Release 2009; 137:110 - 115; PMID: 19361547; http://dx.doi.org/10.1016/j.jconrel.2009.04.005
- Hyoudou K, Nishikawa M, Kobayashi Y, Umeyama Y, Yamashita F, Hashida M. PEGylated catalase prevents metastatic tumor growth aggravated by tumor removal. Free Radic Biol Med 2006; 41:1449 - 1458; PMID: 17023272; http://dx.doi.org/10.1016/j.freeradbiomed.2006.08.004
- Nishikawa M. Reactive oxygen species in tumor metastasis. Cancer Lett 2008; 266:53 - 59; PMID: 18362051; http://dx.doi.org/10.1016/j.canlet.2008.02.031
- Nishikawa M, Hashida M. Inhibition of tumour metastasis by targeted delivery of antioxidant enzymes. Expert Opin Drug Deliv 2006; 3:355 - 369; PMID: 16640496; http://dx.doi.org/10.1517/17425247.3.3.355
- Nishikawa M, Hashida M, Takakura Y. Catalase delivery for inhibiting ROS-mediated tissue injury and tumor metastasis. Adv Drug Deliv Rev 2009; 61:319 - 326; PMID: 19385054; http://dx.doi.org/10.1016/j.addr.2009.01.001
- Nishikawa M, Tamada A, Hyoudou K, Umeyama Y, Takahashi Y, Kobayashi Y, et al. Inhibition of experimental hepatic metastasis by targeted delivery of catalase in mice. Clin Exp Metastasis 2004; 21:213 - 221; PMID: 15387371; http://dx.doi.org/10.1023/B:CLIN.0000037706.13747.5e
- Nishikawa M, Tamada A, Kumai H, Yamashita F, Hashida M. Inhibition of experimental pulmonary metastasis by controlling biodistribution of catalase in mice. Int J Cancer 2002; 99:474 - 479; PMID: 11992420; http://dx.doi.org/10.1002/ijc.10387
- Goh J, Enns L, Fatemie S, Hopkins H, Morton J, Pettan-Brewer C, et al. Mitochondrial targeted catalase suppresses invasive breast cancer in mice. BMC Cancer 2011; 11:191; PMID: 21605372; http://dx.doi.org/10.1186/1471-2407-11-191
- Schriner SE, Linford NJ, Martin GM, Treuting P, Ogburn CE, Emond M, et al. Extension of murine life span by overexpression of catalase targeted to mitochondria. Science 2005; 308:1909 - 1911; PMID: 15879174; http://dx.doi.org/10.1126/science.1106653
- Sotgia F, Martinez-Outschoorn UE, Lisanti MP. Mitochondrial Oxidative Stress Drives Tumor Progression and Metastasis: Should We Use Antioxidants As A Key Component of Cancer Treatment and Prevention?. BMC Med 2011; 9:62; PMID: 21605374; http://dx.doi.org/10.1186/1741-7015-9-62
- Ahn J, Gammon MD, Santella RM, Gaudet MM, Britton JA, Teitelbaum SL, et al. Associations between breast cancer risk and the catalase genotype, fruit and vegetable consumption and supplement use. Am J Epidemiol 2005; 162:943 - 952; PMID: 16192345; http://dx.doi.org/10.1093/aje/kwi306
- Nechuta S, Lu W, Chen Z, Zheng Y, Gu K, Cai H, et al. Vitamin supplement use during breast cancer treatment and survival: a prospective cohort study. Cancer Epidemiol Biomarkers Prev 2011; 20:262 - 271; PMID: 21177425; http://dx.doi.org/10.1158/1055-9965.EPI-10-1072
- Trimmer C, Sotgia F, Whitaker-Menezes D, Balliet R, Eaton G, Martinez-Outschoorn UE, et al. Caveolin-1 and mitochondrial SOD2 (MnSOD) function as tumor suppressors in the stromal microenvironment: A new genetically tractable model for human cancer associated fibroblasts. Cancer Biol Ther 2011; 11:383 - 394; PMID: 21150282; http://dx.doi.org/10.4161/cbt.11.4.14101
