Abstract
The TOR (target of rapamycin) pathway is involved in aging in diverse organisms from yeast to mammals. We have previously demonstrated in human and rodent cells that mTOR converts stress-induced cell cycle arrest to irreversible senescence (geroconversion), whereas rapamycin decelerates or suppresses geroconversion during cell cycle arrest. Here, we investigated whether rapamycin can suppress replicative senescence of rodent cells. Mouse embryonic fibroblasts (MEFs) gradually acquired senescent morphology and ceased proliferation. Rapamycin decreased cellular hypertrophy, and SA-beta-Gal staining otherwise developed by 4-6 passages, but it blocked cell proliferation, masking its effects on replicative lifespan. We determined that rapamycin inhibited pS6 at 100-300 pM and inhibited proliferation with IC50 around 30 pM. At 30 pM, rapamycin partially suppressed senescence. However, the gerosuppressive effect was balanced by the cytostatic effect, making it difficult to suppress senescence without causing quiescence. We also investigated rat embryonic fibroblasts (REFs), which exhibited markers of senescence at passage 7, yet were able to slowly proliferate until 12–14 passages. REFs grew in size, acquired a large, flat cell morphology, SA-beta-Gal staining and components of DNA damage response (DDR), in particular, γH2AX/53BP1 foci. Incubation of REFs with rapamycin (from passage 7 to passage 10) allowed REFs to overcome the replicative senescence crisis. Following rapamycin treatment and removal, a fraction of proliferating REFs gradually increased and senescent phenotype disappeared completely by passage 24.
Keywords: :
Introduction
In cell culture, mammalian cells undergo permanent cell cycle arrest (replicative senescence) after a limited number of divisions.Citation1,Citation2 In human cells, progressive telomere shortening causes cell cycle arrest.Citation3 Mouse embryonic fibroblasts (MEFs) possess sufficiently long telomeres and telomerase activity.Citation4 Yet in MEFs, replicative senescence occurs after 5–10 divisions by telomere-length-independent mechanisms.Citation5 These mechanisms may involve stresses and cellular over-stimulation.Citation6 The onset of cellular senescence does not simply reflect the accumulation of cell divisions, but can be prematurely activated in response to oncogenic stimuli.Citation7,Citation8 Senescence can be accelerated by expression of Ras, MEK, AktCitation7-Citation14 as well as depletion of TSC2,Citation15,Citation16 all manipulations that activate mTOR (target of rapamycin).Citation17-Citation23
During cell cycle arrest, the mTOR pathway accelerates senescence (geroconversion). Thus, active mTOR converts cell cycle arrest caused by p21, p16 and DNA damage as well as quiescence (caused by serum starvation) to irreversible senescence.Citation24-Citation35 When the cell cycle is blocked and proliferation is impossible, then active mTOR promotes cell-size growth (hypertrophy) and cellular senescence. Rapamycin suppresses geroconversion, maintaining reversible cell cycle arrest and preventing such markers of senescence as hypertrophy, hypersecretory phenotype and pseudo-DDR.Citation28,Citation29 Also, stimulation of mTOR in normal stem cells causes hyper-proliferation, pro-gerogenic conversion and cell exhaustion.Citation36-Citation39 Not co-incidentally, the TOR pathway is involved in organismal longevity from yeast to mammals.Citation40-Citation51
It is noteworthy that inhibition of TOR decelerates chronological senescence in yeast.Citation42,Citation52-Citation55 In amazing analogy, rapamycin also inhibits “yeast-like chronological senescence” due to metabolic self-destruction in mammalian cells.Citation56-Citation58 Most importantly, inhibition of TOR decelerates replicative aging in yeast.Citation43,Citation59-Citation63 Yeast replicative lifespan is a measure of the number of divisions a mother yeast cell can undergo. When the mother cell has divided many times, it begins to enlarge, and its capacity to produce progeny decreases. Yeast replicative aging is comparable with telomere-independent replicative senescence observed in rodent cells in culture. During replicative senescence, cells become larger and eventually stop proliferating. In yeast, genetic deactivation of the TOR pathway decelerates replicative aging.Citation43 Here, we investigate whether rapamycin can decelerate replicative senescence of rodent cells in culture.
Results and Discussion
We used several preparations of MEFs from different mice. After 4–5 passages, cells become large, flat and SA-β-Gal positive. Although rapamycin does not block proliferation in most cell lines, it inhibited proliferation of MEFs cells (). We attempted to select concentrations of rapamycin that partially decrease mTOR activity without blocking proliferation. MEFs were very sensitive to rapamycin, which inhibited phosphorylation of S6 (pS6 is a standard marker of mTORC1 activity) at concentrations as low as 100–300 pM (). However, at these concentrations, rapamycin also blocked cell proliferation (). Therefore, it was impossible to dissociate effects of rapamycin on mTOR and proliferation. For further experiments, we used 30 pM rapamycin. As shown in , rapamycin prevented senescent morphology: cells remained small and mostly SA-β-Gal-negative. However, over time, MEFs were losing the sensitivity to rapamycin, requiring an increase in concentrations of rapamycin, which then blocked proliferation. This made it difficult to prevent both quiescence (when rapamycin levels were too high) and senescence (when rapamycin levels were too low).
