Abstract
Centrosomal proteins intricately regulate diverse microtubule-mediated cellular activities, including cell polarization and migration. However, the direct participation of these proteins in angiogenesis, which involves vascular endothelial cell migration from preexisting blood vessels, remains elusive. Here we show that the centrosomal protein Cep70 is necessary for angiogenic response in mice. This protein is also required for tube formation and capillary sprouting in vitro from vascular endothelial cells. Wound healing and transwell assays reveal that Cep70 plays a significant role in endothelial cell migration. Depletion of Cep70 results in severe defects in membrane ruffling and centrosome reorientation, indicating a requirement for this protein in cell polarization. In addition, Cep70 is critically involved in microtubule rearrangement in response to the migratory stimulus. Our data further demonstrate that Cep70 is important for Cdc42 and Rac1 activation to promote angiogenesis. These findings thus establish Cep70 as a crucial regulator of the angiogenic process and emphasize the significance of microtubule rearrangement and cell polarization and migration in angiogenesis.
Introduction
The centrosome functions as the major microtubule organizing center in animal cells and offers geometrical precision in the planar orientation of cell division by orchestrating the mitotic spindle machinery.Citation1-Citation3 In addition, there is a growing appreciation of the centrosome as a functional hub for exquisite coordination of signaling events related to cell polarization and migration.Citation1,Citation4 In vascular endothelial cells, centrosome reorientation in the intended migratory direction is an early event in the molecular cascade essential for the establishment of the neovasculature.Citation5,Citation6 In particular, centrosome reorientation favors vesicle transport toward the leading edge by positioning the Golgi apparatus between the nucleus and the leading edge, which, in cooperation with the cytoskeleton, sets the stage for vascular proliferation and sprouting during the angiogenic process.Citation7-Citation9
Angiogenesis is tightly orchestrated by a sensitive interplay of growth factors and inhibitors and has been implicated in both physiological and pathological conditions, including embryonic development, wound healing, tissue regeneration and tumor growth.Citation10-Citation14 Angiogenic sprouting encompasses the migration, proliferation, differentiation and morphogenesis of vascular endothelial cells from pre-existing blood vessels.Citation6,Citation11,Citation15 Upon activation by angiogenic factors, vascular endothelial cells produce matrix metalloproteases to degrade the basement membrane of preexisting vessels. This facilitates the migration of endothelial cells into the surrounding matrix, where they proliferate and differentiate to new lumen-containing blood vessels.Citation6,Citation11 These newly formed vessels then deposit vascular basement membrane and recruit pericytes and smooth muscle cells.
Although a vast knowledge pool exists on the role of centrosomal proteins in microtubule organization,Citation10,Citation16 their implication in angiogenesis has received much less attention. Recently, the centrosomal protein ninein has been reported to be localized in the cytoplasm of endothelial cells and highly expressed in the vasculature of normal and pathological human tissues.Citation17,Citation18 Interestingly, ninein expression has been observed in developing blood vessels, suggesting its role in capillary tube morphogenesis.Citation18 Another recent report suggests a crosstalk between the angiogenic circuitry and the centrosome duplication cycle in endothelial cells via vascular endothelial growth factor signaling.Citation19 In this study, we have uncovered an angiogenic role of Cep70, a centrosomal protein.Citation20 We demonstrate that Cep70 promotes angiogenesis by regulating microtubule rearrangement and stimulating cell polarization and migration.
Results
Cep70 is required for angiogenesis in mice.
To characterize the involvement of Cep70 in angiogenesis, we performed a directed in vivo angiogenesis assay in mice. Semiclosed angioreactors filled with basement membrane extract in the presence or absence of angiogenic factors were implanted subcutaneously into the dorsal flank of 6- to 8-week-old athymic nude mice. After implantation for 15 d, angioreactors were removed and photographed. We did not observe obvious vascular growth in angioreactors lacking angiogenic factors (, negative control). In contrast, the blood vasculature was prominent upon addition of angiogenic factors (, positive control). We then analyzed the effect of Cep70 depletion on vascular growth in angioreactors. We used two different siRNAs against mouse Cep70, and both of them could effectively decrease Cep70 expression in primary mouse embryonic fibroblasts (). Strikingly, siRNA-mediated knockdown of mouse Cep70 expression significantly suppressed vascular growth in the angioreactors, whereas control siRNA did not have obvious effect ().
Figure 1. Cep70 is required for angiogenesis in mice. (A) Semiclosed angioreactors were implanted subcutaneously into the dorsal flank of athymic nude mice, and after 15 d the angioreactors were removed and photographed (bar indicates 200 μm). Angioreactors filled with basement membrane extract containing heparin/FGF2 were used as the positive control, and angioreactors filled with basement membrane extract not containing heparin/FGF2 were used as the negative control. (B) Immunoblot analysis of Cep70 and actin expression in primary mouse embryonic fibroblasts transfected with control or two different mouse Cep70 siRNAs. (C) Vascular growth in angioreactors filled with basement membrane extract containing heparin/FGF2 and control or mouse Cep70 siRNAs (bar indicates 200 μm). (D) Immunofluorescence microscopy of blood vessels in angioreactors. Frozen sections of the vessel-containing angioreactors were immunostained with anti-CD31 and anti-desmin antibodies to detect endothelial cells (red) and mural cells (green), respectively (bar indicates 20 μm). (E) Experiments were performed as in panel D, and vascular density in angioreactors was measured by counting vessels with a layer of endothelial cells surrounded by mural cells (**p < 0.01 vs. control siRNA). (F) Hemoglobin content of blood vessels in angioreactors (*p < 0.05 vs. control siRNA). (G) Cell pellets recovered from the angioreactors were stained with fluorescein-lectin, and the level of endothelial cells was reflected by the fluorescence intensity (*p < 0.05 vs. control siRNA).