- Ishii K, Zhen LX, Wang DH, Funamori Y, Ogawa K, Taketa K. Prevention of mammary tumorigenesis in acatalasemic mice by vitamin E supplementation. Jpn J Cancer Res 1996; 87:680 - 684; PMID: 8698615
- Kobayashi M, Sugiyama H, Wang DH, Toda N, Maeshima Y, Yamasaki Y, et al. Catalase deficiency renders remnant kidneys more susceptible to oxidant tissue injury and renal fibrosis in mice. Kidney Int 2005; 68:1018 - 1031; PMID: 16105032; http://dx.doi.org/10.1111/j.1523-755.2005.00494.x
- Sunami R, Sugiyama H, Wang DH, Kobayashi M, Maeshima Y, Yamasaki Y, et al. Acatalasemia sensitizes renal tubular epithelial cells to apoptosis and exacerbates renal fibrosis after unilateral ureteral obstruction. Am J Physiol Renal Physiol 2004; 286:1030 - 1038; PMID: 14722014; http://dx.doi.org/10.1152/ajprenal.00266.2003
- Fukuoka N, Sugiyama H, Inoue T, Kikumoto Y, Takiue K, Morinaga H, et al. Increased susceptibility to oxidant-mediated tissue injury and peritoneal fibrosis in acatalasemic mice. Am J Nephrol 2008; 28:661 - 668; PMID: 18337633; http://dx.doi.org/10.1159/000121357
- Odajima N, Betsuyaku T, Nagai K, Moriyama C, Wang DH, Takigawa T, et al. The role of catalase in pulmonary fibrosis. Respir Res 2010; 11:183; PMID: 21190578; http://dx.doi.org/10.1186/1465-9921-11-183
- Murthy S, Adamcakova-Dodd A, Perry SS, Tephly LA, Keller RM, Metwali N, et al. Modulation of reactive oxygen species by Rac1 or catalase prevents asbestos-induced pulmonary fibrosis. Am J Physiol Lung Cell Mol Physiol 2009; 297:846 - 855; PMID: 19684199; http://dx.doi.org/10.1152/ajplung.90590.2008
- Brezniceanu ML, Liu F, Wei CC, Chenier I, Godin N, Zhang SL, et al. Attenuation of interstitial fibrosis and tubular apoptosis in db/db transgenic mice overexpressing catalase in renal proximal tubular cells. Diabetes 2008; 57:451 - 459; PMID: 17977949; http://dx.doi.org/10.2337/db07-0013
- Maksimenko AV, Bezrukavnikova LM, Grigor'eva EL, Tishchenko EG, Arkhipova OG, Iaglov VV, et al. [Antifibrosis effect of modified forms of catalase and superoxide dismutase in experimental silicosis]. Vopr Med Khim 1992; 38:4 - 8; PMID: 1384235
- Cantin AM, Larivee P, Begin RO. Extracellular glutathione suppresses human lung fibroblast proliferation. Am J Respir Cell Mol Biol 1990; 3:79 - 85; PMID: 2363938
- Fang HL, Lai JT, Lin WC. Inhibitory effect of olive oil on fibrosis induced by carbon tetrachloride in rat liver. Clin Nutr 2008; 27:900 - 907; PMID: 18824281; http://dx.doi.org/10.1016/j.clnu.2008.08.004
- Yildiz H, Durmus AS, Simsek H, Yaman I. The comparison of methylene blue and vitamin E in prevention of abdominal postoperative adhesion formation in rat uterine horn models: Biochemical and histopathologic evaluation. Acta Cir Bras 2011; 26:51 - 57; PMID: 21271204
- Waghray M, Cui Z, Horowitz JC, Subramanian IM, Martinez FJ, Toews GB, et al. Hydrogen peroxide is a diffusible paracrine signal for the induction of epithelial cell death by activated myofibroblasts. FASEB J 2005; 19:854 - 856; PMID: 15857893
