Abstract
A variety of telomere protection programs are utilized to preserve telomere structure. However, the complex nature of telomere maintenance remains elusive. The Timeless protein associates with the replication fork and is thought to support efficient progression of the replication fork through natural impediments, including replication fork block sites. However, the mechanism by which Timeless regulates such genomic regions is not understood. Here, we report the role of Timeless in telomere length maintenance. We demonstrate that Timeless depletion leads to telomere shortening in human cells. This length maintenance is independent of telomerase, and Timeless depletion causes increased levels of DNA damage, leading to telomere aberrations. We also show that Timeless is associated with Shelterin components TRF1 and TRF2. Timeless depletion slows telomere replication in vitro, and Timeless-depleted cells fail to maintain TRF1-mediated accumulation of replisome components at telomeric regions. Furthermore, telomere replication undergoes a dramatic delay in Timeless-depleted cells. These results suggest that Timeless functions together with TRF1 to prevent fork collapse at telomere repeat DNA and ensure stable maintenance of telomere length and integrity.
Introduction
Due to the nature of conventional DNA polymerases, telomeres gradually shorten every cell division, leading to gene loss or replicative senescence.Citation1-Citation3 To circumvent this problem, eukaryotic cells developed telomere protection mechanisms. One such mechanism utilizes telomerase to extend the GT-rich telomeric repeats, which, in higher eukaryotes, are coated by the “Shelterin” protein complex that prevents telomeres from being recognized as DNA breaks.Citation4-Citation7 Shelterin components including TRF1, TRF2 and POT1 specifically bind telomeric repeat DNA sequences to localize and function at telomeres, indicating the importance of the telomeric repeats in chromosome end protection and in telomere length maintenance.Citation8-Citation12
Although telomeric repeats play critical roles in telomere protection, evidence suggests that telomeric DNA is a barrier to efficient replication fork progression. In yeast, replication forks stall at telomere repeats, suggesting inefficient replisome progression through telomeric regions.Citation13,Citation14 Moreover, deletion of the telomere-associated factor taz1+ (related to TRF1/TRF2) results in increased fork stalling and incomplete telomere replication.Citation13 In contrast, studies showed that altering TRF1 or TRF2 protein levels increases fork stalling in human cells.Citation15,Citation16 Despite contradictory results, these findings indicate that telomere sequence plays a role in generating difficult-to-replicate templates at telomeres, and that telomere-specific binding proteins are involved in proper progression of the replisome through telomeric repeats. Interestingly, we have demonstrated dramatic delays in the arrival of lagging-strand DNA polymerase (Pol δ) compared with leading-strand polymerase (Pol ε) at fission yeast telomeres.Citation17 Such uncoupling of leading- and lagging-strand synthesis at telomeres suggests that telomere protection also requires proper replication fork coordination.
To understand the mechanisms of replication fork stabilization, we previously demonstrated in fission yeast that Swi1 and Swi3 form the replication fork protection complex (FPC).Citation18 The FPC is conserved among eukaryotes and is homologous to the Timeless-Tipin complex in humans and Tof1-Csm3 in budding yeast. Studies in animal cells have demonstrated that FPC is localized at the replication fork and is involved in the stabilization of replication forks and in S-phase checkpoints.Citation19-Citation24 Genetic analyses have also suggested that FPC is involved in coordinating leading- and lagging-strand DNA synthesis.Citation25-Citation29 Therefore, it is straightforward to suggest that the action of FPC proteins becomes even more important to ensure proper DNA replication when the fork encounters difficult-to-replicate sites or programmed fork pausing sites that are scattered throughout the genome. Consistently, the FPC plays a critical role in programmed fork pausing and replication termination events near the mating-type (mat1) locus and at fork pausing sites in rDNA repeats in fission yeast.Citation18,Citation27,Citation28,Citation30,Citation31
Since telomeric DNA repeats also present challenges to proper replisome progression, we sought to characterize the role of Timeless-Tipin FPC in telomere protection and replication in human cells. We report that Timeless depletion leads to the loss of telomere DNA. We also show that Timeless-depleted cells display telomere DNA aberrations. Such telomere dysfunction appears to be caused by the inability of cells to perform efficient replication of telomere DNA when Timeless is depleted. We also report that Timeless interacts with Shelterin components, suggesting specific regulation of telomere replication by the FPC. These results suggest that Timeless regulates efficient DNA replication through telomeric repeats, which, in turn, contributes to the preservation of chromosome end structures.
Results
Timeless downregulation leads to shortened telomeres
To investigate the role of the Timeless protein in telomere length maintenance, we first assayed telomere length in Timeless-depleted HeLa human carcinoma cell line. Timeless was downregulated by an shRNA-expressing lentiviral vector. Cells were selected for vector integration and shRNA expression with antibiotics and maintained for about eight weeks for telomere length using telomere restriction fragment (TRF) Southern blotting (). As shown in (left panel), cells stably expressing Timeless shRNA had much shorter telomeres when compared with cells expressing control shRNA. In a separate experiment, we also monitored telomere length over about 8 weeks and found that Timeless-depleted cells showed clear telomere shortening, especially at day 59 as visualized by smaller TRFs (, right panel). Western blotting confirmed that the cells maintained Timeless depletion (). These results indicate that Timeless is required for telomere length maintenance in human cells.
Figure 1. Timeless depletion leads to loss of telomeric DNA. (A) Telomere restriction fragment (TRF) Southern blotting analysis in HeLa cells. HeLa cells were infected by lentivirus expressing control or Timeless shRNA and continuously cultured under selection for the indicated days. Genomic DNA was prepared, digested with RsaI and HinfI, and processed for TRF Southern blot analyses using a radiolabeled telomere-specific probe. RsaI and HinfI are frequent cutters that digest genomic DNA, but do not target telomere repeats,Citation67 generating a broad telomere hybridization signal ranging from 2 kb to 20 kb in HeLa cells. (B) Quantitative telomerase activity assay. Telomerase activity in Timeless shRNA cells is shown as a value relative to the activity in control shRNA cells. Cell line is indicated on each graph. Data are from at least three independent experiments and error bars represent standard deviations. (C) Telomere Flow-FISH assay of MCF-10A cells expressing the indicated shRNA. Six days post infection, cells were subjected to Telomere Flow-FISH using a FITC-conjugated telomere-specific probe, analyzing telomere signal in G1 cells. Error bars correspond to standard deviations obtained from three independent experiments. *The P-value determined by paired Student’s t-test is indicated. (D) Telomere restriction analysis of S. pombe cells. Genomic DNA prepared from the indicated cells was digested by ApaI and processed for Southern blot using a telomere probe. The ApaI site is located 30–40 bp away from telomeric repeat sequences of S. pombe chromosome termini,Citation74 generating a ~300 bp telomere hybridization signal in the wild-type (WT) strain. (E) western blotting analysis of Timeless (Tim) protein in HeLa and MCF-10A cells expressing the indicated shRNA are shown, confirming knockdown of Timeless protein. Tubulin was used as a loading control.
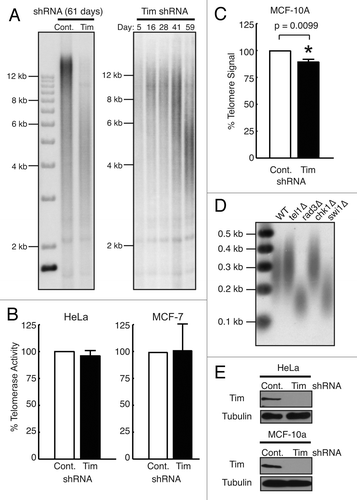
HeLa cells express telomerase.Citation32,Citation33 Therefore, we tested whether Timeless depletion affected the activity of telomerase in human cells as a possible mechanism for telomere shortening. We depleted Timeless by shRNA and determined telomerase activity using quantitative PCR detection of telomerase products in cell lysates prepared from cells expressing Timeless or control shRNA (TRAPEZE assay). Timeless shRNA had no significant effect upon telomerase activity in HeLa cells (). We also obtained similar results with MCF-7 human breast adenocarcinoma cells (), confirming that Timeless plays no significant role in the regulation of telomerase activity.