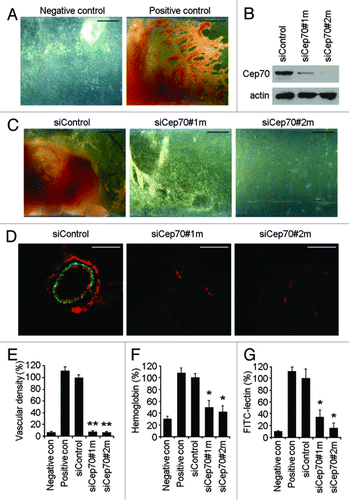
We then stained frozen sections of the vessel-containing angioreactors with anti-CD31 and anti-desmin antibodies to visualize endothelial cells and mural cells (vascular smooth muscle cells/pericytes), respectively. A number of blood vessels were detected in the control siRNA group, which displayed a layer of endothelial cells surrounded by mural cells; in contrast, blood vessels were difficult to find in the Cep70 siRNA groups (). Measurement of vascular density in angioreactors revealed that the two Cep70 siRNAs significantly inhibited angiogenesis (). To quantify the functional vasculature, we measured the hemoglobin content in angioreactors; the two Cep70 siRNAs reduced the hemoglobin level by 50% and 58%, respectively (). Collectively, these data indicate that Cep70 is critically required for angiogenic response in mice through effects on vascular endothelial cells.
We also quantified the amount of endothelial cells in angioreactors by collecting cells with dispase digestion and staining them with fluorescein-lectin, which binds specifically to endothelial cells.Citation21 As shown in , the fluorescence intensity of cells in angioreactors containing Cep70 siRNAs was remarkably reduced compared with the control siRNA group.
Depletion of Cep70 diminishes angiogenesis in vitro.
To further study the function of Cep70 in angiogenesis, we analyzed the effect of Cep70 depletion on tube formation in vitro from vascular endothelial cells. We used two different siRNAs against human Cep70, and both of them could reduce Cep70 expression in HUVECs (). After plating cells for 6 or 18 h, a tubular network of interconnecting branches was observed in the control siRNA group (); in contrast, few tubes were seen in Cep70 siRNA groups (). By calculating the cumulative tube length and the number of tube nodes, we found that knockdown of Cep70 expression significantly inhibited tube formation ().
Figure 2. Depletion of Cep70 diminishes angiogenesis in vitro. (A) Immunoblot analysis of Cep70 and actin expression in HUVECs transfected with control or two different human Cep70 siRNAs. (B) HUVECs transfected with control or Cep70 siRNAs were plated onto matrigel, and photographs were taken 6 and 18 h later (bar indicates 100 μm). (C) Experiments were performed as in panel B, and the cumulative tube length was calculated (**p < 0.01 vs. control). (D) Experiments were performed as in panel B, and the number of tube nodes was examined (**p < 0.01 vs. control). (E) Capillary-like sprouting from spheroids generated from HUVECs transfected with control or Cep70 siRNAs (bar indicates 50 μm). (F) Experiments were performed as in panel E, and the cumulative sprout length was calculated (**p < 0.01 vs. control). (G) Experiments were performed as in panel E, and the number of sprouts was examined (**p < 0.01 vs. control).
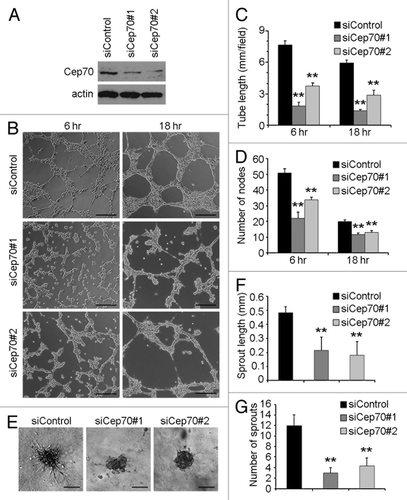
To better examine the effect of Cep70 on angiogenesis in vitro, we conducted a collagen-based three-dimensional angiogenesis assay. As shown in , Cep70 siRNAs significantly blocked endothelial sprouting from the spheroids in comparison with the control siRNA group in terms of sprout length and the number of sprouts per spheroid. These data thus demonstrate that Cep70 is important for angiogenesis in vitro.
Cep70 is critical for vascular endothelial cell migration.
We then sought to investigate the mechanisms underlying the role of Cep70 in angiogenesis. We first assessed the effect of Cep70 on vascular endothelial cell migration, a critical step in the angiogenic process. In vitro wound-healing assay revealed that knockdown of Cep70 expression in HUVECs remarkably inhibited the extent of wound closure (). Transwell cell migration assay showed that knockdown of Cep70 expression dramatically suppressed the migration of HUVECs through the porous membrane ().
Figure 3. Cep70 is critical for vascular endothelial cell migration. (A) HUVECs transfected with control or Cep70 siRNAs were scratched, and wound margins were photographed 0 and 12 h later (bar indicates 100 μm). (B) Experiments were performed as in panel A, and the extent of wound closure was quantified by measuring the wound area compared with the initial wound area (**p < 0.01 vs. control). (C) HUVECs transfected with control or Cep70 siRNAs were plated on the inside of the transwell insert, and after 18 h, cells migrated to the underside of the insert were stained with crystal violet solution (bar indicates 50 μm). (D) Experiments were performed as in panel C, and the number of migrated cells was examined. (E) Immunoblot analysis of Cep70 and actin expression in HUVECs transfected with pSIREN-RetroQ or pSIREN-RetroQ-Cep70 plasmids. (F) HUVECs were transfected with pEGFPC1 and pSIREN-RetroQ or pSIREN-RetroQ-Cep70 plasmids, and the fluorescence of GFP at the leading edge of cells was recorded at 20 sec intervals. Rectangular regions were selected as indicated to analyze membrane ruffle dynamics (bar indicates 10 μm). (G) Experiments were performed as in panel F, and membrane ruffle dynamics were presented as 3-dimensional surface plots.