- Kasapovic J, Pejic S, Todorovic A, Stojiljkovic V, Pajovic SB. Antioxidant status and lipid peroxidation in the blood of breast cancer patients of different ages. Cell Biochem Funct 2008; 26:723 - 730; PMID: 18636415; http://dx.doi.org/10.1002/cbf.1499
- Kasapovic J, Pejic S, Stojiljkovic V, Todorovic A, Radosevic-Jelic L, Saicic ZS, et al. Antioxidant status and lipid peroxidation in the blood of breast cancer patients of different ages after chemotherapy with 5-fluorouracil, doxorubicin and cyclophosphamide. Clin Biochem 2010; 43:1287 - 1293; PMID: 20713039; http://dx.doi.org/10.1016/j.clinbiochem.2010.08.009
- Kumaraguruparan R, Subapriya R, Kabalimoorthy J, Nagini S. Antioxidant profile in the circulation of patients with fibroadenoma and adenocarcinoma of the breast. Clin Biochem 2002; 35:275 - 279; PMID: 12135688; http://dx.doi.org/10.1016/S0009-9120(02)00310-7
- Dilley TK, Bowden GT, Chen QM. Novel mechanisms of sublethal oxidant toxicity: induction of premature senescence in human fibroblasts confers tumor promoter activity. Exp Cell Res 2003; 290:38 - 48; PMID: 14516786; http://dx.doi.org/10.1016/S0014-4827(03)00308-2
- Fukino K, Shen L, Patocs A, Mutter GL, Eng C. Genomic instability within tumor stroma and clinicopathological characteristics of sporadic primary invasive breast carcinoma. JAMA 2007; 297:2103 - 2111; PMID: 17507346; http://dx.doi.org/10.1001/jama.297.19.2103
- Weber F, Shen L, Fukino K, Patocs A, Mutter GL, Caldes T, et al. Total-genome analysis of BRCA1/2-related invasive carcinomas of the breast identifies tumor stroma as potential landscaper for neoplastic initiation. Am J Hum Genet 2006; 78:961 - 972; PMID: 16685647; http://dx.doi.org/10.1086/504090
- Weber F, Xu Y, Zhang L, Patocs A, Shen L, Platzer P, et al. Microenvironmental genomic alterations and clinicopathological behavior in head and neck squamous cell carcinoma. JAMA 2007; 297:187 - 195; PMID: 17213402; http://dx.doi.org/10.1001/jama.297.2.187
- Magistretti PJ. Neuron-glia metabolic coupling and plasticity. J Exp Biol 2006; 209:2304 - 2311; PMID: 16731806; http://dx.doi.org/10.1242/jeb.02208
- Magistretti PJ. Role of glutamate in neuronglia metabolic coupling. Am J Clin Nutr 2009; 90:875 - 880; PMID: 19571222; http://dx.doi.org/10.3945/ajcn.2009.27462CC
- Magistretti PJ, Pellerin L. The contribution of astrocytes to the 18F-2-deoxyglucose signal in PET activation studies. Mol Psychiatry 1996; 1:445 - 452; PMID: 9154245
- Gotthardt M, Bleeker-Rovers CP, Boerman OC, Oyen WJ. Imaging of inflammation by PET, conventional scintigraphy, and other imaging techniques. J Nucl Med 2010; 51:1937 - 1949; PMID: 21078798; http://dx.doi.org/10.2967/jnumed.110.076232
- Kubota K, Nakamoto Y, Tamaki N, Kanegae K, Fukuda H, Kaneda T, et al. FDG-PET for the diagnosis of fever of unknown origin: a Japanese multi-center study. Ann Nucl Med 2011; 25:355 - 364
