Abstract
DNA interstrand cross-links (ICLs) pose a significant threat to genomic and cellular integrity by blocking essential cellular processes, including replication and transcription. In mammalian cells, much ICL repair occurs in association with DNA replication during S phase, following the stalling of a replication fork at the block caused by an ICL lesion. Here, we review recent work showing that the XPF-ERCC1 endonuclease and the hSNM1A exonuclease act in the same pathway, together with SLX4, to initiate ICL repair, with the MUS81-EME1 fork incision activity becoming important in the absence of the XPF-SNM1A-SLX4-dependent pathway. Another nuclease, the Fanconi anemia-associated nuclease (FAN1), has recently been implicated in the repair of ICLs, and we discuss the possible ways in which the activities of different nucleases at the ICL-stalled replication fork may be coordinated. In relation to this, we briefly speculate on the possible role of SLX4, which contains XPF and MUS81- interacting domains, in the coordination of ICL repair nucleases.
A basic model of ICL repair has been built based on work performed in prokaryotes and lower eukaryotes (particularly budding yeast, S. cerevisiae), involving several major ICL repair pathways, as reviewed in references Citation1 and Citation2. Briefly, repair is initiated by the recognition of the lesion by nucleotide excision repair (NER) components, followed by incisions being made flanking the ICL. Such an “unhooking” reaction produces a gapped intermediate, which is subject to gap-filling either by translesion synthesis (TLS), or by homologous recombination (HR) if a sister chromatid is available. Subsequently, the gap-filled intermediate can be processed further, likely by a second round of NER, to remove the cross-linked oligonucleotide.
Mammalian ICL Repair
Data have been presented on mammalian systems that suggest pathways similar to those utilized by prokaryotes and lower eukaryotes may operate, especially in non-replicating cells.Citation3–Citation6 However, a key aspect of mammalian ICL repair is suggested to be its predominant occurrence during S phase, coupled to replication.Citation1,Citation7–Citation10 Such replication-dependent repair was first demonstrated when photoactivation of a psoralen lesion arrested synchronized human skin fibroblasts cells exclusively on passage through S phase, regardless of the cell cycle stage at which cross-linking was induced,Citation11 supported by the observation that repair of ICLs during S phase results in generation of double-strand breaks (DSBs), which is not evident in stationary yeast or Chinese hamster ovary cells.Citation12,Citation13 Additionally, there is a key difference between lower eukaryotes and mammalian cells as mammalian cells with a defective XPF-ERCC1 structure-specific endonuclease complex demonstrate increased ICL sensitivity,Citation12,Citation14–Citation16 suggesting a role for XPF-ERCC1 in ICL repair that is largely independent of its function in NER, also supported by the fact that XPF and ERCC1 defective cells accumulate excess replication-associated DNA DSBs when treated with cross-linking agents.Citation17
The replication-coupled model of ICL repair comes in two variations. The first one, modified from Niedernhofer et al.Citation8 proposes a single replication fork stalling at an ICL lesion, followed by incision by an (unspecified) endonuclease and a subsequent incision by XPF-ERCC1 to complete ICL unhooking. This allows TLS to occur past the tethered oligonucleotide, followed by a dual endonucleolytic incision and removal of the ICL from the second strand, and by gap-filling and HR steps to restore the replication fork (). More recently, an alternative model for replication-dependent ICL repair has been postulated to occur when two forks converge on a single ICLCitation18,Citation19 (). The physical relevance of this model was demonstrated using electron microscopy where two replication forks were observed to converge on a plasmid-based ICL in the presence of Xenopus egg extract. Repair in this context involves initial stalling of both replication forks 20–40 nucleotides from the lesion, followed by one of the leading strands advancing to within one nucleotide from the ICL. Subsequently, dual incisions of the ICL result in the formation of a two-sided DSB, which can be followed by lesion bypass synthesis and HR to restore the replication fork. Regardless of whether replication-coupled ICL repair occurs at a one-sided or a two-sided replication fork, the involvement and coordination of the activities of structure-specific endonucleases is essential during the unhooking steps of ICL repair.
Structure-Specific Endonucleases and Their Substrate Preference
As implied above, incision around the ICL is a key step in initiating the repair process, as it is a prerequisite for the processing of the repair intermediates by downstream pathways. A number of structure-specific endonucleases have been implicated in the incision events of ICL repair, including the canonical NER endonuclease, XPF-ERCC1 heterodimer,Citation12,Citation17,Citation20,Citation21 the MUS81-EME1 heterodimer,Citation22,Citation23 the SLX1-SLX4 heterodimer,Citation24–Citation30 and the Fanconi anemia-associated nuclease 1 (FAN1).Citation31–Citation33 Biochemically, the activities of these nucleases have been studied with non-cross-linked substrates and their activities described. The activities of MUS81-EME1 and XPF-ERCC1 have been recently reviewed in reference Citation34, and are illustrated in and B, respectively. Briefly, vertebrate XPF-ERCC1 has a preference for cleaving splayed-arm, bubble and stem-loop structures with a 3′-flap polarity, with hydrolysis occurring in the double-stranded (ds) DNA region close to the junction between ds and single-stranded (ss) DNA.Citation36–Citation38 The nuclease activity is dependent on residues that reside in the ERCC4 domain of the XPF proteinCitation39,Citation40 and ERCC1 has been postulated to play a role in directing the XPF-ERCC1 complex to its target site during NER.Citation41,Citation42 Meanwhile, MUS81-EME1 and its yeast homologs, while still cleaving a 3′-flap like structure, have a distinct specificity and preferentially cleave 3′-flap and replication fork as compared with splayed arm structures,Citation43–Citation45 together with some activity toward Holliday junction (HJ) resolution intermediates.Citation34,Citation35
Purified Slx1-Slx4 from yeast has been shown to be an endonuclease on 5′-flap, splayed arms and replication fork structures, with a lower level of activity toward static HJs.Citation46 Importantly, yeast Slx1 on its own is inactive, suggesting that Slx4 is required for supporting endonucleolytic activity, in analogy with MUS81-EME1 and XPF-ERCC1 heterodimers.Citation46,Citation47 While mammalian homologs of yeast Slx1 have been apparent, early attempts to identify Slx4 homologs were largely unsuccessful,Citation46 and these have been only recently described by several independent groups using more sophisticated PSI-BLAST methods.Citation24,Citation26,Citation28,Citation30 These groups have demonstrated that SLX1 and SLX4 interact to form a structure-specific endonuclease, with SLX1 being the catalytic and SLX4 the regulatory subunit. The human SLX4-SLX1 heterodimer exhibits hydrolytic activity toward 5′-flap, stem loop and replication fork structures, as well as being shown to be a HJ resolvase,Citation26,Citation28,Citation30 illustrated in . When SLX4-SLX1 was first described and its preference for cleaving 5′-flap structures demonstrated, in opposing polarity to XPF-ERCC1 and MUS81-EME1, it was proposed that SLX4-SLX1 might constitute a nuclease activity that could act together with XPF-ERCC1 (or MUS81) to unhook ICLs. However, since SLX4 interacts with both XPF-ERCC1 and MUS81-EME1, this picture and interpretation of cellular data has become more complex (see below).
The specificity of the recently identified FAN1 nuclease, illustrated in , has been studied by three independent groups.Citation31–Citation33 All suggest that FAN1 possesses 5′-exonuclease and structure-specific endonuclease activities. In terms of the endonuclease activity, Mackay et al. showed preferential activity on 5′-flap structures, with weaker activity on replication fork structures, in agreement with Smogorzewska et al.Citation33 with the latter activity not observed in Kratz et al. In addition, Smogorzewska et al. demonstrate endonuclease activity on nicked substrates opposite the nick, and also label the opposite strand to show that the major endonuclease activity cleaves the strand opposite the 5′-flap, with the equivalent strand also being hydrolyzed more readily within the replication fork structure. All three groups demonstrate a 5′-exonuclease activity, with a preference for ds over ssDNA and with an ability to digest DNA from a nicked or a recessed end.