Figure 1. Dose response to rapamycin in MEFs. (A) Immunoblot. MEFs were treated with indicated concentrations of rapamycin for 24 h and then pS6 (using 2 different antibodies) and S6 were measured by immunoblot (B) Cytostatic assay. MEFs were treated with indicated concentrations of rapamycin for 3 d and then cells were counted. Results are shown as percent of control. The effect of nutlin-3a is shown for comparison.
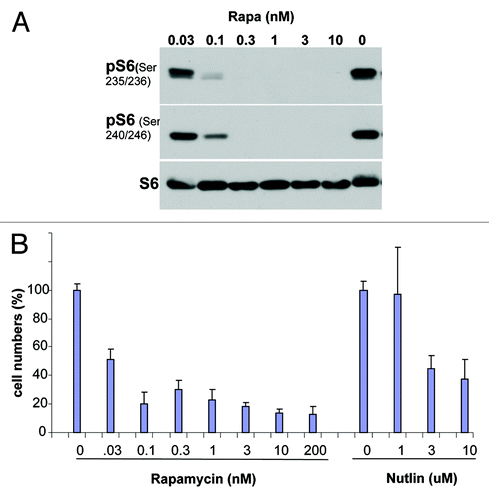
Figure 2. The effect of rapamycin on morphology of aging MEFs. Cells were cultured with (Rapa) or without (control) 30 pM rapamycin. At passages 4–5 cells were stained for SA-β-Gal and microphotographed. Scale bar = 50 μm.
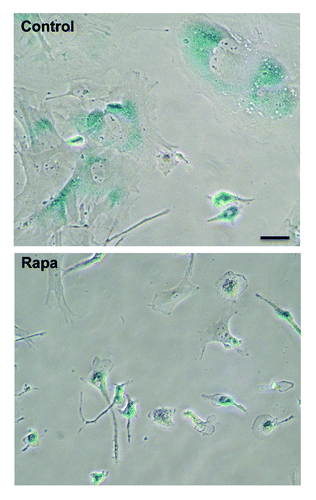
Therefore, in rat embryonic fibroblasts (REFs), we employed a different strategy: rapamycin was added when REFs were becoming senescent (passages 7–10). By passage 7, REFs became large and SA-β-Gal-positive () and also acquired markers of DNA damage response (DDR), as evidenced by γH2AX and 53BP1 (). However, DDR does not necessarily indicate actual DNA damage. In senescent and mitotic cells, DDR may be activated in the absence of DNA damage. In senescence caused by non-DNA damaging agents, DDR was partially inhibited by rapamycin.Citation29 In starved senescent cells, DDR can be induced by serum stimulation,Citation64 which is known to activate mTOR.
Figure 3. Rapamycin decelerates replicative senescence of REF cells. REFs were cultured as described in Methods. After seven passages, rapamycin was added. After three additional passages, rapamycin was removed. Left column: Cellular morphology by Romanovsky-Giemsa. Center column: SA-β-Gal staining. Right: Immunofluorescence for pS6.
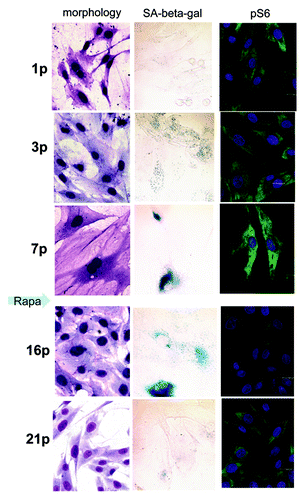
Figure 4. Immunofluorescence for γH2AX and 53BP1 in REF cells. REFs were cultured as described in Methods. After seven passages, rapamycin was added. After three additional passages, rapamycin was removed. Passage - p. REFs stained with DAPI (for DNA), for γH2AX (Ser139) and for 53BP1 at various passages (p) 1, 3, 7 before treatment with rapamycin and at passages 16 and 21 (after rapamycin treatment at p7–10). Immunofluorescence of γH2AX (red) and 53BP1 (green). Nuclei were stained with DAPI (blue), scale 20 μM.