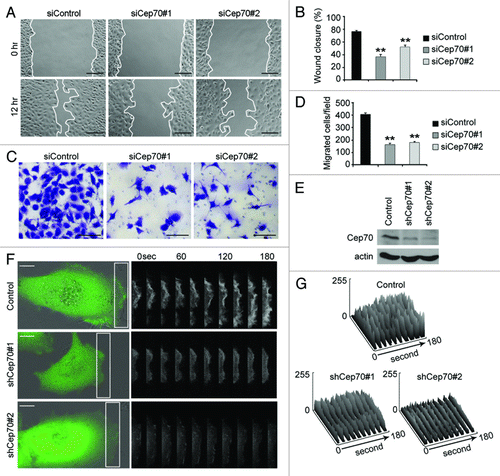
To better understand the involvement of Cep70 in cell migration, we investigated membrane ruffle dynamics at the leading edge of migrating HUVECs. We constructed two pSIREN-RetroQ-Cep70 plasmids, which express small hairpin RNAs targeting Cep70, and both plasmids could reduce Cep70 expression in HUVECs (). HUVECs were transfected with pEGFPC1 and pSIREN-RetroQ-Cep70 plasmids, and GFP fluorescence at the leading edge was recorded at 20 sec intervals (). We transformed the sequential fluorescence images to three-dimensional surface plots indicating dynamic changes in GFP fluorescence. As shown in , inhibition of Cep70 expression remarkably suppressed the dynamic changes in GFP fluorescence at the leading edge of migrating HUVECs, suggesting compromised membrane ruffling. Together, these results reveal that Cep70 is important for the migration of vascular endothelial cells.
Cep70 mediates the polarization of vascular endothelial cells.
To provide mechanistic insight into the function of Cep70, we examined the formation of membrane ruffles in migrating cells. HUVECs were transfected with pEGFPC1 and pSIREN-RetroQ-Cep70 plasmids, and the percentage of cells with membrane ruffles at the leading edge was quantified. As shown in , knockdown of Cep70 expression affected membrane ruffle formation in HUVECs. In cells with membrane ruffles in the leading edge, the number of ruffles was significantly decreased by depletion of Cep70 (). These data indicate that Cep70 plays a role in cell polarization.
Figure 4. Cep70 mediates the polarization of vascular endothelial cells. (A) HUVECs were transfected with pEGFPC1 and pSIREN-RetroQ or pSIREN-RetroQ-Cep70 plasmids, and the percentage of cells with membrane ruffles at the leading edge was examined. (B) Experiments were performed as in panel A, and the percentages of cells with 1–2, 3–4, or > 4 membrane ruffles at the leading edge were examined. (C) HUVECs transfected with control or Cep70 siRNAs were scratched, and cells were fixed 3 h later and stained with anti-α-tubulin antibody, anti-pericentrin antibody, and DAPI to visualize microtubules (green), centrosomes (red), and nuclei (blue), respectively. Broken white lines indicate the wound direction (bar indicates 10 μm). (D) Experiments were performed as in panel C, and the percentage of polarized cells at the wound margin was quantified (**p < 0.01 vs. control). (E) Experiments were performed as in panel C, and the intensity of leading-edge microtubules was quantified (**p < 0.01 vs. control). (F) Experiments were performed as in panel C, and the position of the nucleus and the centrosome relative to the cell centroid was quantified. (Bottom panel) A model shows the position of the nucleus and the centrosome in cells transfected with control or Cep70 siRNAs.
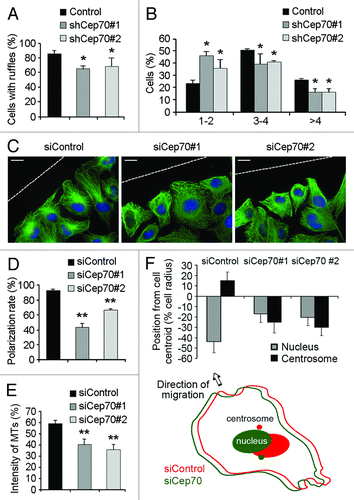
To further examine the involvement of Cep70 in cell polarization, HUVECs transfected with control or Cep70 siRNAs were scratched, and cells were fixed 3 h later and immunostained to visualize microtubules, centrosomes and nuclei. In the control siRNA group, cells at the wound margin demonstrated a typical polarized structure, with the centrosome positioned between the nucleus and the leading edge (). In contrast, the polarized morphology was impaired in cells transfected with Cep70 siRNAs (). By quantifying the percentage of polarized cells at the wound margin and the intensity of leading-edge microtubules, we found that knockdown of Cep70 expression significantly inhibited cell polarization ().
We also examined the position of the nucleus and the centrosome relative to the centroid of migrating HUVECs. As shown in , in the control siRNA group, the centrosome was located at a frontward position from the cell centroid, and the nucleus was at a rearward position. In contrast, in cells transfected with Cep70 siRNAs, both the nucleus and the cenrosome were located at the rearward position from the cell centroid (). Collectively, these data demonstrate that Cep70 mediates the polarization of vascular endothelial cells.
Cep70 regulates microtubule rearrangement in migrating cells.