Structure-Specific Endonucleases in ICL Incision
Despite progress to define the biochemical activities of the structure-specific endonucleases discussed above, only purified XPF-ERCC1 has been shown directly to incise ICL-containing substrates in vitro.Citation20,Citation21,Citation48 The other endonucleases have been implicated in ICL repair based on their depletion increasing sensitivity of cell and animal models to cross-linking agents.
MUS81-EME1 involvement in ICL repair is suggested as mouse embryonic stem cells disrupted of MUS81 or EME1 are sensitive to mitomycin C (MMC) Citation23,Citation49 and fail to form DSBs in response to long exposure of MMC, suggesting MUS81mediated DSB formation.Citation23 However, the same study found formation of DSBs in response to pulsed treatment of MMC to be apparently independent of MUS81, and another conflicting report implies normal DSB formation and persistent Rad51 foci in mus81-/- cells, suggesting a role for MUS81 in later stages of repair.Citation50 In our recent study using human cell lines, we propose a role for MUS81-mediated DSB formation as a late response in repair during S phase, which is important for completion of repair and replication when the XPF-hSNM1A-dependent pathway fails.Citation48
Together with the biochemical data implicating XPF-ERCC1 in ICL repair,Citation20,Citation21,Citation48 this endonuclease is implicated in ICL repair by increased sensitivity of Chinese hamster ovary cells defective in XPF or ERCC1 to nitrogen mustard (HN2) and MMC as compared with those defective in other NER factors.Citation12,Citation14 This hypersensitivity is associated with an unhooking defect,Citation12 consistent with findings from the biochemical studies showing that purified XPF-ERCC1 can make incisions on both the 5′ and 3′ sides of a psoralen ICL placed on a duplex with splayed arms structure.Citation20,Citation21 Although these studies imply that incisions do not take place in the context of a linear substrate, other work suggested that XPF-ERCC1 can incise ICLs within linear DNA.Citation51–Citation53 In our recent study, we found that XPF-ERCC1 was able to incise 5′ to a site-specific SJG-136 ICL within a linear duplex, albeit with low efficiency, a reaction that does not occur on non-crosslinked substrate.Citation48 The low efficiency of purified XPF-ERCC1 activity in vitro may be due to the need for additional co-factors, for example XPF-ERCC1 enzymatic activity has been shown to be stimulated by a scaffold protein, SLX4.Citation28
As discussed below, the importance of SLX4 in ICL repair is likely to be related to its role as a scaffold protein rather than exclusively to its role as the regulatory co-factor for the SLX1 nuclease. It has been shown that SLX4 contains XPF-ERCC1 and MUS81-EME1 interacting domains in addition to interacting with SLX1Citation26,Citation28,Citation30 and other proteins, including TRF2/RAP1, the MSH2-MSH3 mismatch repair complex and the polo-like kinase PLK1,Citation30 and has thus been postulated to act as a scaffold protein for recruiting the XPF-ERCC1 and MUS81-EME1 structure specific endonucleases to the sites of ICLs.Citation24–Citation30 SLX4 is implicated in ICL repair, since cells depleted of SLX4 but not SLX1 are hypersensitive to cross-linking agents.Citation24,Citation26,Citation28,Citation30 Cells deficient in SLX4 have also been shown to be defective in repair of ICL-induced DSBs, as marked by persistence of γH2AX activation following cisplatin treatment,Citation26,Citation28 but this could also be related to defective HJ resolvase activity of the SLX4-SLX1 complex and, thus, impaired HR in later stages of ICL-induced DSB resolution.
Finally the Fanconi anemia (FA)-associated nuclease, FAN1, was identified recently in a genome-wide screen for MLH1-interacting factors and is required for resistance to MMC.Citation31–Citation33,Citation54,Citation55 FAN1 was shown to physically interact with the monoubiquitinated form of FANCD2 via the UBZ domain,Citation31–Citation33,Citation55 and size-exclusion chromatography implicated FAN1 in two sub-complexes,Citation32 one containing MLH1 and the other FANCD2/FANCI. FAN1 is likely involved in ICL repair, since FAN1-depleted cells show specific sensitivity to MMC and cisplatin,Citation31–Citation33 although one study also reports sensitivity to camptothecin.Citation33 The role of FAN1 in ICL repair appears to be evolutionarily conserved as the C. elegans FAN1 mutant (tm423) showed increased embryonic lethality compared with wild-type following MMC, cisplatin or HN2 treatment.Citation31–Citation33 FAN1-depleted cells showed persistence of γH2AX, replication protein A and Rad51 foci following cisplatin treatment, implicating FAN1 in DSB resolution.Citation31–Citation33 Finally, FAN1-depleted cells are also defective in HR repair as directly measured by the GFP reporter assay, suggesting a role for FAN1 in HR-mediated DSB resolution during the final stages of ICL repair.Citation31,Citation32
Trimming Activities at an ICL
Regardless of the steps involved in the incision of an ICL and whether a replication-dependent or replication-independent repair model operates, one of the downstream processing steps involves TLS to synthesize new DNA opposite the damaged base that remains tethered to the unhooked oligonucleotide. The role of the different translesion polymerases in ICL repair has been reviewed recently in reference Citation56, but a key observation that has been made suggests that TLS is more efficient when the oligonucleotide tethered to the unhooked cross-link has been resected.Citation57,Citation58 Moreover, direct evidence of trimming to a single tethered nucleotide was obtained in the recent studies of replication coupled ICL repair in the Xenopus cell-free system.Citation19 Together, this strongly suggests a need for an enzyme to carry out such exonucleolytic processing of the tethered oligonucleotide, as illustrated by .
A good candidate for taking this role in human cells is hSNM1A, a homolog of the Pso2 factor, first identified in two independent screens in budding yeast for mutants sensitive to cross-linking agents.Citation59–Citation61 Homologs of yeast Pso2 in higher eukaryotes have been identified as SNM1A, SNM1B (also known as Apollo) and SNM1C (Artemis), structurally all members of the β-CASP family of metallo-β-lactamase-related nucleases, reviewed in references Citation62 and Citation63. Human SNM1A has been implicated in ICL repair due to its ability to partially rescue the pso2 defect in yeast cells, unlike hSNM1B and hSNM1C.Citation64 Crucially for the functional relevance of hSNM1A in ICL repair, both yeast Pso2 Citation65 and human SNM1ACitation64,Citation66 display 5′-exonuclease activity in vitro. In our recently published study, we have confirmed this activity and have shown, in addition, that hSNM1A can hydrolyze cross-linked substrates past a site-specific SJG-136 cross-link in vitro, with an ability to start digestion from a nick provided by XPF-ERCC1 endonuclease, suggesting hSNM1A's involvement as an ICL “trimming” exonuclease.Citation48
In terms of cellular sensitivity, three mice knockout models of SNM1A have been published to date, all suggesting a modest increase in sensitivity to MMC, but not to melphalan, cisplatin, IR or UV, of snm1a-/- mouse embryonic fibroblasts (MEFs),Citation67–Citation69 which could be complemented by hSNM1A.Citation68 In support, hypersensitivity to a range of cross-linking agents was observed in snm1a-/- chicken DT40 cells,Citation70 although interestingly in the DT40 model, deletion of either SNM1A or SNM1B results in sensitivity to cisplatin and MMC, suggesting a possible redundancy between the two metalloβ-lactamase structural family enzymes. In contrast, SNM1C-depleted cells were found to be sensitive to IR but not to cross-linking agents.