- Vos FJ, Bleeker-Rovers CP, Sturm PD, Krabbe PF, van Dijk AP, Cuijpers ML, et al. 18F-FDG PET/CT for detection of metastatic infection in gram-positive bacteremia. J Nucl Med 2010; 51:1234 - 1240; PMID: 20660384; http://dx.doi.org/10.2967/jnumed.109.072371
- Glaudemans AW, Signore A. FDG-PET/CT in infections: the imaging method of choice?. Eur J Nucl Med Mol Imaging 2010; 37:1986 - 1991; PMID: 20700737; http://dx.doi.org/10.1007/s00259-010-1587-x
- Tsuji AB, Morita M, Li XK, Sogawa C, Sudo H, Sugyo A, et al. 18F-FDG PET for semiquantitative evaluation of acute allograft rejection and immunosuppressive therapy efficacy in rat models of liver transplantation. J Nucl Med 2009; 50:827 - 830; PMID: 19372488; http://dx.doi.org/10.2967/jnumed.108.058925
- Reuter S, Schnockel U, Edemir B, Schroter R, Kentrup D, Pavenstadt H, et al. Potential of noninvasive serial assessment of acute renal allograft rejection by 18FFDG PET to monitor treatment efficiency. J Nucl Med 2010; 51:1644 - 1652; PMID: 20847180; http://dx.doi.org/10.2967/jnumed.110.078550
- Reuter S, Schnockel U, Schroter R, Schober O, Pavenstadt H, Schafers M, et al. Non-invasive imaging of acute renal allograft rejection in rats using small animal F-FDG-PET. PLoS ONE 2009; 4:5296; PMID: 19390685; http://dx.doi.org/10.1371/journal.pone.0005296
- Schnöckel U, Reuter S, Stegger L, Schlatter E, Schafers KP, Hermann S, et al. Dynamic 18F-fluoride small animal PET to noninvasively assess renal function in rats. Eur J Nucl Med Mol Imaging 2008; 35:2267 - 2274; PMID: 18622612; http://dx.doi.org/10.1007/s00259-0080878-y
- Pierce BL, Ballard-Barbash R, Bernstein L, Baumgartner RN, Neuhouser ML, Wener MH, et al. Elevated biomarkers of inflammation are associated with reduced survival among breast cancer patients. J Clin Oncol 2009; 27:3437 - 3444; PMID: 19470939; http://dx.doi.org/10.1200/JCO.2008.18.9068
- Mankoff DA, Dunnwald LK, Gralow JR, Ellis GK, Charlop A, Lawton TJ, et al. Blood flow and metabolism in locally advanced breast cancer: relationship to response to therapy. J Nucl Med 2002; 43:500 - 509; PMID: 11937594
- Specht JM, Kurland BF, Montgomery SK, Dunnwald LK, Doot RK, Gralow JR, et al. Tumor metabolism and blood flow as assessed by positron emission tomography varies by tumor subtype in locally advanced breast cancer. Clin Cancer Res 2010; 16:2803 - 2810; PMID: 20460489; http://dx.doi.org/10.1158/1078-0432.CCR-10-0026
- Dunnwald LK, Gralow JR, Ellis GK, Livingston RB, Linden HM, Specht JM, et al. Tumor metabolism and blood flow changes by positron emission tomography: relation to survival in patients treated with neoadjuvant chemotherapy for locally advanced breast cancer. J Clin Oncol 2008; 26:4449 - 4457; PMID: 18626006; http://dx.doi.org/10.1200/JCO.2007.15.4385
- Mankoff DA, Eary JF, Link JM, Muzi M, Rajendran JG, Spence AM, et al. Tumor-specific positron emission tomography imaging in patients: [18F] fluorodeoxyglucose and beyond. Clin Cancer Res 2007; 13:3460 - 3469; PMID: 17575208; http://dx.doi.org/10.1158/1078-0432.CCR-07-0074