We then investigated whether Cep70 has a regulatory role in microtubule rearrangement, which is critical for cell polarization and migration.Citation22-Citation24 To test this possibility, HUVECs transfected with control or Cep70 siRNAs were scratched, and 2 h later, cells were treated with nocodazole to depolymerize microtubules. Nocodazole was then washed out, and microtubule regrowth in migrating cells was examined by immunofluorescence microscopy. Microtubule asters were evident 1 min after nocodazole removal; interestingly, the asters were smaller in cells transfected with Cep70 siRNAs compared with the control siRNA group (). The importance of Cep70 in microtubule regrowth in migrating cells was also reflected by decreased microtubule intensity in Cep70 siRNA groups ().
Figure 5. Cep70 regulates microtubule rearrangement in migrating cells. (A-B) HUVECs transfected with control or Cep70 siRNAs were scratched, and 2 h later cells were treated with nocodazole to depolymerize microtubules. Nocodazole was then washed out and microtubule regrowth was examined 1 min (A) or 5 min (B) later, by immunofluorescent staining with anti-α-tubulin antibody and DAPI (bar indicates 10 μm). (C-D) Experiments were performed as in panels A and B, respectively, and the intensity of microtubules was quantified (*p < 0.05 vs. control; **p < 0.01 vs. control). (E) Experiments were performed as in panel B, and the percentage of cells with organized microtubules was examined (**p < 0.01 vs. control).
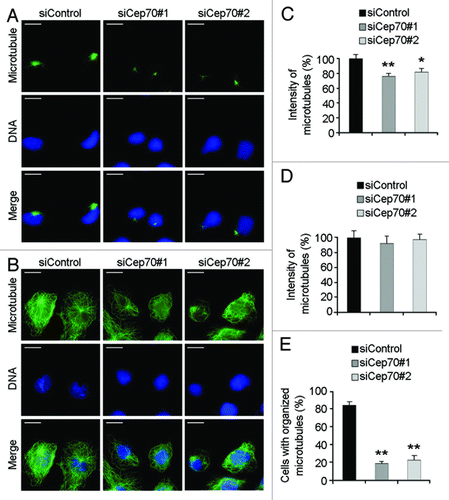
Microtubule regrowth was also examined 5 min after nocodazole removal. Knockdown of Cep70 expression did not have obvious effect on the amount of cellular microtubules (). Strikingly, while cells in the control siRNA group exhibited well-organized microtubule networks with radial arrays, the majority of cells of the Cep70 siRNA groups had disorganized microtubules (). Taken together, these findings suggest that Cep70 regulates microtubule rearrangement in migrating cells.
Cdc42 and Rac1 activation contribute to the role of Cep70 in angiogenesis.
We then sought to gain more mechanistic insight into how Cep70 regulates endothelial cell polarization and migration. The Rho family GTPases RhoA, Rac1 and Cdc42 are known as key components of the signaling pathways that control cell migration; in particular, these GTPases play a central role in orchestrating signals that link the regulation of cytoskeleton dynamics to membrane extension at the leading edge, stimulating cell polarization and migration.Citation25-Citation27 To analyze whether RhoA, Rac1 and Cdc42 contribute to the action of Cep70 in endothelial cell polarization and migration, HUVECs transfected with control or Cep70 siRNAs were scratched, and the activities of these GTPases were examined 3 h later. We found that Cep70 siRNAs could significantly decrease Cdc42 and Rac1 activities, but not RhoA activity, in HUVECs compared with the control siRNA group ().
Figure 6. Cdc42 and Rac1 activation contribute to the role of Cep70 in angiogenesis. (A) HUVECs transfected with control or Cep70 siRNAs were scratched, and cells were lysed 3 h later. The levels of activated (GTP-bound) Cdc42 and Rac1 were examined by immunoblot analysis of the GST-PBD pulldown preparation with anti-Cdc42 and anti-Rac1 antibodies. The level of activated (GTP-bound) RhoA was examined by immunoblot analysis of the GST-RBD pulldown preparation with anti-RhoA antibody. The levels of total Cdc42, Rac1, RhoA and actin were examined by immunoblot analysis of cell lysates. (B) Experiments were performed as in panel A, and the relative activities of Cdc42, Rac1 and RhoA were measured by densitometric analysis of the blots (*p < 0.05 vs. control; **p < 0.01 vs. control). (C) HUVECs transfected with Cep70 siRNA and pEGFPC1-Cdc42, pEGFPC1-Rac1, pEGFPC1-RhoA or pEGFPC1 vector were plated onto matrigel, and photographs were taken 6 and 18 h later (bar indicates 100 μm). (D) Experiments were performed as in panel C, and the cumulative tube length was calculated (**p < 0.01 vs. control).
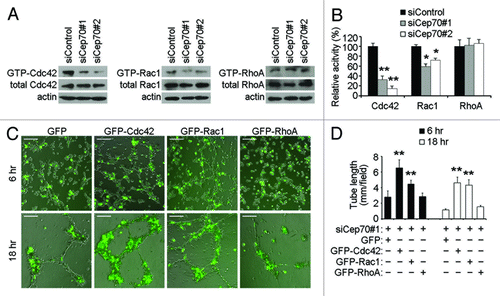
We then investigated whether Cdc42 and Rac1 are involved in the action of Cep70 in regulating angiogenesis. HUVECs were transfected with Cep70 siRNA together with GFP-tagged Cdc42, Rac1 or RhoA, and tube formation assays were performed. We found that GFP-Cdc42 and GFP-Rac1, but not GFP-RhoA, could significantly rescue the inhibitory effect of Cep70 siRNA on tube formation (). Together, these data suggest that Cep70 may play a role in the activation of Cdc42 and Rac1 in HUVECs to promote angiogenesis.