Biochemically, the related exonuclease hSNM1B/Apollo, another member of the β-CASP family of metallo-β-lactamase related nucleases, has also been shown to posses 5′-exonuclease activity,Citation71,Citation72 which is stimulated by the TRF2 telomere-associated factor, although this activity has been postulated to be involved in telomere processing. In cellular studies, as mentioned above, SNM1B disruption in chicken DT40 cells results in hypersensitivity to cross-linking agents,Citation70 but the role of human SNM1B in DNA repair is not entirely resolved, with some studies indicating sensitivity of hSNM1B-depleted cells to cross-linking agents and IR and others a much more drastic increase in sensitivity to MMC but not to IR.Citation73–Citation76 The function of hSNM1B in ICL repair has also been attributed to a possible role in mediating checkpoint signaling, although conflicting views exist on which aspects of signaling might be linked to hSNM1B.Citation73,Citation75,Citation77,Citation78 It remains of interest whether the exonuclease activity of hSNM1B is able to digest cross-linked DNA past a lesion in analogy to hSNM1A, and the possible redundancy between the hSNM1A and hSNM1B cross-link trimming activities remains an area for investigation. Although hSNM1C/Artemis has previously been proposed to also act as a 5′-exonucleaseCitation79,Citation80 as well as a structure-specific endonuclease in nonhomologous end-joining and V(D)J recombination, this was shown more recently to have been a contaminating activity and a consequence of the purification method.Citation81 It is worth noting that the entire SNM1 family has also been proposed to have roles independent of their nuclease activity, including in mitotic cell cycle checkpoints, and this remains a topic for investigation.Citation63,Citation82
Finally, the recently identified FAN1 nuclease has been shown to have a 5′-exonuclease activity with a high specificity for nicked substrates,Citation31–Citation33 which would clearly be a good candidate for trimming the tethered oligonucleotide prior to TLS. However, the FAN1 activity has so far been only demonstrated on unmodified substrate, so it remains of interest to study this activity on substrates containing ICLs. To this end, it is helpful that methods have been developed recently to make ICL-containing substrates with high efficiency and high specificity, as discussed in our work with relation to the SJG-136 compound,Citation48,Citation83 and in the work of the Schärer and Miller groups, who have developed methods to generate DNA ICLs using post-synthetic reductive amination or an approach combining solid phase oligonucleotide synthesis with selective removal of protecting groups of a cross-linked thymidine dimer.Citation57,Citation84–Citation86 Although there is a possible role for FAN1 in trimming a tethered oligonucleotide, it is worth noting that the groups that have identified FAN1 propose a possible role for its 5′-exonuclease activity in processing DNA strands to generate 3′ overhangs to allow strand invasion and HR, required for the restart of the replication fork once the cross-link is removed.Citation31–Citation33 It is also worth noting that rodents may use FAN1 and SNM1A redundantly, explaining the relatively mild phenotype of SNM1Adeficient mice.
Coordinating the Nucleases at an ICL
A key question to answer is whether all of the different endonucleases are involved in the incision step, or whether they are recruited specifically and sequentially to ICL sites depending on the context in which the ICLs are recognized at different stages of the cell cycle. Second, it remains to be resolved how the exo and endonucleases are coordinated spatially and temporally during ICL repair. Based on the observation that mus81-/- MEFs appear to have suppressed DSB formation,Citation23 while ercc1-/- MEFs accumulate DSBs,Citation17 the model for replication-dependent ICL repair illustrated in has previously been proposed. Here the initiating incision is made by MUS81, possibly on the leading strand template of the replication fork, followed by a second XPF-ERCC1-dependent incision 5′ to the ICL, which results in ICL unhooking, accepted to be a prerequisite for downstream repair processes such as TLS and HR.Citation17,Citation23,Citation87 However, evidence for the order in which XPF-ERCC1- and MUS81-mediated incisions occur had remained inconclusive. In our recent study, we now provide evidence supporting a differential employment of incision activities of XPF-ERCC1 and MUS81-EME1 depending on the context in which the ICL is detected by the replication fork. We found that depleting ERCC1 results in an increase in DSB formation, which was MUS81-dependent, a result reminiscent of hSNM1A and SLX4/FANCP depletion, implying that MUS81 acts on repair intermediates that persist when the XPF-ERCC1/SLX4/hSNM1A controlled pathway fails.Citation48 This suggested that MUS81-mediated incision is not the initiating event during ICL repair normally, but has increased importance when ICLs persist, or in late S phase when completion of replication requires DSB ends for HR, as illustrated by our proposed context-dependent ICL repair models in .
To date, three important regulators of ICL repair have been identified, FANCD2-FANCI, SLX4 and RAD18. It appears that the FANCD2-FANCI complex plays a pivotal role in controlling the incision step.Citation18,Citation88 FANCD2 ubiquitination occurs rapidly in response to cross-linking agent treatment, as a result of replication fork stalling triggering the ATR signaling, as evident by similar kinetics to Chk1 phosphorylation. However, whether the activation of FANCD2 is in response to replication fork stalling or to the presence of ICL per se is unclear. Furthermore, how FANCD2 controls incision events remains to be determined, although we have previously shown that XPF-ERCC1 influences dynamics of FANCD2 chromatin association.Citation87 Additionally, the recent structure of the FANCD2-FANCI complex implicates monoubiquitination, as well regulatory phosphorylation, in stabilization of the FANCD2-FANCI heterodimer.Citation89 The identification of the FAN1 nuclease that interacts with monoubiquitinated FANCD2 may provide the missing link between FANCD2 and incision. All three groups that identified FAN1 propose the possibility that FAN1 is involved in an early incision step at the ICL, acting in concert with but with opposite polarity to (5′-flap rather than 3′-flap specificity) MUS81 (or XPF-ERCC1) to cleave the same strand on the opposite side of the ICL (). However, it is acknowledged that there are problems with this proposal, since it is difficult to see how MUS81 (or XPF-ERCC1) would only cleave one of the two leading strands of the converged replication forks.Citation31,Citation32 Cleavage of both forks would result in two DSBs and a linear duplex containing an ICL, and from the proposed biochemistry of the enzyme this is unlikely to be a substrate for FAN1. Additionally, Mackay et al. and Kratz et al. also investigated how depletion of FAN1 affects the appearance and persistence of DSBs in response to cisplatin or MMC treatment and show that reducing FAN1 does not prevent DSB formation but slows down DSB disappearance.Citation31,Citation32 Since DSBs formed in ICL repair are removed predominantly by HR, this may implicate FAN1 in late stages of ICL repair, potentially in DSB resection through its 5′ exonuclease activity.Citation31–Citation33
SLX4 has been suggested to be an important regulator of incision as it is found to interact with XPF-ERCC1 and MUS81-EME1 as well as SLX1, which has led to a proposed role for SLX4 in controlling the nuclease activity at ICL-stalled replication forks. Our data indicate that SLX4 acts in the same pathway as XPF-ERCC1 and hSNM1A,Citation48 and the fact that chromatin recruitment of XPF-ERCC1 is dependent on SLX4 Citation25,Citation27,Citation29 makes SLX4 an attractive candidate for acting as a regulator of the recruitment of repair nucleases, particularly XPF-ERCC1. However, SLX4-deficient cells also accumulate ICL-associated DSBs,Citation48 suggesting that MUS81 recruitment to stalled replication forks may also be controlled by another factor independently of the SLX4-MUS81 interaction. Crucially, SLX4 has been recently shown to be a new Fanconi anemia factor, FANCP, providing another key link between the FA pathway and ICL repair.Citation25,Citation27,Citation29 However, SLX4 has been termed as the downstream effector of the FA pathway, since SLX4/FANCP patient cells show normal ubiquitination of FANCD2.Citation25,Citation27,Citation29 It remains to be determined if FANCD2 controls ICL repair through SLX4, or if SLX4 acts in parallel with FANCD2-FANCI, providing another layer of regulation of the ICL repair process. Finally, it has been recently suggested that RAD18 and ubiquitinated PCNA might be involved in targeting hSNM1A to damaged replication forks,Citation90 possibly providing a means of targeting this protein to repair intermediates requiring exonucleolytic trimming. In this regard, several recent publications suggest that RAD18 contributes to the FA-mediated repair, as PCNA K164 monoubiquitination appears to promote full activation of the FA pathway.Citation91–Citation93 This would potentially provide an attractive means of co-opting hSNM1A into FA-controlled repair processes, and coordinating this with TLS-mediated downstream steps also involving monoubiquinated PCNA. In fact, RAD18 has also been recently implicated in chromatin localization of FANCD2-FANCI,Citation94 further supporting its role as a coordinator involved in ICL repair.