Next, we investigated whether telomere shortening occurs after Timeless depletion in the absence of immortalizing telomerase activity using the human mammary epithelial cells MCF-10A. This cell line contains no significant telomerase activity and cannot be indefinitely cultured.Citation34,Citation35 MCF-10A cells were infected with Timeless or control shRNA lentivirus. After selection, Timeless-depleted MCF-10A cells were dramatically less viable than Timeless-depleted HeLa cells and could not be cultured for long-term assessment of telomere length (data not shown). Therefore, we employed telomere fluorescent in situ hybridization (FISH) coupled with flow cytometric assessment of telomere signal (Flow-FISH) to determine the overall amount of telomere DNA on a per-cell basis in control or Timeless shRNA-expressing MCF-10A cells. Six days after infection, we observed that Timeless-depleted MCF-10A cells showed a statistically significant reduction in median telomere DNA quantity as compared with control cells, indicating that the average length of telomeres is shorter than in control cells (). Together, these results are consistent with the notion that Timeless protein has a role in human telomere maintenance that is independent of telomerase activity.
Previous reports have shown that swi1 (fission yeast Timeless) mutants exhibit shorter telomeres,Citation36 while tof1 (budding yeast Timeless) mutants show increased size heterogeneity but not significant telomere shortening.Citation37 Our investigation confirmed that, compared with wild-type cells, swi1-deleted fission yeast (swi1∆) contained much shorter telomeres, comparable to those of rad3 (ATR)-deleted cells (rad3∆) (). This result suggests an evolutionarily conserved role for Timeless-related proteins in telomere length maintenance.
Telomere specific DNA damage and telomere aberrations are both increased in Timeless-depleted cells
To further understand the role of Timeless in telomere maintenance, we performed a telomere-dysfunction induced foci (TIF) assay to examine the colocalization of the DNA repair protein 53BP1 with a telomere marker, the telomere-binding factor TRF1.Citation38,Citation39 In Timeless-depleted MCF-10A cells, 10.5% of cells had four or more foci that contained both 53BP1 and TRF1, whereas only 3.8% of control cells showed this phenotype (). These results indicate that Timeless depletion leads to telomeric DNA damage.
Figure 2. Timeless-depleted cells have telomere specific DNA damage. (A) Telomere dysfunction-induced foci (TIF) assay of MCF-10A cells expressing the indicated shRNA. Cells were in situ-extracted with Triton X-100. Colocalization of 53BP1 and TRF1 was determined using anti-53BP1 (red) and anti-TRF1 (green) antibodies. DNA was co-stained with DAPI (4’, 6’-diamidino-2-phenylindole). The merged images of 53BP1, TRF1 and DNA are shown. (B) Quantification of cells containing TIFs (colocalization of 53BP1 and TRF1). Cells with four or more TIFs were scored as TIF positive (n > 100 nuclei; Error bars correspond to standard deviations obtained from three independent experiments). *Denotes P-value determined by paired Student’s t-test.
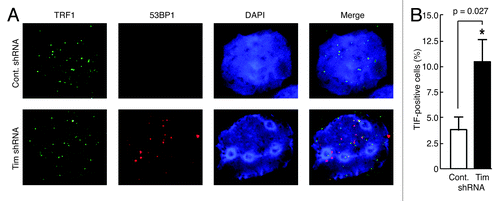
Telomeres are thought to be sites of chromosome fragility in the context of DNA replication.Citation15 Therefore, we asked whether replication stress induced by Timeless knockdown leads to an aberrant telomere phenotype. We prepared metaphase chromosome spreads from both control and Timeless shRNA-expressing HeLa cells. To evaluate their telomere structures, we performed fluorescent in situ hybridization (FISH) using a fluorophore-conjugated telomere probe (PNA probe) (). Analysis revealed that HeLa cells displayed an increase in aberrant telomere phenotypes in Timeless shRNA cells. We observed a particularly large increase in non-sister chromatid fusions and signal doublets (). The overall aberrant telomere ends increased ~71% per metaphase spread in HeLa cells when Timeless was depleted, indicating that the replication stress caused by Timeless depletion increases telomere abnormalities ().
Figure 3. Timeless-depleted cells have telomere aberrations. (A, B) Frequency of metaphase telomere phenotypes observed in HeLa (A) or MCF-10A (B) cells expressing control or Timeless shRNA. Metaphase spreads were hybridized with a telomere-PNA probe. n > 100 metaphase spreads; Error bars correspond to standard deviations obtained from three independent experiments. (C) Representative images of a normal metaphase chromosome and telomere abnormalities are shown.
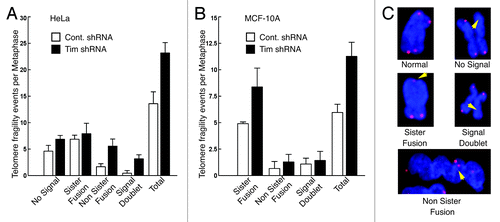
Since HeLa is a human papillomavirus transformed tumor cell line with approximately 76–80 chromosomes,Citation40 we also determined if MCF-10A cells (which possess a near normal karyotype)Citation35 induced telomere abnormalities upon Timeless loss. We utilized the same protocol for FISH analysis of metaphase chromosomes as described for HeLa cells. Hybridization with the telomere probe revealed that Timeless-depleted MCF-10A cells displayed an increase in aberrant telomere ends, similar to HeLa cells (). In particular, there was a large increase in sister chromatid fusions in Timeless-depleted cells compared with control cells (). Importantly, the overall number of chromosomes with telomere aberrations nearly doubled from six per spread in control cells to over 11 per spread after Timeless loss (). These results indicate that Timeless depletion induces telomere aberrations in human epithelial cells, regardless of karyotype or telomerase status.
Timeless interacts with Shelterin components
Aforementioned results prompted us to investigate whether Timeless interacts with telomere-specific proteins. Timeless-FLAG was expressed in 293T cells and immunoprecipitated. Endogenous TRF1 and TRF2 proteins were consistently co-precipitated with Timeless-FLAG (). We also expressed Tipin-FLAG in 293T cells and found that only small amounts of TRF1 and TRF2 were co-precipitated with Tipin (), indicating that a subset of TRF1 and TRF2 may be closely associated with Timeless but not Tipin. To further confirm the interaction of Timeless with TRF1 or TRF2, we expressed TRF1 or TRF2 as FLAG fusion proteins in 293T cells (). Endogenous Timeless was readily co-precipitated with FLAG-TRF1 and weakly with FLAG-TRF2. In addition, we obtained weak but reproducible interaction between Timeless and other shelterin components, including RAP1 and POT1 when they are expressed as FLAG-fusion proteins ().
Figure 4. Timeless interacts with TRF1 and TRF2. (A) Timeless associates with TRF1 and TRF2. 293T cells were transfected with the indicated plasmids to express Timeless or Tipin. 48 h later, immunoprecipitation (FLAG-IP) was performed on cell extracts with the anti-FLAG beads. Associated proteins were probed with anti-TRF1 and anti-TRF2 antibodies. (B) 293T cells were transfected with indicated plasmids to express Shelterin components. 48 h later, immunoprecipitation (FLAG-IP) was performed using anti-FLAG beads. Immunoprecipitates were probed with indicated antibodies to determine interaction between Timeless and Shelterin components. (C) The indicated GST-fused recombinant proteins were incubated with the Timeless protein for 45 min. GST-fusion proteins were precipitated using glutathione Sepharose beads and analyzed by western blotting using the anti-Timeless antibody (Timeless WB). Recombinant Timeless and GST-fusion proteins were stained by Colloidal blue staining. WCE, whole cell extract; FLAG-IP, immunoprecipitated fraction; WB, western blotting. Representative results of repeat experiments are shown.