Discussion
Cep70 was originally described as a putative centrosomal protein in a mass spectrometry-based proteomic study of the human centrosome.Citation28 Our previous work has demonstrated that Cep70 interacts with ©-tubulin to localize at the centrosome and is critical for mitotic spindle assembly.Citation20 A member of the conserved Cep70 family, CRC70, has recently been shown to function as a scaffold for the centriole precursor assembly in Chlamydomonas.Citation29 In addition, Cep70 has been implicated in ciliogenesis in zebrafish embryos.Citation30 In this study, we provide several lines of evidence showing that Cep70 contributes to the angiogenic process: (1) this protein is necessary for angiogenic response in mice; (2) it mediates tube formation in vitro from vascular endothelial cells; and (3) it is required for capillary sprouting from endothelial spheroids. These findings thus establish Cep70 as a critical regulator of angiogenesis.
Our study also provides mechanistic insights into the role of Cep70 in angiogenesis. We demonstrate by in vitro wound-healing and transwell assays that Cep70 mediates the migration of vascular endothelial cells, a critical step in angiogenesis. The requirement of Cep70 for cell migration is further supported by the finding that knockdown of Cep70 expression decreases membrane ruffle dynamics at the leading edge of cells. It is noteworthy that, in addition to the centrosome, the basal bodies of cilia and flagella have been implicated in cell movement.Citation1 Considering the functions of Cep70 in cell migration and ciliogenesis and the high similarity of centrosomes and basal bodies, it will be interesting to explore whether Cep70 has a function in cells with cilia or flagella.
Efficient migration of vascular endothelial cells is known to depend on cell polarization.Citation6,Citation22,Citation31 In the present study, our data reveal that Cep70 is critical for the formation of membrane ruffles at the leading edge of cells in addition to its requirement for membrane ruffle dynamics. We also show by immunofluorescence microscopy that silencing of Cep70 expression impairs centrosome reorientation toward the leading edge of cells. These findings point out a crucial role for Cep70 in the polarization of vascular endothelial cells in response to signals that stimulate cell migration. Considering the conserved mechanisms and importance of cell polarization and migration in different cell types, it is conceivable that Cep70 might be involved in a wide spectrum of physiological and pathological processes.
The establishment of cell polarity is known to require dynamic rearrangement of the cytoskeleton; the microtubule cytoskeleton is especially known to undergo dynamic disassembly/assembly and reorganization in response to migratory cues.Citation7,Citation22,Citation32 In this context, it is easy to interpret our finding that knockdown of Cep70 expression in vascular endothelial cells significantly delays microtubule regrowth after nocodazole removal and causes disorganized microtubule network, leading to defects in cell polarization. It will be of great importance to investigate in the future how Cep70 coordinates with other centrosomal proteins to regulate microtubule organization. In addition, it will be interesting to explore how Cep70 participates in Cdc42 and Rac1 activation to promote cell migration and angiogenesis. Elucidation of these questions will undoubtedly help understand the molecular mechanisms of action of Cep70 in cell polarization, migration and angiogenesis.
Materials and Methods
Materials.
Nocodazole and 4',6-diamidino-2-phenylindole (DAPI) were purchased from Sigma-Aldrich. Antibodies against ®-actin (Sigma-Aldrich), ©-tubulin (Sigma-Aldrich), pericentrin (Covance), 〈-tubulin (Sigma-Aldrich), CD31 (Biolegend), desmin (Abcam), RhoA (Santa Cruz Biotechnology), Rac1 (Cell Signaling) and Cdc42 (Cell Signaling) were from the indicated sources. Horseradish peroxidase-conjugated goat anti-mouse and goat anti-rabbit secondary antibodies were from Santa Cruz Biotechnology. Fluorescein-conjugated donkey anti-mouse and donkey anti-rabbit secondary antibodies and rhodamine-conjugated donkey anti-mouse and donkey anti-rabbit secondary antibodies were from Jackson ImmunoResearch Laboratories. Cy3-conjugated goat anti-rat secondary antibody was from Beyotime. Human Cep70 siRNAs (siCep70#1: 5'-GAG GAU GAA UCA CUA AGU A-3'; siCep70#2: 5'-CCU CAU ACC GUC UUG GAU A-3'), mouse Cep70 siRNAs (siCep70#1m: 5'-GCA ACU CAA GGA AUC CAA A-3'; siCep70#2m: 5'-GUG UAA ACU GCA UUC GUA A-3'), and luciferase control siRNA (5'-CGU ACG CGG AAU ACU UCG A-3') were synthesized by Dharmacon.
Cell culture and transfection.
Pooled primary human umbilical vein endothelial cells (HUVECs) were obtained from the American Type Culture Collection and propagated in the accompanying endothelial cell growth medium supplemented with bovine brain extract and 10% fetal bovine serum at 37°C in a humidified atmosphere with 5% CO2. Primary mouse embryonic fibroblasts were cultured in RPMI 1640 medium supplemented with 10% fetal bovine serum at 37°C in a humidified atmosphere with 5% CO2. Plasmids were transfected into cells with the Entranster-D reagent (Engreen Biosystem), and siRNAs were transfected with the DharmaFECT1 reagent (Dharmacon).
Immunofluorescence microscopy.
HUVECs grown on glass coverslips were fixed with methanol at -20°C and blocked with 2% bovine serine albumin in phosphate-buffered saline (PBS). Cells were incubated with primary antibodies and then with fluorescein- or rhodamine-conjugated secondary antibodies, followed by staining with DAPI as described previously in references Citation33 and Citation34. Coverslips were then examined with an Axio Observer A1 fluorescence microscope (Carl Zeiss Inc.). The percentage of polarized cells and the position of the nucleus and the centrosome relative to the cell centroid were examined as described previously in references Citation22 and Citation35. For immunofluorescent staining of blood vessels, frozen sections of angioreactors were mounted onto slides precoated with 3-aminopropyltriethoxysilane. The sections were fixed with 4% paraformaldehyde, stained with anti-CD31 and anti-desmin antibodies and then examined by fluorescence microscopy.