Future Directions
Work published recently by our group and others suggests that there is more than one system for the repair of ICLs.Citation6,Citation19,Citation31–Citation33,Citation48,Citation95 Clearly, the regulation of the choice of repair mechanism represents the next major challenge in the field. While our data suggest that SLX4 acts in the same pathway as XPF-ERCC1 and hSNM1A, which together with the known interaction of SLX4 and XPF-ERCC1 indicates a possible role for SLX4 as a regulator of the pathway, a number of outstanding questions remain to be addressed in the proposed model of ICL repair. In particular, delineation of the order of recruitment of different nucleases to the chromatin will be critical. We have attempted to examine the chromatin recruitment of XPF-ERCC1 and MUS81-EME1 nucleases following cross-linking agent treatment, but this has proven technically difficult as the nucleases appear to be constitutively associated with chromatin (Wang AT and McHugh PJ, unpublished data). Thus examining the localization of fluorochrome-tagged nucleases as well employing proximity ligation assays following cross-linking agent treatment could provide an alternative approach for determining the spatial and temporal relationships of key factors deployed in ICL repair. It would be of particular interest to investigate whether or not depletion of SLX4, the putative nuclease coordinator, affects the timing and location of nuclease recruitment.
Further understanding of the mechanistic details of ICL repair could be derived from a comprehensive analysis of the substrate specificity for each of the nucleases, with particular emphasis on using crosslinked substrates, such as in the studies discussed previously in references Citation20 and Citation21. It will be helpful to utilize the recently described methods for making crosslinked substrates with high specificity and high efficiency,Citation48,Citation57,Citation84–Citation86 introducing a site-selective ICL in the context of a variety of replication fork-like substrates, including leading and lagging strands and converged replication forks. In particular, the interplay between the various nucleases, which have been highly purified in recombinant formCitation31–Citation33,Citation40,Citation48,Citation66 and the SLX4 scaffold proteinCitation28,Citation30 in vitro will be of interest as it is likely that a number of the proteins require the presence and/or activity of other proteins to support their function.
Although it may suggest which steps of ICL the nucleases participate in, the in vitro approach would clearly need to be validated in the in vivo setting. Therefore, it will be important to identify and confirm direct interactions between the nucleases and associated coordinator factors in order to better understand the dynamics of ICL repair. The interaction of SLX4 with both XPF and MUS81 Citation24,Citation26,Citation28,Citation30 is a key area for investigation, especially since the two nucleases appear to have distinct roles during ICL repair, and so it will be interesting to examine the physical interaction of SLX4 with XPF/MUS81, and how it varies during the different stages of ICL repair. Furthermore, the significance of the interaction between FANCD2 and FAN1 during ICL repair, and the interplay between this interaction and the SLX4-XPF/MUS81 interactions remains to be determined. Finally, how hSNM1A is targeted to ICLs has only recently begun to be examined, and this will shed further light on the control of this important repair pathway.
Abbreviations
DSB | = | double-stranded break |
dsDNA/ssDNA | = | double/single-stranded DNA |
FA | = | fanconi anemia |
HJ | = | holliday junction |
HN2 | = | nitrogen mustard |
HR | = | homologous recombination |
ICL | = | interstrand cross-link |
MEF | = | mouse embryonic fibroblast |
MMC | = | mitomycin C |
NER | = | nucleotide excision repair |
TLS | = | translesion synthesis |
Figures and Tables
Figure 1 Existing models for replication coupled ICL repair in mammalian cells. (A) Model for the repair of ICLs during S phase in mammalian cells as adapted from Niedernhofer et al.Citation17 (B) Converging fork model of ICL repair, as based on Knipscheer et al. and raschle et al.Citation18,Citation19
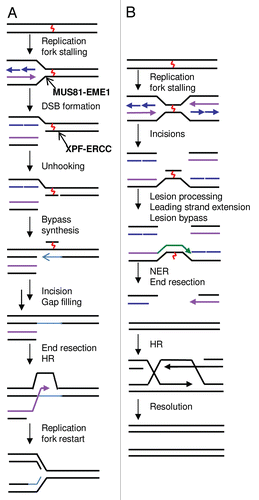
Figure 2 Nuclease activity of structure-specific endonucleases implicated in ICL repair. The substrate preference for human (A) MUS81-EME1, (B) XPF-ERCC1, (C) SLX1–SLX4 and (D) FAN1 is summarized. The region of cleavage is indicated by a red arrow in the DNA structure. The preference of cleaving one DNA substrate over another is indicated by chevron arrows, commas denote similar levels of incision efficiency. Figure adapted from Ciccia et al., Svendsen et al. and Smogorzewska et al.Citation33–Citation35
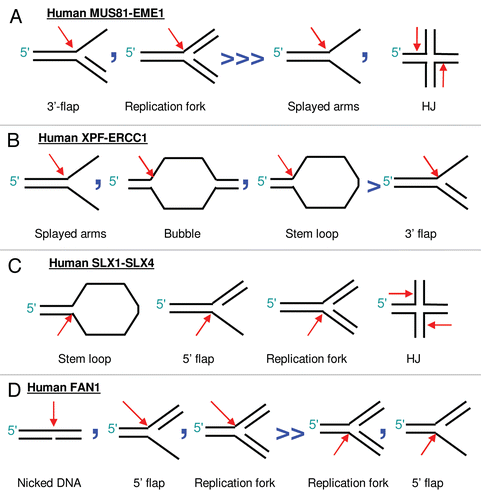
Figure 3 Translesion synthesis is more efficient following trimming of the tethered oligonucleotide. (A) Increasing efficiency of bypass synthesis/TLS as the cross-linked oligonucleotide is shortened. (B) the hSNM1A exonuclease is able to digest DNA past a cross-linked base, as described by our recent study in reference Citation48.
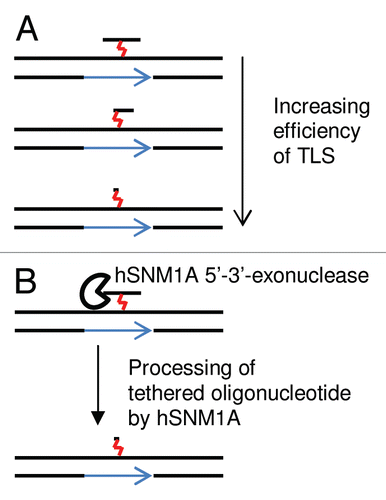
Figure 4 Role of structure-specific endonucleases in ICL repair. (A) the initial steps of the models for context-dependent ICL repair during replication, discussed in our recent study in reference Citation48, and in this review. The initial incisions could be on either the (i) leading or (ii) lagging strand or (iii) two replication forks converge on an ICL, more likely during late S phase or in the absence of the XPF-ERCC1/hSNM1A pathway. (B) Potential roles for the recently identified FAN1 nuclease in ICL repair, based on models proposed by Kratz et al., Mackay et al. and Smogorzewska et al.Citation31–Citation33 (i) when two replication forks converge, FAN1 could be involved in early endonucleolytic incision of the replication forks, in the removal of the cross-linked oligonuclotide from the second strand or in later steps required for HR, including ssDNA resection or 5′-flap incisions during HJ resolution. (ii) In a single stalled replication fork model, FAN1 may also function in later steps required for HR, including ssDNA resection or D-loop incision.