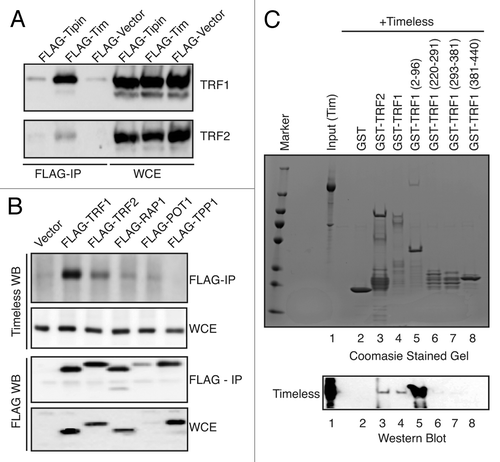
These results suggest that Timeless associates with the Shelterin complex by directly interacting with TRF1. To test this possibility, we purified recombinant TRF1 and TRF2 as GST-fusion proteins from E. coli. These proteins were incubated with recombinant Timeless protein that was purified via a baculovirus expression system. As shown in (lower panel), both GST-TRF1 and GST-TRF2 interacted directly with Timeless. To identify domains responsible for TRF1-Timeless interaction, we examined the ability of GST-fused TRF1 truncation mutants to interact with Timeless. GST-TRF1 (2–96 aa) showed strong interaction with Timeless, whereas other TRF1 truncation mutants failed to interact with Timeless, mapping the Timeless interaction domain within the N-terminal region of TRF1 (). Interestingly, this interaction was much stronger than that with full-length TRF1 (). This suggests that TRF1 contains domains that hinder TRF1-Timeless interaction, and that a conformational change of TRF1 may be required to achieve optimal interaction between Timeless and TRF1.
Timeless promotes efficient telomere replication in vitro
TRF1 has recently been shown to promote telomere replication.Citation15 Therefore, the Timeless-TRF1 interaction suggests an important role of Timeless in telomere replication. To test this possibility, we performed an in vitro telomeric DNA replication assay.Citation16,Citation41 In the presence of SV40 large T antigen in human cell extracts, canonical DNA replication occurs bidirectionally following initiation at an SV40 origin of replication.Citation42,Citation43 We utilized a plasmid (pT2AG3) containing an SV40 origin and approximately 1.8 kb of metazoan telomeric repeats as a template for in vitro replication ().Citation16 Restriction digestion of this plasmid with the BbsI enzyme generates a linear DNA molecule with the SV40 origin in the middle, telomeric repeats (Telo) at the one end and regular DNA sequences (Non-Telo) at the other end (). This system allows us to directly compare DNA replication of telomeric repeats and regular DNA in the same reaction.Citation16 Replication-competent cell extracts from control or Timeless-depleted cells were mixed with buffer containing dNTPs, the template DNA molecules described above, and the SV40 Large T antigen. The rate of DNA replication was evaluated by the incorporation radiolabeled dCTP. The replication products were purified, digested by AlwNI and HindIII enzymes to separate telomeric and regular DNA regions, run on gel electrophoresis and detected by autoradiography. As expected from previous studies,Citation16 telomere replication is less efficient compared with regular replication in control cells (control shRNA in ). As shown in , regular replication (Non-Telo) was not inhibited by Timeless downregulation. In contrast, telomere replication was largely inhibited in Timeless-depleted cells (Timeless shRNA in , Telo), suggesting that Timeless modulates the efficiency of telomere replication.
Figure 5. Timeless depletion slows replication of telomere, but not nonrepetitive DNA templates in vitro. (A) Schematic representation of the replication template, pT2AG3 plasmid linearized with BbsI enzyme. The largest fragment (right) contains ~1.8 kb of telomere repeats. (B and C) Autoradiography of radiolabeled replication products from in vitro SV40-mediated DNA replication reactions using the indicated cell extracts. Reactions were stopped at the indicated times and samples were purified, digested, and separated by agarose gel electrophoresis. In (B) no exogenous human protein added. In (C), recombinant TRF1 protein added. (D and E) Quantification of the replication products, at the indicated times, as shown above (in B and C, respectively). Top Panels are telomere DNA products, and bottom Panels are non-telomere DNA products. Representative results of repeat experiments are shown.
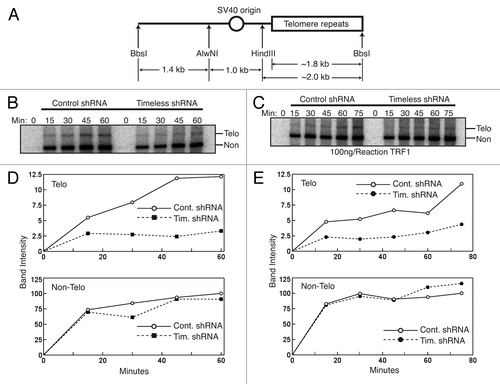
The TRF1 protein has been reported to promote telomere replication,Citation15 while overexpression of TRF1 results in replication fork stalling.Citation16 To evaluate the effect of TRF1 addition on telomere replication efficiency, we performed in vitro telomere replication assays in the presence of exogenous recombinant TRF1. Interestingly, exogenous TRF1 appeared to cause a mild decrease in the efficiency of telomere replication in control extracts compared with replication performed without exogenous TRF1 (). We also examined whether exogenous TRF1 further inhibits telomere replication in Timeless-depleted cells. Although there is no additional reduction in the efficiency of telomere replication (), our results are consistent with the notion that excess TRF1 hampers DNA replication progression through telomere repeats.
Timeless prevents TRF1-dependent accumulation of replisome components at subtelomeric repeats
While endogenous TRF1 protein appears important for replication of telomeric sequences, overexpression of TRF1 has previously been shown to induce replication fork stalling specifically at telomere repeats in vivo and in vitro.Citation16 Stalled replication forks are unstable structures, and prolonged stalling can lead to replication fork collapse, which is exacerbated in the absence of Timeless homologs.Citation24,Citation27,Citation44 To induce fork stalling at telomeres, we overexpressed TRF1 in cells stably expressing control or Timeless shRNA () and assessed whether Timeless-depleted cells have higher incidence of replication fork collapse specifically at telomeric regions. To this end, we utilized chromatin immunoprecipation and determined localization of replisome components in TRF1 overexpressing cells. Quantification of most telomere repeats by PCR is rendered inaccurate by the repetitive nature of telomeres. However, human cells contain stretches of sub-telomeric repeats bound to telomere proteins, allowing us to amplify subtelomeric DNA by PCR.Citation45 These telomeric DNA repeats bind telomere proteins and present similar challenges (aside from end-replication) to DNA replication as terminal telomere repeats. We monitored replication fork occupancy of sub-telomeric regions by Cdc45 and RPA, two proteins required for replication fork progression, which move with the replisome.Citation46,Citation47 When TRF1 was overexpressed in control shRNA cells, Cdc45 and RPA occupancy of the two distinct (TTAGGG)n-repeat containing sub-telomere regions (ST1 and ST2) increased (). These results confirmed that TRF1 overexpression causes replisome stalling at sub-telomere regions. In contrast, when Timeless was depleted, this TRF1-dependent increase in Cdc45 association was abrogated at both ST1 and ST2. Similarly, the increased occupancy of sub-telomeres by RPA was diminished in Timeless shRNA cells (). In all conditions, the percentage of cells in S-phase was not significantly altered (), indicating that TRF1 overexpression and/or Timeless depletion does not cause major defects in overall S-phase progression. Taken together with the increase in telomere aberrations in Timeless-depleted cells (), our data suggests that lower occupancy of sub-telomere repeats by the replisome is due to replication fork collapse, which is preceded by the absence of proper replication fork stalling.