- Juweid ME, Stroobants S, Hoekstra OS, Mottaghy FM, Dietlein M, Guermazi A, et al. Use of positron emission tomography for response assessment of lymphoma: consensus of the Imaging Subcommittee of International Harmonization Project in Lymphoma. J Clin Oncol 2007; 25:571 - 578; PMID: 17242397; http://dx.doi.org/10.1200/JCO.2006.08.2305
- Ambrosone CB, Abrams SM, Gorlewska-Roberts K, Kadlubar FF. Hair dye use, meat intake and tobacco exposure and presence of carcinogen-DNA adducts in exfoliated breast ductal epithelial cells. Arch Biochem Biophys 2007; 464:169 - 175; PMID: 17601487; http://dx.doi.org/10.1016/j.abb.2007.05.018
- Kinae N, Masuda H, Shin IS, Furugori M, Shimoi K. Functional properties of wasabi and horseradish. Biofactors 2000; 13:265 - 269; PMID: 11237192; http://dx.doi.org/10.1002/biof.5520130140
- Merliss RR. Talc-treated rice and Japanese stomach cancer. Science 1971; 173:1141 - 1142; PMID: 5098957; http://dx.doi.org/10.1126/science.173.4002.1141
- Khan N, Afaq F, Mukhtar H. Cancer chemoprevention through dietary antioxidants: progress and promise. Antioxid Redox Signal 2008; 10:475 - 510; PMID: 18154485; http://dx.doi.org/10.1089/ars.2007.1740
- López-Lázaro M. Dual role of hydrogen peroxide in cancer: possible relevance to cancer chemoprevention and therapy. Cancer Lett 2007; 252:1 - 8; PMID: 17150302; http://dx.doi.org/10.1016/j.canlet.2006.10.029
- Rui Q, Komori K, Tian Y, Liu H, Luo Y, Sakai Y. Electrochemical biosensor for the detection of H2O2 from living cancer cells based on ZnO nanosheets. Anal Chim Acta 2010; 670:57 - 62; PMID: 20685417; http://dx.doi.org/10.1016/j.aca.2010.04.065
- Van de Bittner GC, Dubikovskaya EA, Bertozzi CR, Chang CJ. In vivo imaging of hydrogen peroxide production in a murine tumor model with a chemoselective bioluminescent reporter. Proc Natl Acad Sci USA 2010; 107:21316 - 21321; PMID: 21115844; http://dx.doi.org/10.1073/pnas.1012864107
- Stolarek RA, Potargowicz E, Seklewska E, Jakubik J, Lewandowski M, Jeziorski A, et al. Increased H2O2 level in exhaled breath condensate in primary breast cancer patients. J Cancer Res Clin Oncol 2010; 136:923 - 930; PMID: 19967414; http://dx.doi.org/10.1007/s00432-009-0734-x
- Chan HP, Tran V, Lewis C, Thomas PS. Elevated levels of oxidative stress markers in exhaled breath condensate. J Thorac Oncol 2009; 4:172 - 178; PMID: 19179892; http://dx.doi.org/10.1097/JTO.0b013e3181949eb9
- Chan HP, Lewis C, Thomas PS. Exhaled breath analysis: novel approach for early detection of lung cancer. Lung Cancer 2009; 63:164 - 168; PMID: 18599152; http://dx.doi.org/10.1016/j.lungcan.2008.05.020
- Psathakis K, Mermigkis D, Papatheodorou G, Loukides S, Panagou P, Polychronopoulos V, et al. Exhaled markers of oxidative stress in idiopathic pulmonary fibrosis. Eur J Clin Invest 2006; 36:362 - 367; PMID: 16634841; http://dx.doi.org/10.1111/j.1365-2362.2006.01636.x
- Kobayashi Y, Nishikawa M, Hyoudou K, Yamashita F, Hashida M. Hydrogen peroxide-mediated nuclear factor kappaB activation in both liver and tumor cells during initial stages of hepatic metastasis. Cancer Sci 2008; 99:1546 - 1552; PMID: 18754865; http://dx.doi.org/10.1111/j.1349-7006.2008.00856.x