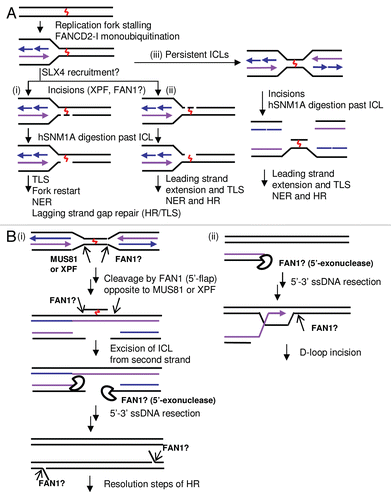
Acknowledgments
This work was supported by a Cancer Research UK Programme Grant to P.Mc, and a New Zealand Top Achiever Scholarship to A.T.W.
References
- Dronkert ML, Kanaar R. Repair of DNA inter-strand cross-links. Mutat Res 2001; 486:217 - 247; PMID: 11516927
- Lehoczký P, McHugh PJ, Chovanec M. DNA interstrand cross-link repair in Saccharomyces cerevisiae. FEMS Microbiol Rev 2007; 31:109 - 133; PMID: 17096663; http://dx.doi.org/10.1111/j.15746976.2006.00046.x
- Bessho T, Mu D, Sancar A. Initiation of DNA inter-strand cross-link repair in humans: the nucleotide excision repair system makes dual incisions 5′ to the cross-linked base and removes a 22- to 28-nucleotide-long damage-free strand. Mol Cell Biol 1997; 17:6822 - 6830; PMID: 9372913
- Muniandy PA, Thapa D, Thazhathveetil AK, Liu ST, Seidman MM. Repair of laser-localized DNA interstrand cross-links in G1 phase mammalian cells. J Biol Chem 2009; 284:27908 - 27917; PMID: 19684342; http://dx.doi.org/10.1074/jbc.M109.029025
- Smeaton MB, Hlavin EM, McGregor Mason T, Noronha AM, Wilds CJ, Miller PS. Distortion-dependent unhooking of interstrand cross-links in mammalian cell extracts. Biochemistry 2008; 47:9920 - 9930; PMID: 18702509; http://dx.doi.org/10.1021/bi800925e
- Ben-Yehoyada M, Wang LC, Kozekov ID, Rizzo CJ, Gottesman ME, Gautier J. Checkpoint signaling from a single DNA interstrand cross-link. Mol Cell 2009; 35:704 - 715; PMID: 19748363; http://dx.doi.org/10.1016/j.molcel.2009.08.014
- McHugh PJ, Spanswick VJ, Hartley JA. Repair of DNA interstrand cross-links: molecular mechanisms and clinical relevance. Lancet Oncol 2001; 2:483 - 490; PMID: 11905724; http://dx.doi.org/10.1016/S14702045(01)00454-5
- Niedernhofer LJ, Lalai AS, Hoeijmakers JH. Fanconi anemia (cross)linked to DNA repair. Cell 2005; 123:1191 - 1198; PMID: 16377561; http://dx.doi.org/10.1016/j.cell.2005.12.009
- Patel KJ, Joenje H. Fanconi anemia and DNA replication repair. DNA Repair (Amst) 2007; 6:885 - 890; PMID: 17481966; http://dx.doi.org/10.1016/j.dnarep.2007.02.002
- Thompson LH, Hinz JM. Cellular and molecular consequences of defective Fanconi anemia proteins in replication-coupled DNA repair: mechanistic insights. Mutat Res 2009; 668:54 - 72; PMID: 19622404; http://dx.doi.org/10.1016/j.mrfmmm.2009.02.003
- Akkari YM, Bateman RL, Reifsteck CA, Olson SB, Grompe M. DNA replication is required to elicit cellular responses to psoralen-induced DNA inter-strand cross-links. Mol Cell Biol 2000; 20:8283 - 8289; PMID: 11027296; http://dx.doi.org/10.1128/MCB.20.21.82839.2000
- De Silva IU, McHugh PJ, Clingen PH, Hartley JA. Defining the roles of nucleotide excision repair and recombination in the repair of DNA inter-strand cross-links in mammalian cells. Mol Cell Biol 2000; 20:7980 - 7990; PMID: 11027268; http://dx.doi.org/10.1128/MCB.20.21.7980-90.2000
- McHugh PJ, Sones WR, Hartley JA. Repair of intermediate structures produced at DNA interstrand cross-links in Saccharomyces cerevisiae. Mol Cell Biol 2000; 20:3425 - 33; PMID: 10779332; http://dx.doi.org/10.1128/MCB.20.10.3425-33.2000
- Andersson BS, Sadeghi T, Siciliano MJ, Legerski R, Murray D. Nucleotide excision repair genes as determinants of cellular sensitivity to cyclophosphamide analogs. Cancer Chemother Pharmacol 1996; 38:406 - 416; PMID: 8765433; http://dx.doi.org/10.1007/s002800050504
- Damia G, Imperatori L, Stefanini M, D'Incalci M. Sensitivity of CHO mutant cell lines with specific defects in nucleotide excision repair to different anti-cancer agents. Int J Cancer 1996; 66:779 - 783; PMID: 8647649; http://dx.doi.org/10.1002/(SICI)1097-0215(19960611)66:6<779::AIDIJC12>3.0.CO;2-Z
- Hoy CA, Thompson LH, Mooney CL, Salazar EP. Defective DNA cross-link removal in Chinese hamster cell mutants hypersensitive to bifunctional alkylating agents. Cancer Res 1985; 45:1737 - 1743; PMID: 3919945
- Niedernhofer LJ, Odijk H, Budzowska M, van Drunen E, Maas A, Theil AF, et al. The structure-specific endonuclease Ercc1-Xpf is required to resolve DNA interstrand cross-link-induced double-strand breaks. Mol Cell Biol 2004; 24:5776 - 57787; PMID: 15199134; http://dx.doi.org/10.1128/MCB.24.13.577687.2004
- Knipscheer P, Raschle M, Smogorzewska A, Enoiu M, Ho TV, Scharer OD, et al. The Fanconi anemia pathway promotes replication-dependent DNA inter-strand cross-link repair. Science 2009; 326:1698 - 1701; PMID: 19965384; http://dx.doi.org/10.1126/science.1182372
- Räschle M, Knipsheer P, Enoiu M, Angelov T, Sun J, Griffith JD, et al. Mechanism of replication-coupled DNA interstrand cross-link repair. Cell 2008; 134:969 - 980; PMID: 18805090; http://dx.doi.org/10.1016/j.cell.2008.08.030
- Fisher LA, Bessho M, Bessho T. Processing of a psoralen DNA interstrand cross-link by XPF-ERCC1 complex in vitro. J Biol Chem 2008; 283:1275 - 1281; PMID: 18006494; http://dx.doi.org/10.1074/jbc.M708072200
- Kuraoka I, Kobertz WR, Ariza RR, Biggerstaff M, Essigmann JM, Wood RD. Repair of an inter-strand DNA cross-link initiated by ERCC1-XPF repair/recombination nuclease. J Biol Chem 2000; 275:26632 - 26636; PMID: 10882712; http://dx.doi.org/10.1074/jbc.C000337200
- Hanada K, Budzowska M, Davies SL, van Drunen E, Onizawa H, Beverloo HB, et al. The structure-specific endonuclease Mus81 contributes to replication restart by generating double-strand DNA breaks. Nat Struct Mol Biol 2007; 14:1096 - 1104; PMID: 17934473; http://dx.doi.org/10.1038/nsmb1313
- Hanada K, Budzowska M, Modesti M, Maas A, Wyman C, Essers J, et al. The structure-specific endonuclease Mus81-Eme1 promotes conversion of inter-strand DNA cross-links into double-strands breaks. EMBO J 2006; 25:4921 - 4932; PMID: 17036055; http://dx.doi.org/10.1038/sj.emboj.7601344