Figure 6. Timeless downregulation reduces the level of replisome association at telomeres. (A) Western analysis of cells used in ChIP experiments. (B) HeLa cells expressing the indicated shRNA (C: Control; T: Timeless) were transfected with control vector (Vec), pcDNA3-TRF1-FL (TRF1). Cells were processed for ChIP using antibodies against Cdc45 or RPA. Precipitated DNA recovered from antibody-conjugated beads was used to monitor the association of Cdc45 or RPA using primers targeting different sub-telomeric (TTAGGG)n repeats (ST1 and ST2). TRF1 overexpression results in an increase in replisome association at sub-telomeres, which was abrogated by Timeless depletion. Relative DNA precipitation from each sample to control precipitation (set to 1) is shown for each experiment. Data are from at least three independent experiments, and error bars represent standard deviations. (C) Cell cycle analysis of cells used in B was performed using flow cytometry.
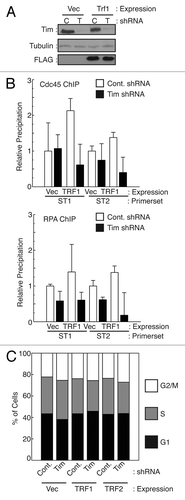
Telomere replication is dramatically delayed in the absence of Timeless
The reduction in TRF1/2-dependent replication fork stalling in Timeless-depleted cells suggests that Timeless is required for stabilization of the replisome especially at telomeres. We also showed that Timeless-depleted cell extracts have defects in efficient telomere replication in vitro (). Therefore, it is possible that an unstable replisome leads to inefficient DNA synthesis at telomeres. To test this possibility, we monitored nascent DNA synthesis throughout S-phase in human cells. Stable control and Timeless-depleted HeLa cell lines were first synchronized at the onset of S-phase using a double-thymidine block released into S-phase and collected at the indicated times. To label nascent DNA synthesis, cells were incubated with 5-ethynyl-2'-deoxyuridine (EdU) for 30 min before each time point, and EdU localization was compared with telomere FISH signal (). Colocalized EdU and telomere signals were scored as positive for telomere replication (). As a control, we monitored bulk DNA replication by measuring incorporation of bromo-deoxyuridine (BrdU) in genomic DNA (). The overall incorporation of BrdU into genomic DNA was mildly delayed in Timeless knockdown cells, consistent with the previous observation that Timeless depletion causes a delay in cell cycle progression after G1/S arrest.Citation24 The peak of DNA replication occurred at 6 h in control cells, but at 6–9 h in Timeless-depleted cells (). EdU foci colocalizing with telomere FISH signal was quantified to determine the status of telomere replication in replicating cells. Strikingly, EdU incorporation at telomere repeats was further delayed by Timeless knockdown, with a peak at 12 h (). In contrast, the peak of EdU incorporation at telomere repeats in control cells was at 6 h (). These data suggest that Timeless depletion leads to a dramatic delay in telomere replication that continues after bulk DNA synthesis is completed. Consistently, cell cycle analysis of Timeless-depleted cells showed that most cells completed S-phase by 9 h after the release from the double-thymidine block (). These results, coupled with the loss of the TRF1-dependent replisome occupancy at telomere repeats in Timeless-depleted cells (), suggest that cells experience DNA replication fork collapse in the absence of Timeless, leading to inefficient DNA replication at telomeres.
Figure 7. Telomere replication is profoundly delayed when Timeless is depleted. (A) Timeless depletion caused a profound delay in telomere replication timing. HeLa cells expressing control or Timeless shRNA were synchronized by thymidine at the beginning of S-phase and released. Cells were incubated with EdU for 30 min prior to the time point and processed for EdU detection and telomere-FISH (telomere-PNA probe). Foci containing both EdU and telomere signals were scored as telomere replication foci. Percentages of telomere replication signals over total telomere signals were obtained. Data were obtained from three independent experiments, and error bars represent standard deviations. At least 400 foci were counted for each experiment. (B) Representative image of stained cells with replicating (colocalized EdU and telomere signals) and non-replicating (not colocalized) telomere foci are shown. (C) Timeless depletion caused a mild delay in bulk BrdU incorporation. To assess the rate of DNA replication, cells used in A were also incubated with BrdU for 30 min at the indicated times after the release from a double thymidine block. Genomic DNA was fixed on nitrocellulose membrane to monitor the incorporation of BrdU by western blotting using an anti-BrdU antibody. Relative intensities of signals are shown below. Asy: Asynchronous cells. (D) Cell cycle analysis of cells used for telomere replication assays in (A and B).
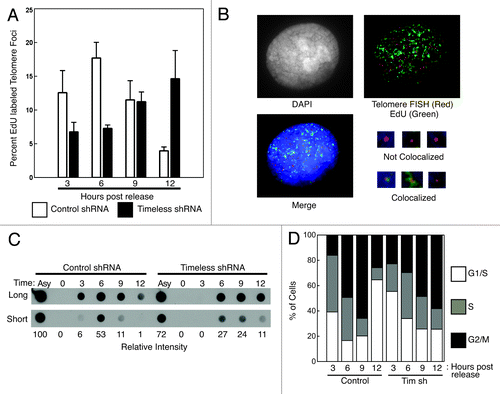
Discussion
Proper DNA replication throughout the genome is proposed to require Timeless-FPC function at the replication fork. Consistently, previous studies have shown that replication delay and DNA damage accumulation are general phenotypes associated with loss of Timeless in human cells.Citation19,Citation24,Citation48 It is also reported that Timeless and its homologs move with the replication fork as replisome components in yeast and mammalian cells.Citation18,Citation24,Citation49 However, the dramatic telomere shortening and accumulation of DNA damage at telomere suggest that Timeless plays a specific role at the telomere. Why is Timeless important for telomere replication? There are at least two challenges that may require the function of Timeless at telomeres: (1) the repetitive nature of telomeric DNA and (2) telomere-specific factors that associate with telomeres and alter their architecture.
In the first mechanism, the (TTAGGG)n repeats that stretch for kilobases at chromosome ends could present a significant challenge for DNA replication machinery. The repeats generate high-order DNA structures, including G-quadruplex and the end-capping T-loop within the telomeric repeats, which hinder replication-associated unwinding of the parental DNA strands.Citation50,Citation51 It has been reported that budding yeast tof1 (Timeless homolog) deletion mutants show increased genetic instability at trinucleotide repeats, causing elevated fork pausing and repeat expansion or contraction depending on the types of repeats.Citation52-Citation55 Therefore, it is straightforward to suggest that telomere repeats can cause similar genetic instability in the absence of Timeless, causing DNA damage and a subsequent increase in DNA repair activity at telomeres. Consistent with this idea, Timeless-depleted cells showed accumulation of DNA damage at telomeres and aberrant telomere phenotypes ( and ).