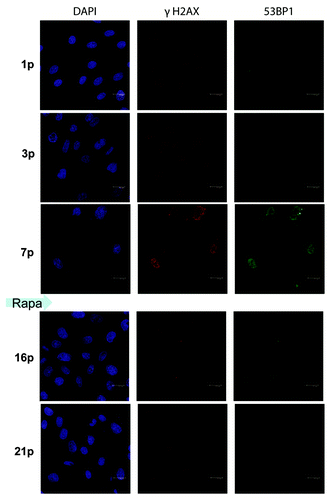
After seven passages, we added rapamycin to aging REFs. At that time point, REFs proliferated very slowly, consistent with a marked decrease in a number of cells in S phase (). Noteworthy, REFs were hypertrophic (, right part). This is consistent with a large, flat cell morphology (). Although rapamycin completely blocked the mTOR activity, as measured by pS6 immunofluorescence (), it did not completely inhibit cell proliferation. After REFs grew for three additional passages, rapamycin was removed. During further passages without rapamycin, the cells became progressively smaller (), losing DDR markers (). Furthermore, by passage 24, a fraction of S phase cells achieved the basal levels of the early passage (). The size of REFs returned to normal ( and ). Noteworthy, mTORC1 inhibition by rapamycin activated autophagy (Fig. S1).
Figure 5. Cell cycle distribution and analysis of cell size by flow cytometric light scattering. REF cells at different passages were prepared as described in Methods and analyzed for cell cycle distribution (left column) as well as cell volume by forward scattering (right column). Cells were fixed and stained with propidium iodide for DNA content (absciss axis). Forward light scattering (right column). (A) passage 1; (B) passage 7 and (C) passage 24 after rapamycin treatment during passages 7–10.
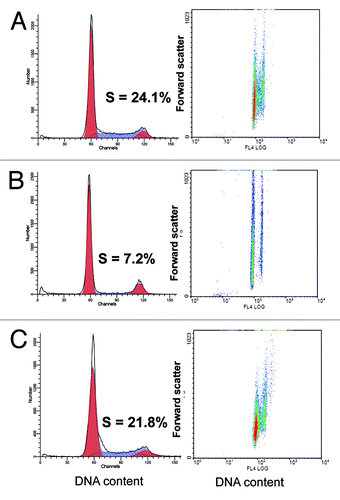
Although the mechanism of rapamycin-induced immortalization needs to be further investigated (Pospelova et al., manuscript in preparation), this may be a reminiscence of immortalization of MEFs by hypoxia. As shown by Campisi and coworkers, MEFs did not senesce in physiological (3%) oxygen levels, but underwent a spontaneous event that allowed indefinite proliferation.Citation65 Although different interpretations are possible,Citation65 we suggest that this effect may be due to inhibition of the mTOR pathway by hypoxia. In fact, hypoxia is known to inhibit the mTOR pathway.Citation66-Citation73 Also, hypoxia suppresses geroconversion during cell cycle arrest (Leontieva et al. submitted).
Finally, our data support the outstanding study by Kolesnichenko et al. By using primary human fibroblasts undergoing either replicative or oncogenic RAS-induced senescence, Kolesnichenko et al. demonstrated that senescence can be delayed, and some aspects of senescence can be reversed by inhibition of mTOR using either rapamycin or overexpression of REDD1, a negative regulator of TORC1, or by depletion of TORC1.Citation74 It is noteworthy that replicative senescence of human fibroblasts is telomere-dependent. Thus, rapamycin suppresses both types of replicative senescence in mammalian cells.
Materials and Methods
Cell culture and reagents.
Primary REF cells (second passage) were cultured in DMEM with 10% FBS (HyClone) without antibiotics in 5% CO2/95% air atmosphere. The early passage cells were split 1:3 twice a week by plating 5 x 105 cells per 60 mm dish. Mid-passage REFs (7–10 passages) were split 1:2 weekly, and late-passage REFs were split 1:2 after confluence. In this way, several subsequent passages were performed until the cells failed to undergo population doublings. Under the given conditions of cultivation, REFs usually reached this state after 14–15 passages. Primary REFs were treated with 200 nM rapamycin from 7–10 or 7–11 pp. After three passages, rapamycin was removed. Primary mouse embryonic fibroblasts (MEFs, second passage) were cultured in DMEM supplemented with 10% FBS in 5% CO2/95% air atmosphere. Cells were split 1:3–4. Stock of rapamycin (LC Laboratories) was prepared as 5 mM in DMSO. Nutlin-3a was purchased from Sigma-Aldrich and dissolved in DMSO as 10 mM stock.
Immunoblot analysis.
Whole-cell lysates were prepared using boiling lysis buffer (1% SDS, 10 mM Tris.HCl, pH 7.4). Equal amounts of proteins were separated on 10% or gradient polyacrylamide gels and transferred to nitrocellulose membranes. The following antibodies were used: rabbit anti-phospho-S6 (Ser235/236) and rabbit anti-phospho-S6 (Ser240/244) and mouse anti-S6 from Cell Signaling Biotechnology. Secondary anti-rabbit and anti-mouse HRP conjugated antibodies were from Cell Signaling Biotechnology. Signals were visualized using ECL chemilumenescence kit from Pierce.
Immunofluorescence microscopy.