- Andersen SL, Bergstralh DT, Kohl KP, LaRocque JR, Moore CB, Sekelsky J. Drosophila MUS312 and the vertebrate ortholog BTBD12 interact with DNA structure-specific endonucleases in DNA repair and recombination. Mol Cell 2009; 35:128 - 135; PMID: 19595722; http://dx.doi.org/10.1016/j.molcel.2009.06.019
- Crossan GP, van der Weyden L, Rosado IV, Langevin F, Gaillard PH, McIntyre RE, et al. Disruption of mouse Slx4, a regulator of structure-specific nucleases, phenocopies Fanconi anemia. Nat Genet 2011; 43:147 - 152; PMID: 21240276; http://dx.doi.org/10.1038/ng.752
- Fekairi S, Scaglione S, Chahwan C, Taylor ER, Tissier A, Coulon S, et al. Human SLX4 is a Holliday junction resolvase subunit that binds multiple DNA repair/recombination endonucleases. Cell 2009; 138:78 - 89; PMID: 19596236; http://dx.doi.org/10.1016/j.cell.2009.06.029
- Kim Y, Lach FP, Desetty R, Hanenberg H, Auerbach AD, Smogorzewska A. Mutations of the SLX4 gene in Fanconi anemia. Nat Genet 2011; 43:142 - 146; PMID: 21240275; http://dx.doi.org/10.1038/ng.750
- Muñoz IM, Hain K, Declais AC, Gardiner M, Toh GW, Sanchez-Pulido L, et al. Coordination of structure-specific nucleases by human SLX4/BTBD12 is required for DNA repair. Mol Cell 2009; 35:116 - 127; PMID: 19595721; http://dx.doi.org/10.1016/j.molcel.2009.06.020
- Stoepker C, Hain K, Schuster B, Hilhorst-Hofstee Y, Rooimans MA, Steltenpool J, et al. SLX4, a coordinator of structure-specific endonucleases, is mutated in a new Fanconi anemia subtype. Nat Genet 2011; 43:138 - 141; PMID: 21240277; http://dx.doi.org/10.1038/ng.751
- Svendsen JM, Smogorzewska A, Sowa ME, O'Connell BC, Gygi SP, Elledge SJ, et al. Mammalian BTBD12/SLX4 assembles a Holliday junction resolvase and is required for DNA repair. Cell 2009; 138:63 - 77; PMID: 19596235; http://dx.doi.org/10.1016/j.cell.2009.06.030
- Kratz K, Schopf B, Kaden S, Sendoel A, Eberhard R, Lademann C, et al. Deficiency of FANCD2-associated nuclease KIAA1018/FAN1 sensitizes cells to interstrand cross-linking agents. Cell 2010; 142:77 - 88; PMID: 20603016; http://dx.doi.org/10.1016/j.cell.2010.06.022
- MacKay C, Declais AC, Lundin C, Agostinho A, Deans AJ, MacArtney TJ, et al. Identification of KIAA1018/FAN1, a DNA repair nuclease recruited to DNA damage by monoubiquitinated FANCD2. Cell 2010; 142:65 - 76; PMID: 20603015; http://dx.doi.org/10.1016/j.cell.2010.06.021
- Smogorzewska A, Desetty R, Saito TT, Schlabach M, Lach FP, Sowa ME, et al. A genetic screen identifies FAN1, a Fanconi anemia-associated nuclease necessary for DNA interstrand cross-link repair. Mol Cell 2010; 39:36 - 47; PMID: 20603073; http://dx.doi.org/10.1016/j.molcel.2010.06.023
- Ciccia A, McDonald N, West SC. Structural and functional relationships of the XPF/MUS81 family of proteins. Annu Rev Biochem 2008; 77:259 - 287; PMID: 18518821; http://dx.doi.org/10.1146/annurev.biochem.77.070306.102408
- Svendsen JM, Harper JW. GEN1/Yen1 and the SLX4 complex: Solutions to the problem of Holliday junction resolution. Genes Dev 2010; 24:521 - 536; PMID: 20203129; http://dx.doi.org/10.1101/gad.1903510
- de Laat WL, Appeldoorn E, Jaspers NG, Hoeijmakers JH. DNA structural elements required for ERCC1XPF endonuclease activity. J Biol Chem 1998; 273:7835 - 7842; PMID: 9525876; http://dx.doi.org/10.1074/jbc.273.14.7835
- Park CH, Bessho T, Matsunaga T, Sancar A. Purification and characterization of the XPF-ERCC1 complex of human DNA repair excision nuclease. J Biol Chem 1995; 270:22657 - 22660; PMID: 7559382; http://dx.doi.org/10.1074/jbc.270.39.22657
- Sijbers AM, de Laat WL, Ariza RR, Biggerstaff M, Wei YF, Moggs JG, et al. Xeroderma pigmentosum group F caused by a defect in a structure-specific DNA repair endonuclease. Cell 1996; 86:811 - 822; PMID: 8797827; http://dx.doi.org/10.1016/S00928674(00)80155-5
- Aravind L, Walker DR, Koonin EV. Conserved domains in DNA repair proteins and evolution of repair systems. Nucleic Acids Res 1999; 27:1223 - 1242; PMID: 9973609; http://dx.doi.org/10.1093/nar/27.5.1223
- Enzlin JH, Scharer OD. The active site of the DNA repair endonuclease XPF-ERCC1 forms a highly conserved nuclease motif. EMBO J 2002; 21:2045 - 2053; PMID: 11953324; http://dx.doi.org/10.1093/emboj/21.8.2045
- Tripsianes K, Folkers G, Ab E, Das D, Odijk H, Jaspers NG, et al. The structure of the human ERCC1/XPF interaction domains reveals a complementary role for the two proteins in nucleotide excision repair. Structure 2005; 13:1849 - 1858; PMID: 16338413; http://dx.doi.org/10.1016/j.str.2005.08.014
- Tsodikov OV, Enzlin JH, Scharer OD, Ellenberger T. Crystal structure and DNA binding functions of ERCC1, a subunit of the DNA structure-specific endonuclease XPF-ERCC1. Proc Natl Acad Sci USA 2005; 102:11236 - 11241; PMID: 16076955; http://dx.doi.org/10.1073/pnas.0504341102
- Bastin-Shanower SA, Fricke WM, Mullen JR, Brill SJ. The mechanism of Mus81-Mms4 cleavage site selection distinguishes it from the homologous endonuclease Rad1–Rad10. Mol Cell Biol 2003; 23:3487 - 3496; PMID: 12724407; http://dx.doi.org/10.1128/MCB.23.10.3487-96.2003
- Ciccia A, Constantinou A, West SC. Identification and characterization of the human mus81-eme1 endonuclease. J Biol Chem 2003; 278:25172 - 25178; PMID: 12721304; http://dx.doi.org/10.1074/jbc.M302882200
- Ciccia A, Ling C, Coulthard R, Yan Z, Xue Y, Meetei AR, et al. Identification of FAAP24, a Fanconi anemia core complex protein that interacts with FANCM. Mol Cell 2007; 25:331 - 343; PMID: 17289582; http://dx.doi.org/10.1016/j.molcel.2007.01.003
- Fricke WM, Brill SJ. Slx1–Slx4 is a second structure-specific endonuclease functionally redundant with Sgs1-Top3. Genes Dev 2003; 17:1768 - 1778; PMID: 12832395; http://dx.doi.org/10.1101/gad.1105203
- Coulon S, Gaillard PH, Chahwan C, McDonald WH, Yates JR 3rd, Russell P. Slx1–Slx4 are subunits of a structure-specific endonuclease that maintains ribosomal DNA in fission yeast. Mol Biol Cell 2004; 15:71 - 80; PMID: 14528010; http://dx.doi.org/10.1091/mbc.E0308-0586