In the second mechanism, abundant telomere-binding factors, which contribute to telomere structure, pose a formidable obstacle for DNA unwinding and nascent DNA synthesis at telomeres. Indeed, Myb DNA-binding domain-containing factors such as mammalian TRF1 and S. pombe Taz1 tightly bind and bend telomeric DNA.Citation9,Citation56,Citation57 Previous in vitro experiments suggest that TRF1 and TRF2 residing on telomere repeats can lower replication efficiency,Citation16 although these factors are important for DNA replication at telomeres in vivo.Citation13,Citation15 It appears that maintaining the appropriate quantity and balance of these factors are essential to support proper telomere replication.Citation13,Citation58 Myb DNA-binding domain factors are also involved in programmed fork pausing events. In S. pombe, deletion of reb1 and rtf1 abrogates fork pausing at rDNA and mating-type switching loci, respectively.Citation59,Citation60 Importantly, S. pombe Swi1 is also required for fork pausing events at these loci, suggesting that Swi1 works with Myb-domain factors to pause and stabilize replication forks at these specific loci. Consistently, we have previously reported that swi1∆ cells show abnormal fork structures at rDNA repeats.Citation27 Therefore, the interaction between Timeless and a Myb-domain factor TRF1 may be an important regulator of telomere replication. Consistent with this model, telomere replication timing was dramatically inefficient and delayed when Timeless is downregulated ( and ). It is possible that Myb-domain factors regulate replication fork pausing at a great number of genomic loci, many of which are repetitive elements. These sites are likely difficult to replicate and thus require the function of Timeless-related proteins at the replication fork to achieve efficient DNA replication.
Current knowledge of how cells prevent the loss of DNA and minimize the need for telomere extension programs is limited. Our present results suggest that Timeless maintains telomere length by preventing replication fork collapse when the replisome encounters telomere repeats, which generates a significant barrier to normal fork progression. In this model, Timeless-depletion generates genetic instability “near” chromosome ends. In the absence of Timeless, fork collapse may results in higher recombination levels at telomeres and contraction of telomere repeats. Consistently, Timeless depletion causes an increase in the number of recombination foci on chromosomes.Citation48 Thus, we suggest that Timeless may function directly or indirectly as an anti-recombinase at the replication fork, promoting efficient DNA replication and preventing hyper-recombination at regions of repeat DNA sequences. At the telomere, this function may avert contraction of telomeric repeats, i.e., telomere shortening. Therefore, we propose that this end-replication problem is mechanistically distinct from the classic end-replication problem, where conventional DNA polymerases fail to finish replication of chromosome ends. This idea is consistent with the proposal by Miller and colleagues, in which telomere binding proteins are required at proper levels to usher the replication fork through telomere repeats, or replication problems will commence when the replisome encounters telomere repeats.Citation13,Citation61 Our results suggest that Timeless depletion generates a “near-end” problem. Similarly, genetic instability would also occur at a number of difficult-to-replicate sites throughout the chromosome, which may be prevented by Timeless; further investigation is needed to address this possibility.
Experimental Procedures
Cell culture
HeLa and 293T cells were grown as described.Citation24 MCF-10A and MCF-7 cells were grown as described.Citation62 HeLa cells were synchronized at the onset of S-phase twice in the presence of 2 mM thymidine for 15 h, with a 9-h interval of growth, and released. Cell cycle analysis was performed as described elsewhere.Citation24 Where indicated, 40 μM BrdU (Sigma) or 10 μM EdU (Invitrogen) was added to media 30 min before cells were collected.
Plasmids
Full-length cDNAs for human TRF1 and TRF2 were cloned in to the pcDNA3.1-Hygro vector, resulting in pcDNA3.1-Hygro-TRF1 and pcDNA3.1-Hygro-TRF2, respectively. Flag-tagged proteins were generated by cloning full-length cDNA for Timeless, Tipin, TRF1, TRF2, hRap1, Pot1, TPP1 into p3XFLAG-CMV24. GST fusion proteins for TRF1 and TRF2 were described previously.Citation63
Protein preparation and in vitro pull down
Purification of GST-fusion proteins and in vitro pull down experiments has been described previously.Citation63 Full-length Timeless fused to 6xHis-Flag-tag was generated in baculovirus infected cells.
Immunoprecipitation
Immunoprecipitation of FLAG-tagged proteins were performed as described with minor modifications,Citation24 using lysis buffer [20 mM TRIS-HCl, pH 7.4, 1 mM EDTA, 0.1 mM EGTA, 2 mM MgCl2, 150 mM NaCl, 1 mM Na3VO4, 1 mM NaF, 20 mM sodium glycerophosphate, 5% glycerol, 0.5% TritonX100, 0.5% Nonidet P-40, supplemented with protease inhibitors (Sigma), phosphatase inhibitors (Sigma), and 1 mM PMSF] and the anti-FLAG M2 agarose beads (Sigma). The beads were precipitated and washed, and proteins associated with the beads were analyzed by western blotting.
Antibodies
The anti-Timeless antibody was previously described.Citation24 Polyclonal antibodies to replication protein A 32kDa subunit (RPA-32) (H-100) and Cdc45 (M-300) were purchased from Santa Cruz Biotechnology; TRF1 (TRF1–78) and TRF1 (ab1423) from Abcam; 53BP1 (BP13) from Milipore; Alpha-Tubulin (B512) and FLAG-M2 from Sigma; and BrdU (7580) from Becton Dickinson. The anti-Timeless antibody (Bethyl Laboratories) and the anti-TRF1 and TRF2 antibodies (Pocono Rabbit Farms) used in were generated by immunizing rabbits with the corresponding full-length proteins purified by the baculovirus system.
shRNA infection and stable cell line generation
shRNA targeting was performed using the lentiviral vector pLKO.1-PURO encoding shRNA sequences for control knockdown (CCTAAGGTTAATGCGCCCTCGCTCTAGCGAGGGCCACTTAACCTT) or Timeless (Tim#3, GCCCACACTAACCATTGCATTCTCGAGAATGCAATGGTTAGTGTGGGCT and Tim#5, CGATACCAGATTGAGGATGATCTCGAGATCATCCTCAATCTGGTATCGT) (Open Biosystems). VSV-G-pseudotyped lentivirus was generated in 293T cells using 10 μg plasmid DNA and packaging vectors as described.Citation64 Cells were infected overnight with viral particles in culture medium containing 8 μg/ml polybrene, medium was replaced, and infected cells were selected using 2 μg/ml puromycin for at least 96 h. Stable cell lines were maintained in medium containing puromycin.
Genomic DNA preparation and restriction analysis of telomeric DNA
Genomic DNA was prepared using standard techniques.Citation65 Telomere restriction fragment length comparison was performed essentially as described.Citation66,Citation67 Briefly, 2 μg of genomic DNA was digested with HinfI and RsaI endonucleases separated by 0.6% agarose gel and processed for Southern blotting using a telomere probe (CCCTAA)5.
Telomerase activity (TRAPEZE) assay
Telomerase activity in cell proteins from HeLa and MCF-7 was determined by Telomere Repeat Amplification Protocol utilizing the TRAPEZE RT Telomerase Detection Kit (Millipore) and HS Taq DNA polymerase (Takara).
Telomere flow-FISH
Measurement of telomeric DNA amount in individual cells was performed by fluorescence in situ hybridization (FISH) coupled with cytometric analysis of FISH signal. MCF-10A cells were grown to confluency. Cell preparation, hybridization and staining with the (CCCTAA)4-FITC probe were performed as described.Citation68 Analysis was performed on a Guava Easy-Cyte Plus (Millipore). G1 cells were selected for analysis by propidium iodide staining and the telomere FITC signal was measured. The mean telomere staining intensity of Timeless knockdown cells was compared with the mean intensity of control shRNA cells, with control shRNA telomere level set to 100% for each experiment.
In vitro replication
Cell extract preparation from HeLa cells and in vitro replication experiments were performed as described.Citation16,Citation41,Citation69 SV40 large T antigen was purchases from Chimerx. pT2AG3 was linearized by BbsI digestion, purified and used as a template for replication. For experiments with TRF1 protein, 100 ng of TRF1 protein was preincubated with linear pT2AG3 in reaction buffer for 30 min prior to replication reactions.