Live cell imaging.
HUVECs were cultured in a 37°C chamber on a TCS SP5 confocal microscope (Leica) equipped with a live-cell imaging workstation. The fluorescence of GFP at the leading edge of cells was recorded at 20 sec intervals with LASAF software. The obtained image series were examined using ImageJ software (National Institutes of Health), and membrane ruffle dynamics were exhibited in three-dimensional surface plots.
Immunoblotting.
Cells were lysed in a buffer containing 1% Triton X-100, 150 mM NaCl and 50 mM Tris (pH 7.5). Proteins were resolved by SDS-PAGE and transferred onto polyvinylidene difluoride membranes (Millipore). The membranes were blocked in Tris-buffered saline containing 0.2% Tween 20 and 5% fat-free dry milk and incubated with primary antibodies and then with horseradish peroxidase-conjugated secondary antibodies as described previously in references Citation36–Citation38. Specific proteins were visualized with enhanced chemiluminescence detection reagent (Pierce Biotechnology) according to the manufacturer’s instruction.
In vivo angiogenesis assay.
The angiogenic response in mice was examined with the directed in vivo angiogenesis assay kit (Trevigen) as described previously in references Citation22 and Citation31. Briefly, semiclosed angioreactors were filled with basement membrane extract containing heparin/fibroblast growth factor 2 (FGF2) and control or mouse Cep70 siRNAs. The angioreactors were incubated at 37°C for 1 h to allow gel formation and then implanted subcutaneously into the dorsal flank of 6- to 8-week-old athymic nude mice. After 15 d, angioreactors were removed and photographed with an M165FC stereo fluorescence microscope and objective lens used was Plan-Apochromat 1.0x/0.151 NA (Leica). To quantify the amount of endothelial cells in angioreactors, cells were recovered by dispase digestion and stained with fluorescein-conjugated lectin. To quantify the functional vasculature, hemoglobin content in the angioreactors was determined by the Drabkin’s reagent (Sigma-Aldrich) as described in reference Citation39. The use of mice was approved by the Animal Care and Use Committee of Nankai University.
In vitro angiogenesis assays.
HUVECs were plated onto 24-well plates precoated with matrigel (BD Biosciences). Photographs were taken 6 and 18 h later, and the extent of tube formation was assessed by calculating the cumulative tube length and counting the number of tube nodes. In another group of experiments, endothelial cell spheroids were obtained by culturing HUVECs in medium containing 0.3% carboxymethylcellulose in round-bottom 96-well plates overnight. The spheroids were embedded into collagen gels and transferred into 24-well plates. After polymerization at 37°C for 30 min, culture medium was added on top of the collagen gel. Photographs were taken 24 h later, and the extent of capillary sprouting was evaluated by measuring the cumulative sprout length and the number of sprouts per spheroid.
In vitro wound-healing assay.
Confluent monolayers of cells cultured in 24-well plates in serum-free medium were scratched with a 10-∝L pipette tip to generate the wound. Cells were washed with PBS, and complete medium was added to allow wound healing. Phase-contrast photographs of the wound were taken 0 and 12 h later to determine the extent of wound closure.
Transwell cell migration assay.
Transwell cell migration assays were performed as described previously in reference Citation40. Briefly, HUVECs suspended in serum-free medium were added to the upper chamber of an insert (Falcon) precoated with matrigel, and the chamber was placed in a 24-well plate containing complete medium. After 18 h, cells on the inside of the transwell insert were removed with a cotton swab, and cells on the underside of the insert were fixed with 4% paraformaldehyde and stained with crystal violet solution. Three randomly selected fields were photographed, and the number of migrated cells was counted.
Measurement of Cdc42, Rac1 and RhoA activities.
The GST-PBD (p21-binding domain of Pak1) pulldown assays were used to detect cellular-activated (GTP-bound) Cdc42 and Rac1. The GST-RBD (Rho GTPase-binding domain of Rhotekin) pulldown assays were used to detect activated (GTP-bound) RhoA. In brief, cell lysates were incubated with GST-PBD or GST-RBD immobilized on glutathione sepharose beads. The beads were washed extensively, and proteins bound on beads were examined by immunoblot analysis with Cdc42, Rac1 or RhoA antibodies.
Microtubule regrowth assay.
HUVECs were treated with 10 μg/mL nocodazole for 1 h to depolymerize microtubules. Nocodazole was then washed out, and microtubule regrowth was examined 1 or 5 min later, by immunofluorescent staining with anti-〈 tubulin antibody and DAPI as described previously in reference Citation41. For better visualization of microtubules, cells were treated with a buffer containing 100 mM PIPES, 1 mM EGTA, 0.5 mM MgCl2 and 0.5% Triton X-100 (pH 6.9) before immunoflurescent staining.
Statistical analysis.
The Student t-test was used to determine statistical significance. p-values less than 0.05 were considered significant. Error bars represent standard deviation between experiments.
Abbreviations: | ||
HUVECs | = | human umbilical vein endothelial cells |
DAPI | = | 4′,6-diamidino-2-phenylindole |
PBS | = | phosphate-buffered saline |
Acknowledgements
We thank Cheng He and Xueliang Zhu for providing reagents, Jinmin Gao and Ruming Liu for technical assistance and Margaret Long for help with data analysis. This work was supported by grants from the National Basic Research Program of China (2012CB945002 and 2010CB912204) and the National Natural Science Foundation of China (30825022).
Disclosure of Potential Conflicts of Interest
No potential conflicts of interest were disclosed.