- Wang AT, Sengerova B, Cattell E, Inagawa T, Hartley JM, Kiakos K, et al. Human SNM1A and XPF-ERCC1 collaborate to initiate DNA interstrand cross-link repair. Genes Dev 2011; 25:1859 - 1870; PMID: 21896658; http://dx.doi.org/10.1101/gad.15699211
- Abraham J, Lemmers B, Hande MP, Moynahan ME, Chahwan C, Ciccia A, et al. Eme1 is involved in DNA damage processing and maintenance of genomic stability in mammalian cells. EMBO J 2003; 22:6137 - 6147; PMID: 14609959; http://dx.doi.org/10.1093/emboj/cdg580
- Dendouga N, Gao H, Moechars D, Janicot M, Vialard J, McGowan CH. Disruption of murine Mus81 increases genomic instability and DNA damage sensitivity but does not promote tumorigenesis. Mol Cell Biol 2005; 25:7569 - 7579; PMID: 16107704; http://dx.doi.org/10.1128/MCB.25.17.7569-79.2005
- Kumaresan KR, Hang B, Lambert MW. Human endonucleolytic incision of DNA 3′ and 5′ to a site-directed psoralen monoadduct and interstrand cross-link. J Biol Chem 1995; 270:30709 - 30716; PMID: 8530510; http://dx.doi.org/10.1074/jbc.270.51.30709
- Kumaresan KR, Hwang M, Thelen MP, Lambert MW. Contribution of XPF functional domains to the 5′ and 3′ incisions produced at the site of a psoralen interstrand cross-link. Biochemistry 2002; 41:890 - 896; PMID: 11790111; http://dx.doi.org/10.1021/bi011614z
- Kumaresan KR, Lambert MW. Fanconi anemia, complementation group A, cells are defective in ability to produce incisions at sites of psoralen inter-strand cross-links. Carcinogenesis 2000; 21:741 - 751; PMID: 10753211; http://dx.doi.org/10.1093/carcin/21.4.741
- Cannavo E, Gerrits B, Marra G, Schlapbach R, Jiricny J. Characterization of the interactome of the human MutL homologues MLH1, PMS1 and PMS2. J Biol Chem 2007; 282:2976 - 2986; PMID: 17148452; http://dx.doi.org/10.1074/jbc.M609989200
- Liu T, Ghosal G, Yuan J, Chen J, Huang J. FAN1 acts with FANCI-FANCD2 to promote DNA inter-strand cross-link repair. Science 2010; 329:693 - 696; PMID: 20671156; http://dx.doi.org/10.1126/science.1192656
- Ho TV, Scharer OD. Translesion DNA synthesis polymerases in DNA interstrand cross-link repair. Environ Mol Mutagen 2010; 51:552 - 566; PMID: 20658647
- Ho TV, Guainazzi A, Derkunt SB, Enoiu M, Scharer OD. Structure-dependent bypass of DNA interstrand cross-links by translesion synthesis polymerases. Nucleic Acids Res 2011; 39:7455 - 7464; PMID: 21666254; http://dx.doi.org/10.1093/nar/gkr448
- Minko IG, Harbut MB, Kozekov ID, Kozekova A, Jakobs PM, Olson SB, et al. Role for DNA polymerase kappa in the processing of N2-N2guanine interstrand cross-links. J Biol Chem 2008; 283:17075 - 17082; PMID: 18434313; http://dx.doi.org/10.1074/jbc.M801238200
- Henriques JA, Moustacchi E. Isolation and characterization of pso mutants sensitive to photo-addition of psoralen derivatives in Saccharomyces cerevisiae. Genetics 1980; 95:273 - 288; PMID: 7009316
- Henriques JA, Moustacchi E. Interactions between mutations for sensitivity to psoralen photoaddition (pso) and to radiation (rad) in Saccharomyces cerevisiae. J Bacteriol 1981; 148:248 - 256; PMID: 7026532
- Rahman KM, Thompson AS, James CH, Narayanaswamy M, Thurston DE. The Pyrrolobenzodiazepine dimer SJG-136 forms sequence-dependent intrastrand DNA cross-links and monoalkylated adducts in addition to interstrand cross-links. J Am Chem Soc 2009; 131:13756 - 13766; PMID: 19725510; http://dx.doi.org/10.1021/ja902986x
- Cattell E, Sengerova B, McHugh PJ. The SNM1/Pso2 family of ICL repair nucleases: from yeast to man. Environ Mol Mutagen 2010; 51:635 - 645; PMID: 20175117
- Yan Y, Akhter S, Zhang X, Legerski R. The multifunctional SNM1 gene family: not just nucleases. Future Oncol 2010; 6:1015 - 1029; PMID: 20528238; http://dx.doi.org/10.2217/fon.10.47
- Hazrati A, Ramis-Castelltort M, Sarkar S, Barber LJ, Schofield CJ, Hartley JA, et al. Human SNM1A suppresses the DNA repair defects of yeast pso2 mutants. DNA Repair (Amst) 2008; 7:230 - 238; PMID: 18006388; http://dx.doi.org/10.1016/j.dnarep.2007.09.013
- Li X, Hejna J, Moses RE. The yeast Snm1 protein is a DNA 5′-exonuclease. DNA Repair (Amst) 2005; 4:163 - 170; PMID: 15590324; http://dx.doi.org/10.1016/j.dnarep.2004.08.012
- Hejna J, Philip S, Ott J, Faulkner C, Moses R. The hSNM1 protein is a DNA 5′-exonuclease. Nucleic Acids Res 2007; 35:6115 - 6123; PMID: 17804464; http://dx.doi.org/10.1093/nar/gkm530
- Ahkter S, Richie CT, Zhang N, Behringer RR, Zhu C, Legerski RJ. Snm1-deficient mice exhibit accelerated tumorigenesis and susceptibility to infection. Mol Cell Biol 2005; 25:10071 - 10078; PMID: 16260620; http://dx.doi.org/10.1128/MCB.25.22.10071-8.2005
- Dronkert ML, de Wit J, Boeve M, Vasconcelos ML, van Steeg H, Tan TL, et al. Disruption of mouse SNM1 causes increased sensitivity to the DNA interstrand cross-linking agent mitomycin C. Mol Cell Biol 2000; 20:4553 - 4561; PMID: 10848582; http://dx.doi.org/10.1128/MCB.20.13.4553-61.2000
- Hemphill AW, Bruun D, Thrun L, Akkari Y, Torimaru Y, Hejna K, et al. Mammalian SNM1 is required for genome stability. Mol Genet Metab 2008; 94:38 - 45; PMID: 18180189; http://dx.doi.org/10.1016/j.ymgme.2007.11.012
- Ishiai M, Kimura M, Namikoshi K, Yamazoe M, Yamamoto K, Arakawa H, et al. DNA cross-link repair protein SNM1A interacts with PIAS1 in nuclear focus formation. Mol Cell Biol 2004; 24:10733 - 10741; PMID: 15572677; http://dx.doi.org/10.1128/MCB.24.24.1073341.2004
- Lenain C, Bauwens S, Amiard S, Brunori M, Giraud-Panis MJ, Gilson E. The Apollo 5′ exonuclease functions together with TRF2 to protect telomeres from DNA repair. Curr Biol 2006; 16:1303 - 1310; PMID: 16730175; http://dx.doi.org/10.1016/j.cub.2006.05.021