Chromatin immunoprecipitation (ChIP)
ChIP assays were performed as described elsewhere with modifications,Citation24,Citation70 using the anti-RPA antibody, anti-Cdc45 antibody or Rabbit IgG in combination with Protein A-Sepharose. Recovered DNA was analyzed by triplicate SYBR Green-based real-time PCR (Bio-Rad) using two separate primer sets (ST1 and ST2) designed to amplify regions adjacent to subtelomeric (TTAGGG)n-like repeats as described.Citation45 Raw percent precipitated DNA values were calculated based on ∆CT between input and immunoprecipitated samples minus IgG control signal, and corrected for PCR efficiency. The value of control ChIP samples was set to 1, and relative values of experimental samples were determined.
In situ cell fractionation, immunofluorescence, chromosome spread FISH and EdU/BrdU labeling of replicating cells
In situ fractionation of cells and immunofluorescence were performed as described.Citation24,Citation71 Chromosome spreads were conducted as described.Citation24,Citation72 The chromosome spreads were then subjected to FISH with a telomere-PNA probe (DAKO) as described.Citation73 For 5-ethynyl-2'-deoxyuridine (EdU) labeling, the Click-IT Azide-488 kit (Invitrogen) was used immediately following telomere FISH and according to manufacturer’s instructions. At least 400 foci for each knockdown at each time point were counted, in triplicate. For measuring BrdU levels as a control to monitor bulk DNA replication, serial dilutions of genomic DNA were drawn onto Nitrocellulose Membranes using a Bio-Dot Apparatus (Bio-Rad). Blotted DNA was crosslinked using a XL-1000 UV Crosslinker (Spectronics). The blots were subjected to western blot analysis with antibodies against BrdU. Relative intensity of BrdU signal was determined using EZQuant software (EZ Quant).
S. pombe methods
S. pombe strains used in this study have been described for tel1 deletion (tel1∆),Citation74rad3 deletion (rad3∆), chk1 deletion (chk1∆) and swi1 deletion (swi1∆).Citation75 Southern blotting analysis of ApaI-digested telomere fragments was performed as described.Citation74
Acknowledgments
We thank Jane Clifford, Dale Haines, F. Bradley Johnson, Toru Nakamura, Mauricio Reginato and Christian Sell for comments and advice. We thank Fuyuki Ishikawa for the generous gift of pT2AG3 plasmid. Members of the Noguchi laboratory are thanked for their support and encouragement. This work was supported by National Institutes of Health (R01GM077604 to E.N., F31AG035480 to A.R.L, R01CA140652 to P.M.L. and P30CA10815 to P.M.L.) and American Heart Association (11SDG5330017 to Z.D.).
Disclosure of Potential Conflicts of Interest
No potential conflicts of interest were disclosed.
References
- Olovnikov AM. [Principle of marginotomy in template synthesis of polynucleotides]. Dokl Akad Nauk SSSR 1971; 201:1496 - 9; PMID: 5158754
- Olovnikov AM. A theory of marginotomy. The incomplete copying of template margin in enzymic synthesis of polynucleotides and biological significance of the phenomenon. J Theor Biol 1973; 41:181 - 90; http://dx.doi.org/10.1016/0022-5193(73)90198-7; PMID: 4754905
- Watson JD. Origin of concatemeric T7 DNA. Nat New Biol 1972; 239:197 - 201; PMID: 4507727
- Bryan TM, Englezou A, Gupta J, Bacchetti S, Reddel RR. Telomere elongation in immortal human cells without detectable telomerase activity. EMBO J 1995; 14:4240 - 8; PMID: 7556065
- Greider CW, Blackburn EH. The telomere terminal transferase of Tetrahymena is a ribonucleoprotein enzyme with two kinds of primer specificity. Cell 1987; 51:887 - 98; http://dx.doi.org/10.1016/0092-8674(87)90576-9; PMID: 3319189
- Greider CW, Blackburn EH. Identification of a specific telomere terminal transferase activity in Tetrahymena extracts. Cell 1985; 43:405 - 13; http://dx.doi.org/10.1016/0092-8674(85)90170-9; PMID: 3907856
- de Lange T. Shelterin: the protein complex that shapes and safeguards human telomeres. Genes Dev 2005; 19:2100 - 10; http://dx.doi.org/10.1101/gad.1346005; PMID: 16166375
- Bilaud T, Brun C, Ancelin K, Koering CE, Laroche T, Gilson E. Telomeric localization of TRF2, a novel human telobox protein. Nat Genet 1997; 17:236 - 9; http://dx.doi.org/10.1038/ng1097-236; PMID: 9326951
- Broccoli D, Smogorzewska A, Chong L, de Lange T. Human telomeres contain two distinct Myb-related proteins, TRF1 and TRF2. Nat Genet 1997; 17:231 - 5; http://dx.doi.org/10.1038/ng1097-231; PMID: 9326950
- van Steensel B, de Lange T. Control of telomere length by the human telomeric protein TRF1. Nature 1997; 385:740 - 3; http://dx.doi.org/10.1038/385740a0; PMID: 9034193
- Baumann P, Cech TR. Pot1, the putative telomere end-binding protein in fission yeast and humans. Science 2001; 292:1171 - 5; http://dx.doi.org/10.1126/science.1060036; PMID: 11349150
- Hanish JP, Yanowitz JL, de Lange T. Stringent sequence requirements for the formation of human telomeres. Proc Natl Acad Sci USA 1994; 91:8861 - 5; http://dx.doi.org/10.1073/pnas.91.19.8861; PMID: 8090736
- Miller KM, Rog O, Cooper JP. Semi-conservative DNA replication through telomeres requires Taz1. Nature 2006; 440:824 - 8; http://dx.doi.org/10.1038/nature04638; PMID: 16598261
- Makovets S, Herskowitz I, Blackburn EH. Anatomy and dynamics of DNA replication fork movement in yeast telomeric regions. Mol Cell Biol 2004; 24:4019 - 31; http://dx.doi.org/10.1128/MCB.24.9.4019-4031.2004; PMID: 15082794
- Sfeir A, Kosiyatrakul ST, Hockemeyer D, MacRae SL, Karlseder J, Schildkraut CL, et al. Mammalian telomeres resemble fragile sites and require TRF1 for efficient replication. Cell 2009; 138:90 - 103; http://dx.doi.org/10.1016/j.cell.2009.06.021; PMID: 19596237
- Ohki R, Ishikawa F. Telomere-bound TRF1 and TRF2 stall the replication fork at telomeric repeats. Nucleic Acids Res 2004; 32:1627 - 37; http://dx.doi.org/10.1093/nar/gkh309; PMID: 15007108
- Moser BA, Subramanian L, Chang Y-T, Noguchi C, Noguchi E, Nakamura TM. Differential arrival of leading and lagging strand DNA polymerases at fission yeast telomeres. EMBO J 2009; 28:810 - 20; http://dx.doi.org/10.1038/emboj.2009.31; PMID: 19214192
- Noguchi E, Noguchi C, McDonald WH, Yates JR 3rd, Russell P. Swi1 and Swi3 are components of a replication fork protection complex in fission yeast. Mol Cell Biol 2004; 24:8342 - 55; http://dx.doi.org/10.1128/MCB.24.19.8342-8355.2004; PMID: 15367656