REF cells were seeded and grown on coverslips, washed in PBS, fixed in fresh 4% paraformaldehyde for 15 min at room temperature and washed three times in PBS for 10 min each time. The cells were permeabilized with 0.2% Triton X-100 for 20 min, and blocked by incubation with 5% bovine serum albumin (V fraction, Sigma) in PBS for 30 min. The coverslips were incubated with the following primary antibodies: anti-S6 mouse monoclonal antibody (Cell Signaling), LC3 rabbit polyclonal (Cell Signaling) or pS6 rabbit polyclonal antibodies and rabbit policlonal anti γH2AX (Ser 139) (Cell Signaling), anti 53BP1 rabbit polyclonal (Santa Cruz) for 1 h. The coverslips were washed three times in PBS-1% BSA for 10 min each time and incubated with the secondary antibodies: rabbit anti-mouse fluorochrome-conjugated Alexa Fluor 488-conjugated or goat anti-rabbit Alexa Fluor 543-conjugated (Invitrogen) and DAPI or To-Pro III, (Molecular Probes) to stain nuclei for 1 h at room temperature. The images were obtained using Leica TCS SL confocal microscope (Leica Microsystems).
SA-β-Gal staining.
For , SA-β-Gal staining was performed using senescence-galactosidase staining kit (Cell Signaling Technology) according to manufacturer’s protocol. For , cells were fixed for 5 min in β-galactosidase fixative (2% formaldehyde; 0.2% glutaraldehyde in PBS) and washed in PBS and stained in β-galactosidase solution (1 mg/ml 5-bromo-4-chloro-3-indolyl-β-Gal (X-gal) in 5 mM potassium ferricyamide, 5 mM potassium ferrocyamide, 2 mM MgCl2 in PBS) at 37°C until β-Gal staining become visible in either experiment or control plates. Thereafter, cells were washed in PBS, and the number of β-galactosidase-positive cells (blue staining) was counted under bright field illumination.
Romanovsky-Giemsa staining.
Cells on coverslips were washed several times in PBS, fixed in cold methanol and stained for 4 min with a dye prepared from a mixture of Azur, eosin and methylene blue. After staining, slides were washed several times with tap water, dried and embedded in Canada balsam. Analysis was performed on Axioscope at magnification 40 x 10.
Flow cytometry.
Cells were trypsinized, washed twice with the phosphate buffered saline (PBS) and treated with 0.01% saponin for 30 min at room temperature. The cells were then washed with PBS and incubated with RNase A (Sigma, 0.1 mg/ml) and propidium iodide (Sigma, 50 mg/ml) for 15 min at 37°C. An analysis of cell cycle distribution was performed by using an Odam cytofluorimeter (Brucker). Cell size of control and rapamycin-treated cells were compared by means of cytometric light scatering of propidium iodide stained cells by using Win MDI program version 2.8.
Additional material
Download Zip (586.3 KB)Acknowledgments
The work was supported by Russian Fund for Basic Research (grants 10–04–01152 and 12–04–01393), Russian Academy of Sciences Program “Molecular and Cell Biology,” and grant from the St. Petersburg State University, contract #1.37.122.2011.
Disclosure of Potential Conflicts of Interest
No potential conflicts of interest were disclosed.
Note
Supplemental materials can be found at: www.landesbioscience.com/journals/cc/article/20882
References
- Shay JW, Wright WE. Hayflick, his limit, and cellular ageing. Nat Rev Mol Cell Biol 2000; 1:72 - 6; http://dx.doi.org/10.1038/35036093; PMID: 11413492
- Campisi J, Kim SH, Lim CS, Rubio M. Cellular senescence, cancer and aging: the telomere connection. Exp Gerontol 2001; 36:1619 - 37; http://dx.doi.org/10.1016/S0531-5565(01)00160-7; PMID: 11672984
- Bodnar AG, Ouellette M, Frolkis M, Holt SE, Chiu CP, Morin GB, et al. Extension of life-span by introduction of telomerase into normal human cells. Science 1998; 279:349 - 52; http://dx.doi.org/10.1126/science.279.5349.349; PMID: 9454332