- Ye J, Lenain C, Bauwens S, Rizzo A, Saint-Leger A, Poulet A, et al. TRF2 and apollo cooperate with topoisomerase 2alpha to protect human telomeres from replicative damage. Cell 2010; 142:230 - 242; PMID: 20655466; http://dx.doi.org/10.1016/j.cell.2010.05.032
- Bae JB, Mukhopadhyay SS, Liu L, Zhang N, Tan J, Akhter S, et al. Snm1B/Apollo mediates replication fork collapse and S Phase checkpoint activation in response to DNA interstrand cross-links. Oncogene 2008; 27:5045 - 5056; PMID: 18469862; http://dx.doi.org/10.1038/onc.2008.139
- Demuth I, Digweed M, Concannon P. Human SNM1B is required for normal cellular response to both DNA interstrand cross-link-inducing agents and ionizing radiation. Oncogene 2004; 23:8611 - 8618; PMID: 15467758; http://dx.doi.org/10.1038/sj.onc.1207895
- Liu L, Akhter S, Bae JB, Mukhopadhyay SS, Richie CT, Liu X, et al. SNM1B/Apollo interacts with astrin and is required for the prophase cell cycle checkpoint. Cell Cycle 2009; 8:628 - 638; PMID: 19197158; http://dx.doi.org/10.4161/cc.8.4.7791
- Wang AT, McHugh PJ. Apollo: a healer of the genome?. Cell Cycle 2009; 8:1980 - 1981; PMID: 19550156; http://dx.doi.org/10.4161/cc.8.13.8977
- Anders M, Mattow J, Digweed M, Demuth I. Evidence for hSNM1B/Apollo functioning in the HSP70 mediated DNA damage response. Cell Cycle 2009; 8:1725 - 1732; PMID: 19411856; http://dx.doi.org/10.4161/cc.8.11.8605
- Demuth I, Bradshaw PS, Lindner A, Anders M, Heinrich S, Kallenbach J, et al. Endogenous hSNM1B/Apollo interacts with TRF2 and stimulates ATM in response to ionizing radiation. DNA Repair (Amst) 2008; 7:1192 - 1201; PMID: 18468965; http://dx.doi.org/10.1016/j.dnarep.2008.03.020
- Pannicke U, Ma Y, Hopfner KP, Niewolik D, Lieber MR, Schwarz K. Functional and biochemical dissection of the structure-specific nuclease ARTEMIS. EMBO J 2004; 23:1987 - 1997; PMID: 15071507; http://dx.doi.org/10.1038/sj.emboj.7600206
- Goodarzi AA, Yu Y, Riballo E, Douglas P, Walker SA, Ye R, et al. DNA-PK autophosphorylation facilitates Artemis endonuclease activity. EMBO J 2006; 25:3880 - 3889; PMID: 16874298; http://dx.doi.org/10.1038/sj.emboj.7601255
- Pawelczak KS, Turchi JJ. Purification and characterization of exonuclease-free Artemis: Implications for DNA-PK-dependent processing of DNA termini in NHEJ-catalyzed DSB repair. DNA Repair (Amst) 2010; 9:670 - 677; PMID: 20347402; http://dx.doi.org/10.1016/j.dnarep.2010.03.002
- Hosono Y, Abe T, Ishiai M, Takata M, Enomoto T, Seki M. The role of SNM1 family nucleases in etoposide-induced apoptosis. Biochem Biophys Res Commun 2011; 410:568 - 573; PMID: 21683065; http://dx.doi.org/10.1016/j.bbrc.2011.06.027
- Gregson SJ, Howard PW, Hartley JA, Brooks NA, Adams LJ, Jenkins TC, et al. Design, synthesis and evaluation of a novel pyrrolobenzodiazepine DNA-interactive agent with highly efficient cross-linking ability and potent cytotoxicity. J Med Chem 2001; 44:737 - 748; PMID: 11262084; http://dx.doi.org/10.1021/jm001064n
- Angelov T, Guainazzi A, Scharer OD. Generation of DNA interstrand cross-links by post-synthetic reductive amination. Org Lett 2009; 11:661 - 664; PMID: 19132933; http://dx.doi.org/10.1021/ol802719a
- Guainazzi A, Campbell AJ, Angelov T, Simmerling C, Scharer OD. Synthesis and molecular modeling of a nitrogen mustard DNA interstrand cross-link. Chemistry 2010; 16:12100 - 12103; PMID: 20842675; http://dx.doi.org/10.1002/chem.201002041
- Wilds CJ, Noronha AM, Robidoux S, Miller PS. Synthesis and characterization of DNA duplexes containing an N3T-ethyl-N3T interstrand cross-link in opposite orientations. Nucleosides Nucleotides Nucleic Acids 2005; 24:965 - 969; PMID: 16248073; http://dx.doi.org/10.1081/NCN-200059329
- Bhagwat N, Olsen AL, Wang AT, Hanada K, Stuckert P, Kanaar R, et al. XPF-ERCC1 participates in the Fanconi anemia pathway of cross-link repair. Mol Cell Biol 2009; 29:6427 - 6437; PMID: 19805513; http://dx.doi.org/10.1128/MCB.00086-09
- Garcia-Higuera I, Taniguchi T, Ganesan S, Meyn MS, Timmers C, Hejna J, et al. Interaction of the Fanconi anemia proteins and BRCA1 in a common pathway. Mol Cell 2001; 7:249 - 262; PMID: 11239454; http://dx.doi.org/10.1016/S1097-2765(01)00173-3
- Joo W, Xu G, Persky NS, Smogorzewska A, Rudge DG, Buzovetsky O, et al. Structure of the FANCI-FANCD2 complex: insights into the Fanconi anemia DNA repair pathway. Science 2011; 333:312 - 316; PMID: 21764741; http://dx.doi.org/10.1126/science.1205805
- Yang K, Moldovan GL, D'Andrea AD. RAD18-dependent recruitment of SNM1A to DNA repair complexes by a ubiquitin-binding zinc finger. J Biol Chem 2010; 285:19085 - 19091; PMID: 20385554; http://dx.doi.org/10.1074/jbc.M109.100032
- Geng L, Huntoon CJ, Karnitz LM. RAD18-mediated ubiquitination of PCNA activates the Fanconi anemia DNA repair network. J Cell Biol 2010; 191:249 - 257; PMID: 20937699; http://dx.doi.org/10.1083/jcb.201005101
- Park HK, Wang H, Zhang J, Datta S, Fei P. Convergence of Rad6/Rad18 and Fanconi anemia tumor suppressor pathways upon DNA damage. PLoS ONE 2010; 5:13313; PMID: 20967207; http://dx.doi.org/10.1371/journal.pone.0013313
- Song IY, Palle K, Gurkar A, Tateishi S, Kupfer GM, Vaziri C. Rad18-mediated translesion synthesis of bulky DNA adducts is coupled to activation of the Fanconi anemia DNA repair pathway. J Biol Chem 2010; 285:31525 - 31536; PMID: 20675655; http://dx.doi.org/10.1074/jbc.M110.138206
- Williams SA, Longerich S, Sung P, Vaziri C, Kupfer GM. The E3 ubiquitin ligase RAD18 regulates ubiquitylation and chromatin loading of FANCD2 and FANCI. Blood 2011; 117:5078 - 5087; PMID: 21355096; http://dx.doi.org/10.1182/blood-2010-10-311761
- Shen X, Do H, Li Y, Chung WH, Tomasz M, de Winter JP, et al. Recruitment of fanconi anemia and breast cancer proteins to DNA damage sites is differentially governed by replication. Mol Cell 2009; 35:716 - 723; PMID: 19748364; http://dx.doi.org/10.1016/j.molcel.2009.06.034