- Chou DM, Elledge SJ. Tipin and Timeless form a mutually protective complex required for genotoxic stress resistance and checkpoint function. Proc Natl Acad Sci USA 2006; 103:18143 - 7; http://dx.doi.org/10.1073/pnas.0609251103; PMID: 17116885
- Gotter AL, Suppa C, Emanuel BS. Mammalian TIMELESS and Tipin are evolutionarily conserved replication fork-associated factors. J Mol Biol 2007; 366:36 - 52; http://dx.doi.org/10.1016/j.jmb.2006.10.097; PMID: 17141802
- Unsal-Kaçmaz K, Chastain PD, Qu P-P, Minoo P, Cordeiro-Stone M, Sancar A, et al. The human Tim/Tipin complex coordinates an Intra-S checkpoint response to UV that slows replication fork displacement. Mol Cell Biol 2007; 27:3131 - 42; http://dx.doi.org/10.1128/MCB.02190-06; PMID: 17296725
- Unsal-Kaçmaz K, Mullen TE, Kaufmann WK, Sancar A. Coupling of human circadian and cell cycles by the timeless protein. Mol Cell Biol 2005; 25:3109 - 16; http://dx.doi.org/10.1128/MCB.25.8.3109-3116.2005; PMID: 15798197
- Yoshizawa-Sugata N, Masai H. Human Tim/Timeless-interacting protein, Tipin, is required for efficient progression of S phase and DNA replication checkpoint. J Biol Chem 2007; 282:2729 - 40; http://dx.doi.org/10.1074/jbc.M605596200; PMID: 17102137
- Leman AR, Noguchi C, Lee CY, Noguchi E. Human Timeless and Tipin stabilize replication forks and facilitate sister-chromatid cohesion. J Cell Sci 2010; 123:660 - 70; http://dx.doi.org/10.1242/jcs.057984; PMID: 20124417
- Chan RC, Chan A, Jeon M, Wu TF, Pasqualone D, Rougvie AE, et al. Chromosome cohesion is regulated by a clock gene paralogue TIM-1. Nature 2003; 424:1002 - 9; http://dx.doi.org/10.1038/nature01697; PMID: 12944946
- Errico A, Costanzo V, Hunt T. Tipin is required for stalled replication forks to resume DNA replication after removal of aphidicolin in Xenopus egg extracts. Proc Natl Acad Sci USA 2007; 104:14929 - 34; http://dx.doi.org/10.1073/pnas.0706347104; PMID: 17846426
- Noguchi E, Noguchi C, Du LL, Russell P. Swi1 prevents replication fork collapse and controls checkpoint kinase Cds1. Mol Cell Biol 2003; 23:7861 - 74; http://dx.doi.org/10.1128/MCB.23.21.7861-7874.2003; PMID: 14560029
- Dalgaard JZ, Klar AJ. swi1 and swi3 perform imprinting, pausing, and termination of DNA replication in S. pombe. Cell 2000; 102:745 - 51; http://dx.doi.org/10.1016/S0092-8674(00)00063-5; PMID: 11030618
- Foss EJ. Tof1p regulates DNA damage responses during S phase in Saccharomyces cerevisiae. Genetics 2001; 157:567 - 77; PMID: 11156979
- Hodgson B, Calzada A, Labib K. Mrc1 and Tof1 regulate DNA replication forks in different ways during normal S phase. Mol Biol Cell 2007; 18:3894 - 902; http://dx.doi.org/10.1091/mbc.E07-05-0500; PMID: 17652453
- Krings G, Bastia D. swi1- and swi3-dependent and independent replication fork arrest at the ribosomal DNA of Schizosaccharomyces pombe. Proc Natl Acad Sci USA 2004; 101:14085 - 90; http://dx.doi.org/10.1073/pnas.0406037101; PMID: 15371597
- Greider CW, Blackburn EH. A telomeric sequence in the RNA of Tetrahymena telomerase required for telomere repeat synthesis. Nature 1989; 337:331 - 7; http://dx.doi.org/10.1038/337331a0; PMID: 2463488
- Morin GB. The human telomere terminal transferase enzyme is a ribonucleoprotein that synthesizes TTAGGG repeats. Cell 1989; 59:521 - 9; http://dx.doi.org/10.1016/0092-8674(89)90035-4; PMID: 2805070
- Saeki T, Takashima S, Tachibana M, Koga M, Hiyama E, Salomon DS, et al. Inhibitory effect of telomere-mimic phosphorothioate oligodeoxy nucleotides (S-ODNS) on human tumor cell lines. Oncology 1999; 57:Suppl 2 27 - 36; http://dx.doi.org/10.1159/000055272; PMID: 10545800
- Soule HD, Maloney TM, Wolman SR, Peterson WD Jr., Brenz R, McGrath CM, et al. Isolation and characterization of a spontaneously immortalized human breast epithelial cell line, MCF-10. Cancer Res 1990; 50:6075 - 86; PMID: 1975513
- Xhemalce B, Riising EM, Baumann P, Dejean A, Arcangioli BÆ, Seeler J-S. Role of SUMO in the dynamics of telomere maintenance in fission yeast. Proc Natl Acad Sci USA 2007; 104:893 - 8; http://dx.doi.org/10.1073/pnas.0605442104; PMID: 17209013
- Grandin N, Charbonneau M. Mrc1, a non-essential DNA replication protein, is required for telomere end protection following loss of capping by Cdc13, Yku or telomerase. Mol Genet Genomics 2007; 277:685 - 99; http://dx.doi.org/10.1007/s00438-007-0218-0; PMID: 17323081
- d’Adda di Fagagna F, Reaper PM, Clay-Farrace L, Fiegler H, Carr P, Von Zglinicki T, et al, Fagagna FdAd. A DNA damage checkpoint response in telomere-initiated senescence. Nature 2003; 426:194 - 8; http://dx.doi.org/10.1038/nature02118; PMID: 14608368
- Takai H, Smogorzewska A, de Lange T. DNA damage foci at dysfunctional telomeres. Curr Biol 2003; 13:1549 - 56; http://dx.doi.org/10.1016/S0960-9822(03)00542-6; PMID: 12956959
- Macville M, Schröck E, Padilla-Nash H, Keck C, Ghadimi BM, Zimonjic D, et al. Comprehensive and definitive molecular cytogenetic characterization of HeLa cells by spectral karyotyping. Cancer Res 1999; 59:141 - 50; PMID: 9892199
- Ohki R, Tsurimoto T, Ishikawa F. In vitro reconstitution of the end replication problem. Mol Cell Biol 2001; 21:5753 - 66; http://dx.doi.org/10.1128/MCB.21.17.5753-5766.2001; PMID: 11486015
- Stillman B. Chromatin assembly during SV40 DNA replication in vitro. Cell 1986; 45:555 - 65; http://dx.doi.org/10.1016/0092-8674(86)90287-4; PMID: 3011272
- Li JJ, Kelly TJ. Simian virus 40 DNA replication in vitro. Proc Natl Acad Sci USA 1984; 81:6973 - 7; http://dx.doi.org/10.1073/pnas.81.22.6973; PMID: 6095264
- McGlynn P, Lloyd RG. Recombinational repair and restart of damaged replication forks. Nat Rev Mol Cell Biol 2002; 3:859 - 70; http://dx.doi.org/10.1038/nrm951; PMID: 12415303
- Snyder AR, Zhou J, Deng Z, Lieberman PM. Therapeutic doses of hydroxyurea cause telomere dysfunction and reduce TRF2 binding to telomeres. Cancer Biol Ther 2009; 8:1136 - 45; http://dx.doi.org/10.4161/cbt.8.12.8446; PMID: 19363303
- Tercero JA, Labib K, Diffley JFX. DNA synthesis at individual replication forks requires the essential initiation factor Cdc45p. EMBO J 2000; 19:2082 - 93; http://dx.doi.org/10.1093/emboj/19.9.2082; PMID: 10790374
- Maniar HS, Wilson R, Brill SJ. Roles of replication protein-A subunits 2 and 3 in DNA replication fork movement in Saccharomyces cerevisiae. Genetics 1997; 145:891 - 902; PMID: 9093844