- Russo I, Silver AR, Cuthbert AP, Griffin DK, Trott DA, Newbold RF. A telomere-independent senescence mechanism is the sole barrier to Syrian hamster cell immortalization. Oncogene 1998; 17:3417 - 26; http://dx.doi.org/10.1038/sj.onc.1202261; PMID: 10030665
- Itahana K, Campisi J, Dimri GP. Mechanisms of cellular senescence in human and mouse cells. Biogerontology 2004; 5:1 - 10; http://dx.doi.org/10.1023/B:BGEN.0000017682.96395.10; PMID: 15138376
- Serrano M, Blasco MA. Putting the stress on senescence. Curr Opin Cell Biol 2001; 13:748 - 53; http://dx.doi.org/10.1016/S0955-0674(00)00278-7; PMID: 11698192
- Serrano M, Lin AW, McCurrach ME, Beach D, Lowe SW. Oncogenic ras provokes premature cell senescence associated with accumulation of p53 and p16INK4a. Cell 1997; 88:593 - 602; http://dx.doi.org/10.1016/S0092-8674(00)81902-9; PMID: 9054499
- Lin AW, Barradas M, Stone JC, van Aelst L, Serrano M, Lowe SW. Premature senescence involving p53 and p16 is activated in response to constitutive MEK/MAPK mitogenic signaling. Genes Dev 1998; 12:3008 - 19; http://dx.doi.org/10.1101/gad.12.19.3008; PMID: 9765203
- Lloyd AC, Obermüller F, Staddon S, Barth CF, McMahon M, Land H. Cooperating oncogenes converge to regulate cyclin/cdk complexes. Genes Dev 1997; 11:663 - 77; http://dx.doi.org/10.1101/gad.11.5.663; PMID: 9119230
- Sewing A, Wiseman B, Lloyd AC, Land H. High-intensity Raf signal causes cell cycle arrest mediated by p21Cip1. Mol Cell Biol 1997; 17:5588 - 97; PMID: 9271434
- Woods D, Parry D, Cherwinski H, Bosch E, Lees E, McMahon M. Raf-induced proliferation or cell cycle arrest is determined by the level of Raf activity with arrest mediated by p21Cip1. Mol Cell Biol 1997; 17:5598 - 611; PMID: 9271435
- Zhu JY, Woods D, McMahon M, Bishop JM. Senescence of human fibroblasts induced by oncogenic Raf. Genes Dev 1998; 12:2997 - 3007; http://dx.doi.org/10.1101/gad.12.19.2997; PMID: 9765202
- Kerkhoff E, Rapp UR. High-intensity Raf signals convert mitotic cell cycling into cellular growth. Cancer Res 1998; 58:1636 - 40; PMID: 9563474
- Kortum RL, Johnson HJ, Costanzo DL, Volle DJ, Razidlo GL, Fusello AM, et al. The molecular scaffold kinase suppressor of Ras 1 is a modifier of RasV12-induced and replicative senescence. Mol Cell Biol 2006; 26:2202 - 14; http://dx.doi.org/10.1128/MCB.26.6.2202-2214.2006; PMID: 16507997
- Zhang H, Cicchetti G, Onda H, Koon HB, Asrican K, Bajraszewski N, et al. Loss of Tsc1/Tsc2 activates mTOR and disrupts PI3K-Akt signaling through downregulation of PDGFR. J Clin Invest 2003; 112:1223 - 33; PMID: 14561707
- Zhang H, Bajraszewski N, Wu E, Wang H, Moseman AP, Dabora SL, et al. PDGFRs are critical for PI3K/Akt activation and negatively regulated by mTOR. J Clin Invest 2007; 117:730 - 8; http://dx.doi.org/10.1172/JCI28984; PMID: 17290308
- Hay N, Sonenberg N. Upstream and downstream of mTOR. Genes Dev 2004; 18:1926 - 45; http://dx.doi.org/10.1101/gad.1212704; PMID: 15314020
- Inoki K, Corradetti MN, Guan KL. Dysregulation of the TSC-mTOR pathway in human disease. Nat Genet 2005; 37:19 - 24; http://dx.doi.org/10.1038/ng1494; PMID: 15624019
- Wullschleger S, Loewith R, Hall MN. TOR signaling in growth and metabolism. Cell 2006; 124:471 - 84; http://dx.doi.org/10.1016/j.cell.2006.01.016; PMID: 16469695
- Dann SG, Selvaraj A, Thomas G. mTOR Complex1-S6K1 signaling: at the crossroads of obesity, diabetes and cancer. Trends Mol Med 2007; 13:252 - 9; http://dx.doi.org/10.1016/j.molmed.2007.04.002; PMID: 17452018
- Dazert E, Hall MN. mTOR signaling in disease. Curr Opin Cell Biol 2011; 23:744 - 55; http://dx.doi.org/10.1016/j.ceb.2011.09.003; PMID: 21963299
- Conn CS, Qian SB. mTOR signaling in protein homeostasis: less is more?. Cell Cycle 2011; 10:1940 - 7; http://dx.doi.org/10.4161/cc.10.12.15858; PMID: 21555915
- Ma XM, Blenis J. Molecular mechanisms of mTOR-mediated translational control. Nat Rev Mol Cell Biol 2009; 10:307 - 18; http://dx.doi.org/10.1038/nrm2672; PMID: 19339977