References
- Nigg EA, Raff JW. Centrioles, centrosomes, and cilia in health and disease. Cell 2009; 139:663 - 78; http://dx.doi.org/10.1016/j.cell.2009.10.036; PMID: 19914163
- Hinchcliffe EH. The centrosome and bipolar spindle assembly: does one have anything to do with the other?. Cell Cycle 2011; 10:3841 - 8; http://dx.doi.org/10.4161/cc.10.22.18293; PMID: 22071626
- Schmidt S, Essmann F, Cirtea IC, Kuck F, Thakur HC, Singh M, et al. The centrosome and mitotic spindle apparatus in cancer and senescence. Cell Cycle 2010; 9:4469 - 73; http://dx.doi.org/10.4161/cc.9.22.13684; PMID: 21088502
- Xie Z, Tsai LH. Cdk5 phosphorylation of FAK regulates centrosome-associated miocrotubules and neuronal migration. Cell Cycle 2004; 3:108 - 10; http://dx.doi.org/10.4161/cc.3.2.646; PMID: 14712065
- Hotchkiss KA, Ashton AW, Mahmood R, Russell RG, Sparano JA, Schwartz EL. Inhibition of endothelial cell function in vitro and angiogenesis in vivo by docetaxel (Taxotere): association with impaired repositioning of the microtubule organizing center. Mol Cancer Ther 2002; 1:1191 - 200; PMID: 12479700
- Lamalice L, Le Boeuf F, Huot J. Endothelial cell migration during angiogenesis. Circ Res 2007; 100:782 - 94; http://dx.doi.org/10.1161/01.RES.0000259593.07661.1e; PMID: 17395884
- Lee JS, Gotlieb AI. Microtubule-actin interactions may regulate endothelial integrity and repair. Cardiovasc Pathol 2002; 11:135 - 40; http://dx.doi.org/10.1016/S1054-8807(01)00080-1; PMID: 12031763
- Bornens M. Organelle positioning and cell polarity. Nat Rev Mol Cell Biol 2008; 9:874 - 86; http://dx.doi.org/10.1038/nrm2524; PMID: 18946476
- Sütterlin C, Colanzi A. The Golgi and the centrosome: building a functional partnership. J Cell Biol 2010; 188:621 - 8; http://dx.doi.org/10.1083/jcb.200910001; PMID: 20212314
- Carmeliet P. Angiogenesis in life, disease and medicine. Nature 2005; 438:932 - 6; http://dx.doi.org/10.1038/nature04478; PMID: 16355210
- Angiogenesis FJ. Annu Rev Med 2006; 57:1 - 18; http://dx.doi.org/10.1146/annurev.med.57.121304.131306; PMID: 16409133
- Rouhi P, Lee SL, Cao Z, Hedlund EM, Jensen LD, Cao Y. Pathological angiogenesis facilitates tumor cell dissemination and metastasis. Cell Cycle 2010; 9:913 - 7; http://dx.doi.org/10.4161/cc.9.5.10853; PMID: 20160500
- Naumov GN, Akslen LA, Folkman J. Role of angiogenesis in human tumor dormancy: animal models of the angiogenic switch. Cell Cycle 2006; 5:1779 - 87; http://dx.doi.org/10.4161/cc.5.16.3018; PMID: 16931911
- Kaur S, Roberts DD. CD47 applies the brakes to angiogenesis via vascular endothelial growth factor receptor-2. Cell Cycle 2011; 10:10 - 2; http://dx.doi.org/10.4161/cc.10.1.14324; PMID: 21191182
- Cheong A, Wood IC, Beech DJ. Less REST, more vascular disease? Regulation of cell cycle and migration of vascular smooth muscle cells. Cell Cycle 2006; 5:129 - 31; http://dx.doi.org/10.4161/cc.5.2.2310; PMID: 16357530
- Krämer A, Lukas J, Bartek J. Checking out the centrosome. Cell Cycle 2004; 3:1390 - 3; http://dx.doi.org/10.4161/cc.3.11.1252; PMID: 15483402
- Stillwell EE, Zhou J, Joshi HC. Human ninein is a centrosomal autoantigen recognized by CREST patient sera and plays a regulatory role in microtubule nucleation. Cell Cycle 2004; 3:923 - 30; http://dx.doi.org/10.4161/cc.3.7.947; PMID: 15190203
- Matsumoto T, Schiller P, Dieterich LC, Bahram F, Iribe Y, Hellman U, et al. Ninein is expressed in the cytoplasm of angiogenic tip-cells and regulates tubular morphogenesis of endothelial cells. Arterioscler Thromb Vasc Biol 2008; 28:2123 - 30; http://dx.doi.org/10.1161/ATVBAHA.108.169128; PMID: 18772498
- Taylor SM, Nevis KR, Park HL, Rogers GC, Rogers SL, Cook JG, et al. Angiogenic factor signaling regulates centrosome duplication in endothelial cells of developing blood vessels. Blood 2010; 116:3108 - 17; http://dx.doi.org/10.1182/blood-2010-01-266197; PMID: 20664058
- Shi X, Sun X, Liu M, Li D, Aneja R, Zhou J. CEP70 protein interacts with γ-tubulin to localize at the centrosome and is critical for mitotic spindle assembly. J Biol Chem 2011; 286:33401 - 8; http://dx.doi.org/10.1074/jbc.M111.252262; PMID: 21795687
- Roussel F, Dalion J. Lectins as markers of endothelial cells: comparative study between human and animal cells. Lab Anim 1988; 22:135 - 40; http://dx.doi.org/10.1258/002367788780864457; PMID: 3392947