- Urtishak KA, Smith KD, Chanoux RA, Greenberg RA, Johnson FB, Brown EJ. Timeless Maintains Genomic Stability and Suppresses Sister Chromatid Exchange during Unperturbed DNA Replication. J Biol Chem 2009; 284:8777 - 85; http://dx.doi.org/10.1074/jbc.M806103200; PMID: 19112184
- Bando M, Katou Y, Komata M, Tanaka H, Itoh T, Sutani T, et al. Csm3, Tof1, and Mrc1 form a heterotrimeric mediator complex that associates with DNA replication forks. J Biol Chem 2009; 284:34355 - 65; http://dx.doi.org/10.1074/jbc.M109.065730; PMID: 19819872
- Chavez A, Tsou AM, Johnson FB. Telomeres do the (un)twist: helicase actions at chromosome termini. Biochim Biophys Acta 2009; 1792:329 - 40; PMID: 19245831
- Paeschke K, McDonald KR, Zakian VA. Telomeres: structures in need of unwinding. FEBS Lett 2010; 584:3760 - 72; http://dx.doi.org/10.1016/j.febslet.2010.07.007; PMID: 20637196
- Shishkin AA, Voineagu I, Matera R, Cherng N, Chernet BT, Krasilnikova MM, et al. Large-scale expansions of Friedreich’s ataxia GAA repeats in yeast. Mol Cell 2009; 35:82 - 92; http://dx.doi.org/10.1016/j.molcel.2009.06.017; PMID: 19595718
- Voineagu I, Surka CF, Shishkin AA, Krasilnikova MM, Mirkin SM. Replisome stalling and stabilization at CGG repeats, which are responsible for chromosomal fragility. Nat Struct Mol Biol 2009; 16:226 - 8; http://dx.doi.org/10.1038/nsmb.1527; PMID: 19136957
- Voineagu I, Narayanan V, Lobachev KS, Mirkin SM. Replication stalling at unstable inverted repeats: interplay between DNA hairpins and fork stabilizing proteins. Proc Natl Acad Sci USA 2008; 105:9936 - 41; http://dx.doi.org/10.1073/pnas.0804510105; PMID: 18632578
- Razidlo DF, Lahue RS. Mrc1, Tof1 and Csm3 inhibit CAG.CTG repeat instability by at least two mechanisms. DNA Repair (Amst) 2008; 7:633 - 40; http://dx.doi.org/10.1016/j.dnarep.2008.01.009; PMID: 18321795
- Bianchi A, Smith S, Chong L, Elias P, de Lange T. TRF1 is a dimer and bends telomeric DNA. EMBO J 1997; 16:1785 - 94; http://dx.doi.org/10.1093/emboj/16.7.1785; PMID: 9130722
- Cooper JP, Nimmo ER, Allshire RC, Cech TR. Regulation of telomere length and function by a Myb-domain protein in fission yeast. Nature 1997; 385:744 - 7; http://dx.doi.org/10.1038/385744a0; PMID: 9034194
- Takai KK, Hooper S, Blackwood S, Gandhi R, de Lange T. In vivo stoichiometry of shelterin components. J Biol Chem 2010; 285:1457 - 67; http://dx.doi.org/10.1074/jbc.M109.038026; PMID: 19864690
- Mejía-Ramírez E, Sánchez-Gorostiaga A, Krimer DB, Schvartzman JB, Hernández P. The mating type switch-activating protein Sap1 Is required for replication fork arrest at the rRNA genes of fission yeast. Mol Cell Biol 2005; 25:8755 - 61; http://dx.doi.org/10.1128/MCB.25.19.8755-8761.2005; PMID: 16166653
- Eydmann T, Sommariva E, Inagawa T, Mian S, Klar AJS, Dalgaard JZ. Rtf1-mediated eukaryotic site-specific replication termination. Genetics 2008; 180:27 - 39; http://dx.doi.org/10.1534/genetics.108.089243; PMID: 18723894
- Germe T, Miller K, Cooper JP. A non-canonical function of topoisomerase II in disentangling dysfunctional telomeres. EMBO J 2009; 28:2803 - 11; http://dx.doi.org/10.1038/emboj.2009.223; PMID: 19680223
- Whelan KA, Caldwell SA, Shahriari KS, Jackson SR, Franchetti LD, Johannes GJ, et al. Hypoxia suppression of Bim and Bmf blocks anoikis and luminal clearing during mammary morphogenesis. Mol Biol Cell 2010; 21:3829 - 37; http://dx.doi.org/10.1091/mbc.E10-04-0353; PMID: 20861305
- Deng Z, Norseen J, Wiedmer A, Riethman H, Lieberman PM. TERRA RNA binding to TRF2 facilitates heterochromatin formation and ORC recruitment at telomeres. Mol Cell 2009; 35:403 - 13; http://dx.doi.org/10.1016/j.molcel.2009.06.025; PMID: 19716786
- Rubinson DA, Dillon CP, Kwiatkowski AV, Sievers C, Yang L, Kopinja J, et al. A lentivirus-based system to functionally silence genes in primary mammalian cells, stem cells and transgenic mice by RNA interference. Nat Genet 2003; 33:401 - 6; http://dx.doi.org/10.1038/ng1117; PMID: 12590264
- Sambrook J, Russell DW. Molecular Cloning: A Laboratory Manual, the third edition. Cold Spring Harbor, NY: Cold Spring Harbor Laboratory Press, 2001.
- Steinert S, White DM, Zou Y, Shay JW, Wright WE. Telomere biology and cellular aging in nonhuman primate cells. Exp Cell Res 2002; 272:146 - 52; http://dx.doi.org/10.1006/excr.2001.5409; PMID: 11777339
- Herbert B-S, Shay JW, Wright WE. Analysis of Telomeres and Telomerase. John Wiley & Sons, Inc., 2001.
- Hathcock KS, Hodes RJ, Weng N-P. Analysis of Telomere Length and Telomerase Activity. In: Coligan JE, ed. Current Protocols in Immunology. New York, NY: John Wiley and Sons, Inc., 2004.
- Saharia A, Teasley DC, Duxin JP, Dao B, Chiappinelli KB, Stewart SA. FEN1 ensures telomere stability by facilitating replication fork re-initiation. J Biol Chem 2010; 285:27057 - 66; http://dx.doi.org/10.1074/jbc.M110.112276; PMID: 20551483
- Nelson JD, Denisenko O, Bomsztyk K. Protocol for the fast chromatin immunoprecipitation (ChIP) method. Nat Protoc 2006; 1:179 - 85; http://dx.doi.org/10.1038/nprot.2006.27; PMID: 17406230
- Mirzoeva OK, Petrini JH. DNA damage-dependent nuclear dynamics of the Mre11 complex. Mol Cell Biol 2001; 21:281 - 8; http://dx.doi.org/10.1128/MCB.21.1.281-288.2001; PMID: 11113202
- Henegariu O, Heerema NA, Lowe Wright L, Bray-Ward P, Ward DC, Vance GH. Improvements in cytogenetic slide preparation: controlled chromosome spreading, chemical aging and gradual denaturing. Cytometry 2001; 43:101 - 9; http://dx.doi.org/10.1002/1097-0320(20010201)43:2<101::AID-CYTO1024>3.0.CO;2-8; PMID: 11169574
- Lansdorp PM, Verwoerd NP, van de Rijke FM, Dragowska V, Little MT, Dirks RW, et al. Heterogeneity in telomere length of human chromosomes. Hum Mol Genet 1996; 5:685 - 91; http://dx.doi.org/10.1093/hmg/5.5.685; PMID: 8733138
- Nakamura TM, Moser BA, Russell P. Telomere binding of checkpoint sensor and DNA repair proteins contributes to maintenance of functional fission yeast telomeres. Genetics 2002; 161:1437 - 52; PMID: 12196391
- Ansbach AB, Noguchi C, Klansek IW, Heidlebaugh M, Nakamura TM, Noguchi E. RFCCtf18 and the Swi1-Swi3 complex function in separate and redundant pathways required for the stabilization of replication forks to facilitate sister chromatid cohesion in Schizosaccharomyces pombe. Mol Biol Cell 2008; 19:595 - 607; http://dx.doi.org/10.1091/mbc.E07-06-0618; PMID: 18045993