- Demidenko ZN, Blagosklonny MV. Growth stimulation leads to cellular senescence when the cell cycle is blocked. Cell Cycle 2008; 7:3355 - 61; http://dx.doi.org/10.4161/cc.7.21.6919; PMID: 18948731
- Demidenko ZN, Zubova SG, Bukreeva EI, Pospelov VA, Pospelova TV, Blagosklonny MV. Rapamycin decelerates cellular senescence. Cell Cycle 2009; 8:1888 - 95; http://dx.doi.org/10.4161/cc.8.12.8606; PMID: 19471117
- Demidenko ZN, Shtutman M, Blagosklonny MV. Pharmacologic inhibition of MEK and PI-3K converges on the mTOR/S6 pathway to decelerate cellular senescence. Cell Cycle 2009; 8:1896 - 900; http://dx.doi.org/10.4161/cc.8.12.8809; PMID: 19478560
- Demidenko ZN, Blagosklonny MV. At concentrations that inhibit mTOR, resveratrol suppresses cellular senescence. Cell Cycle 2009; 8:1901 - 4; http://dx.doi.org/10.4161/cc.8.12.8810; PMID: 19471118
- Demidenko ZN, Blagosklonny MV. Quantifying pharmacologic suppression of cellular senescence: prevention of cellular hypertrophy versus preservation of proliferative potential. Aging 2009; 1:1008 - 16; PMID: 20157583
- Pospelova TV, Demidenko ZN, Bukreeva EI, Pospelov VA, Gudkov AV, Blagosklonny MV. Pseudo-DNA damage response in senescent cells. Cell Cycle 2009; 8:4112 - 8; http://dx.doi.org/10.4161/cc.8.24.10215; PMID: 19946210
- Romanov VS, Abramova MV, Svetlikova SB, Bykova TV, Zubova SG, Aksenov ND, et al. p21(Waf1) is required for cellular senescence but not for cell cycle arrest induced by the HDAC inhibitor sodium butyrate. Cell Cycle 2010; 9:3945 - 55; http://dx.doi.org/10.4161/cc.9.19.13160; PMID: 20935470
- Demidenko ZN, Korotchkina LG, Gudkov AV, Blagosklonny MV. Paradoxical suppression of cellular senescence by p53. Proc Natl Acad Sci USA 2010; 107:9660 - 4; http://dx.doi.org/10.1073/pnas.1002298107; PMID: 20457898
- Korotchkina LG, Leontieva OV, Bukreeva EI, Demidenko ZN, Gudkov AV, Blagosklonny MV. The choice between p53-induced senescence and quiescence is determined in part by the mTOR pathway. Aging 2010; 2:344 - 52; PMID: 20606252
- Leontieva OV, Blagosklonny MV. DNA damaging agents and p53 do not cause senescence in quiescent cells, while consecutive re-activation of mTOR is associated with conversion to senescence. Aging 2010; 2:924 - 35; PMID: 21212465
- Leontieva OV, Gudkov AV, Blagosklonny MV. Weak p53 permits senescence during cell cycle arrest. Cell Cycle 2010; 9:4323 - 7; http://dx.doi.org/10.4161/cc.9.21.13584; PMID: 21051933
- Leontieva OV, Demidenko ZN, Gudkov AV, Blagosklonny MV. Elimination of proliferating cells unmasks the shift from senescence to quiescence caused by rapamycin. PLoS ONE 2011; 6:e26126; http://dx.doi.org/10.1371/journal.pone.0026126; PMID: 22022534
- Blagosklonny MV. Aging, stem cells, and mammalian target of rapamycin: a prospect of pharmacologic rejuvenation of aging stem cells. Rejuvenation Res 2008; 11:801 - 8; http://dx.doi.org/10.1089/rej.2008.0722; PMID: 18729812
- Chen C, Liu Y, Liu Y, Zheng P. mTOR regulation and therapeutic rejuvenation of aging hematopoietic stem cells. Sci Signal 2009; 2:ra75; http://dx.doi.org/10.1126/scisignal.2000559; PMID: 19934433
- Gan B, DePinho RA. mTORC1 signaling governs hematopoietic stem cell quiescence. Cell Cycle 2009; 8:1003 - 6; http://dx.doi.org/10.4161/cc.8.7.8045; PMID: 19270523
- Castilho RM, Squarize CH, Chodosh LA, Williams BO, Gutkind JS. mTOR mediates Wnt-induced epidermal stem cell exhaustion and aging. Cell Stem Cell 2009; 5:279 - 89; http://dx.doi.org/10.1016/j.stem.2009.06.017; PMID: 19733540
- Stipp D. A new path to longevity. Sci Am 2012; 306:32 - 9; http://dx.doi.org/10.1038/scientificamerican0112-32; PMID: 22279832
- Vellai T, Takacs-Vellai K, Zhang Y, Kovacs AL, Orosz L, Müller F. Genetics: influence of TOR kinase on lifespan in C. elegans. Nature 2003; 426:620; http://dx.doi.org/10.1038/426620a; PMID: 14668850