- Gao J, Sun L, Huo L, Liu M, Li D, Zhou J. CYLD regulates angiogenesis by mediating vascular endothelial cell migration. Blood 2010; 115:4130 - 7; http://dx.doi.org/10.1182/blood-2009-10-248526; PMID: 20194890
- Watanabe T, Noritake J, Kaibuchi K. Regulation of microtubules in cell migration. Trends Cell Biol 2005; 15:76 - 83; http://dx.doi.org/10.1016/j.tcb.2004.12.006; PMID: 15695094
- Sun X, Shi X, Liu M, Li D, Zhang L, Liu X, et al. Mdp3 is a novel microtubule-binding protein that regulates microtubule assembly and stability. Cell Cycle 2011; 10:3929 - 37; http://dx.doi.org/10.4161/cc.10.22.18106; PMID: 22142902
- Raftopoulou M, Hall A. Cell migration: Rho GTPases lead the way. Dev Biol 2004; 265:23 - 32; http://dx.doi.org/10.1016/j.ydbio.2003.06.003; PMID: 14697350
- Charest PG, Firtel RA. Big roles for small GTPases in the control of directed cell movement. Biochem J 2007; 401:377 - 90; http://dx.doi.org/10.1042/BJ20061432; PMID: 17173542
- Narumiya S, Oceguera-Yanez F, Yasuda S. A new look at Rho GTPases in cell cycle: role in kinetochore-microtubule attachment. Cell Cycle 2004; 3:855 - 7; http://dx.doi.org/10.4161/cc.3.7.990; PMID: 15190208
- Andersen JS, Wilkinson CJ, Mayor T, Mortensen P, Nigg EA, Mann M. Proteomic characterization of the human centrosome by protein correlation profiling. Nature 2003; 426:570 - 4; http://dx.doi.org/10.1038/nature02166; PMID: 14654843
- Shiratsuchi G, Kamiya R, Hirono M. Scaffolding function of the Chlamydomonas procentriole protein CRC70, a member of the conserved Cep70 family. J Cell Sci 2011; 124:2964 - 75; http://dx.doi.org/10.1242/jcs.084715; PMID: 21878503
- Wilkinson CJ, Carl M, Harris WA. Cep70 and Cep131 contribute to ciliogenesis in zebrafish embryos. BMC Cell Biol 2009; 10:17; http://dx.doi.org/10.1186/1471-2121-10-17; PMID: 19254375
- Li D, Xie S, Ren Y, Huo L, Gao J, Cui D, et al. Microtubule-associated deacetylase HDAC6 promotes angiogenesis by regulating cell migration in an EB1-dependent manner. Protein Cell 2011; 2:150 - 60; http://dx.doi.org/10.1007/s13238-011-1015-4; PMID: 21359602
- Gotlieb AI. The endothelial cytoskeleton: organization in normal and regenerating endothelium. Toxicol Pathol 1990; 18:603 - 17; PMID: 2091238
- Huo L, Li D, Sun L, Liu M, Shi X, Sun X, et al. Tat acetylation regulates its actions on microtubule dynamics and apoptosis in T lymphocytes. J Pathol 2011; 223:28 - 36; http://dx.doi.org/10.1002/path.2768; PMID: 20821734
- Huo L, Li D, Sun X, Shi X, Karna P, Yang W, et al. Regulation of Tat acetylation and transactivation activity by the microtubule-associated deacetylase HDAC6. J Biol Chem 2011; 286:9280 - 6; http://dx.doi.org/10.1074/jbc.M110.208884; PMID: 21220424
- Gomes ER, Jani S, Gundersen GG. Nuclear movement regulated by Cdc42, MRCK, myosin, and actin flow establishes MTOC polarization in migrating cells. Cell 2005; 121:451 - 63; http://dx.doi.org/10.1016/j.cell.2005.02.022; PMID: 15882626
- Liu M, Wang X, Yang Y, Li D, Ren H, Zhu Q, et al. Ectopic expression of the microtubule-dependent motor protein Eg5 promotes pancreatic tumourigenesis. J Pathol 2010; 221:221 - 8; http://dx.doi.org/10.1002/path.2706; PMID: 20455257
- Sun L, Gao J, Dong X, Liu M, Li D, Shi X, et al. EB1 promotes Aurora-B kinase activity through blocking its inactivation by protein phosphatase 2A. Proc Natl Acad Sci U S A 2008; 105:7153 - 8; http://dx.doi.org/10.1073/pnas.0710018105; PMID: 18477699
- Dong X, Liu F, Sun L, Liu M, Li D, Su D, et al. Oncogenic function of microtubule end-binding protein 1 in breast cancer. J Pathol 2010; 220:361 - 9; PMID: 19967727
- Kundumani-Sridharan V, Niu J, Wang D, Van Quyen D, Zhang Q, Singh NK, et al. 15(S)-hydroxyeicosatetraenoic acid-induced angiogenesis requires Src-mediated Egr-1-dependent rapid induction of FGF-2 expression. Blood 2010; 115:2105 - 16; http://dx.doi.org/10.1182/blood-2009-09-241802; PMID: 20053757
- Sun XD, Shi XJ, Sun XO, Luo YG, Wu XJ, Yao CF, et al. Dimethylenastron suppresses human pancreatic cancer cell migration and invasion in vitro via allosteric inhibition of mitotic kinesin Eg5. Acta Pharmacol Sin 2011; 32:1543 - 8; http://dx.doi.org/10.1038/aps.2011.130; PMID: 21986572
- Gao J, Huo L, Sun X, Liu M, Li D, Dong JT, et al. The tumor suppressor CYLD regulates microtubule dynamics and plays a role in cell migration. J Biol Chem 2008; 283:8802 - 9; http://dx.doi.org/10.1074/jbc.M708470200; PMID: 18222923