- Powers RW 3rd, Kaeberlein M, Caldwell SD, Kennedy BK, Fields S. Extension of chronological lifespan in yeast by decreased TOR pathway signaling. Genes Dev 2006; 20:174 - 84; http://dx.doi.org/10.1101/gad.1381406; PMID: 16418483
- Kaeberlein M, Powers RW 3rd, Steffen KK, Westman EA, Hu D, Dang N, et al. Regulation of yeast replicative life span by TOR and Sch9 in response to nutrients. Science 2005; 310:1193 - 6; http://dx.doi.org/10.1126/science.1115535; PMID: 16293764
- Jia K, Chen D, Riddle DL. The TOR pathway interacts with the insulin signaling pathway to regulate C. elegans larval development, metabolism and life span. Development 2004; 131:3897 - 906; http://dx.doi.org/10.1242/dev.01255; PMID: 15253933
- Kapahi P, Zid BM, Harper T, Koslover D, Sapin V, Benzer S. Regulation of lifespan in Drosophila by modulation of genes in the TOR signaling pathway. Curr Biol 2004; 14:885 - 90; http://dx.doi.org/10.1016/j.cub.2004.03.059; PMID: 15186745
- Harrison DE, Strong R, Sharp ZD, Nelson JF, Astle CM, Flurkey K, et al. Rapamycin fed late in life extends lifespan in genetically heterogeneous mice. Nature 2009; 460:392 - 5; PMID: 19587680
- Selman C, Tullet JM, Wieser D, Irvine E, Lingard SJ, Choudhury AI, et al. Ribosomal protein S6 kinase 1 signaling regulates mammalian life span. Science 2009; 326:140 - 4; http://dx.doi.org/10.1126/science.1177221; PMID: 19797661
- Moskalev AA, Shaposhnikov MV. Pharmacological inhibition of phosphoinositide 3 and TOR kinases improves survival of Drosophila melanogaster. Rejuvenation Res 2010; 13:246 - 7; http://dx.doi.org/10.1089/rej.2009.0903; PMID: 20017609
- Bjedov I, Toivonen JM, Kerr F, Slack C, Jacobson J, Foley A, et al. Mechanisms of life span extension by rapamycin in the fruit fly Drosophila melanogaster. Cell Metab 2010; 11:35 - 46; http://dx.doi.org/10.1016/j.cmet.2009.11.010; PMID: 20074526
- Miller RA, Harrison DE, Astle CM, Baur JA, Boyd AR, de Cabo R, et al. Rapamycin, but not resveratrol or simvastatin, extends life span of genetically heterogeneous mice. J Gerontol A Biol Sci Med Sci 2011; 66:191 - 201; http://dx.doi.org/10.1093/gerona/glq178; PMID: 20974732
- Anisimov VN, Zabezhinski MA, Popovich IG, Piskunova TS, Semenchenko AV, Tyndyk ML, et al. Rapamycin increases lifespan and inhibits spontaneous tumorigenesis in inbred female mice. Cell Cycle 2011; 10:4230 - 6; http://dx.doi.org/10.4161/cc.10.24.18486; PMID: 22107964
- Bonawitz ND, Chatenay-Lapointe M, Pan Y, Shadel GS. Reduced TOR signaling extends chronological life span via increased respiration and upregulation of mitochondrial gene expression. Cell Metab 2007; 5:265 - 77; http://dx.doi.org/10.1016/j.cmet.2007.02.009; PMID: 17403371
- Alvers AL, Wood MS, Hu D, Kaywell AC, Dunn WA Jr., Aris JP. Autophagy is required for extension of yeast chronological life span by rapamycin. Autophagy 2009; 5:847 - 9; PMID: 19458476
- Pan Y, Shadel GS. Extension of chronological life span by reduced TOR signaling requires down-regulation of Sch9p and involves increased mitochondrial OXPHOS complex density. Aging 2009; 1:131 - 45; PMID: 20157595
- Pan Y, Schroeder EA, Ocampo A, Barrientos A, Shadel GS. Regulation of yeast chronological life span by TORC1 via adaptive mitochondrial ROS signaling. Cell Metab 2011; 13:668 - 78; http://dx.doi.org/10.1016/j.cmet.2011.03.018; PMID: 21641548
- Leontieva OV, Blagosklonny MV. Yeast-like chronological senescence in mammalian cells: phenomenon, mechanism and pharmacological suppression. Aging 2011; 3:1078 - 91; PMID: 22156391
- Fabrizio P, Wei M. Conserved role of medium acidification in chronological senescence of yeast and mammalian cells. Aging 2011; 3:1127 - 9; PMID: 22184281
- Kaeberlein M, Kennedy BK. A new chronological survival assay in mammalian cell culture. Cell Cycle 2012; 11:201 - 2; http://dx.doi.org/10.4161/cc.11.2.18959; PMID: 22214665
- Kaeberlein M, Hu D, Kerr EO, Tsuchiya M, Westman EA, Dang N, et al. Increased life span due to calorie restriction in respiratory-deficient yeast. PLoS Genet 2005; 1:e69; http://dx.doi.org/10.1371/journal.pgen.0010069; PMID: 16311627
- Urban J, Soulard A, Huber A, Lippman S, Mukhopadhyay D, Deloche O, et al. Sch9 is a major target of TORC1 in Saccharomyces cerevisiae. Mol Cell 2007; 26:663 - 74; http://dx.doi.org/10.1016/j.molcel.2007.04.020; PMID: 17560372
- Steffen KK, MacKay VL, Kerr EO, Tsuchiya M, Hu D, Fox LA, et al. Yeast life span extension by depletion of 60s ribosomal subunits is mediated by Gcn4. Cell 2008; 133:292 - 302; http://dx.doi.org/10.1016/j.cell.2008.02.037; PMID: 18423200
- Steinkraus KA, Kaeberlein M, Kennedy BK. Replicative aging in yeast: the means to the end. Annu Rev Cell Dev Biol 2008; 24:29 - 54; http://dx.doi.org/10.1146/annurev.cellbio.23.090506.123509; PMID: 18616424
- Ha CW, Huh WK. The implication of Sir2 in replicative aging and senescence in Saccharomyces cerevisiae. Aging 2011; 3:319 - 24; PMID: 21415463
- Satyanarayana A, Greenberg RA, Schaetzlein S, Buer J, Masutomi K, Hahn WC, et al. Mitogen stimulation cooperates with telomere shortening to activate DNA damage responses and senescence signaling. Mol Cell Biol 2004; 24:5459 - 74; http://dx.doi.org/10.1128/MCB.24.12.5459-5474.2004; PMID: 15169907
- Parrinello S, Samper E, Krtolica A, Goldstein J, Melov S, Campisi J. Oxygen sensitivity severely limits the replicative lifespan of murine fibroblasts. Nat Cell Biol 2003; 5:741 - 7; http://dx.doi.org/10.1038/ncb1024; PMID: 12855956
- Sofer A, Lei K, Johannessen CM, Ellisen LW. Regulation of mTOR and cell growth in response to energy stress by REDD1. Mol Cell Biol 2005; 25:5834 - 45; http://dx.doi.org/10.1128/MCB.25.14.5834-5845.2005; PMID: 15988001
- Arsham AM, Howell JJ, Simon MC. A novel hypoxia-inducible factor-independent hypoxic response regulating mammalian target of rapamycin and its targets. J Biol Chem 2003; 278:29655 - 60; http://dx.doi.org/10.1074/jbc.M212770200; PMID: 12777372
- Brugarolas J, Lei K, Hurley RL, Manning BD, Reiling JH, Hafen E, et al. Regulation of mTOR function in response to hypoxia by REDD1 and the TSC1/TSC2 tumor suppressor complex. Genes Dev 2004; 18:2893 - 904; http://dx.doi.org/10.1101/gad.1256804; PMID: 15545625
- Connolly E, Braunstein S, Formenti S, Schneider RJ. Hypoxia inhibits protein synthesis through a 4E-BP1 and elongation factor 2 kinase pathway controlled by mTOR and uncoupled in breast cancer cells. Mol Cell Biol 2006; 26:3955 - 65; http://dx.doi.org/10.1128/MCB.26.10.3955-3965.2006; PMID: 16648488
- Liu L, Cash TP, Jones RG, Keith B, Thompson CB, Simon MC. Hypoxia-induced energy stress regulates mRNA translation and cell growth. Mol Cell 2006; 21:521 - 31; http://dx.doi.org/10.1016/j.molcel.2006.01.010; PMID: 16483933
- DeYoung MP, Horak P, Sofer A, Sgroi D, Ellisen LW. Hypoxia regulates TSC1/2-mTOR signaling and tumor suppression through REDD1-mediated 14-3-3 shuttling. Genes Dev 2008; 22:239 - 51; http://dx.doi.org/10.1101/gad.1617608; PMID: 18198340
- Cam H, Easton JB, High A, Houghton PJ. mTORC1 signaling under hypoxic conditions is controlled by ATM-dependent phosphorylation of HIF-1α. Mol Cell 2010; 40:509 - 20; http://dx.doi.org/10.1016/j.molcel.2010.10.030; PMID: 21095582
- Wolff NC, Vega-Rubin-de-Celis S, Xie XJ, Castrillon DH, Kabbani W, Brugarolas J. Cell-type-dependent regulation of mTORC1 by REDD1 and the tumor suppressors TSC1/TSC2 and LKB1 in response to hypoxia. Mol Cell Biol 2011; 31:1870 - 84; http://dx.doi.org/10.1128/MCB.01393-10; PMID: 21383064
- Kolesnichenko M, Hong L, Liao R, Vogt PK, Sun P. Attenuation of TORC1 signaling delays replicative and oncogenic RAS-induced senescence. [this issue.] Cell Cycle 2012; 11:11 - 2; PMID: 22157090