Abstract
Cells of the early developing mammalian embryo frequently mis-segregate chromosomes during cell division, causing daughter cells to inherit an erroneous numbers of chromosomes. Why the embryo is so susceptible to errors is unknown, and the mechanisms that embryos employ to accomplish chromosome segregation are poorly understood. Chromosome segregation is performed by the spindle, a fusiform-shaped microtubule-based transient organelle. Here we present a detailed analysis of 4D fluorescence-confocal data sets of live embryos progressing from the one-cell embryo stage through to blastocyst in vitro, providing some of the first mechanistic insights into chromosome segregation in the mammalian embryo. We show that chromosome segregation occurs as a combined result of poleward chromosome motion (anaphase-A) and spindle elongation (anaphase-B), which occur simultaneously at the time of cell division. Unexpectedly, however, regulation of the two anaphase mechanisms changes significantly between the first and second embryonic mitoses. In one-cell embryos, the velocity of anaphase-A chromosome motion and the velocity and overall extent of anaphase-B spindle elongation are significantly constrained compared with later stages. As a result chromosomes are delivered close to the center of the forming two-cell stage blastomeres at the end of the first mitosis. In subsequent divisions, anaphase-B spindle elongation is faster and more extensive, resulting in the delivery of chromosomes to the distal plasma membrane of the newly forming blastomeres. Metaphase spindle length scales with cell size from the two-cell stage onwards, but is substantially shorter in the first mitosis than in the second mitosis, and the duration of mitosis-1 is substantially greater than subsequent divisions. Thus, there is a striking and unexpected shift in the approach to cell division between the first and second mitotic divisions, which likely reflects adaptations to the unique environment within the developing embryo.
Introduction
A major goal of cell division is to ensure that replicated chromosomes are accurately and equally dispatched into the two daughter cells. Errors lead to a situation where daughter cells have too few or too many chromosomes, referred to as aneuploidy. Whereas most cells have robust mechanisms for ensuring that the chromosomes are faithfully segregated, human pre-implantation embryo cells frequently mis-segregate chromosomes, leading to so-called “mosaic” or “chaotic” aneuploid embryos, in which blastomeres have different chromosome contents, which may reduce the likelihood of a conceptus being carried to term.Citation1,Citation2 Why the mammalian embryo is susceptible to chromosome segregation errors is not known, and resolving this will require a detailed understanding of the mechanisms of mitosis in this unique setting.
At the heart of chromosome segregation is the spindle, a fusiform-shaped transient organelle assembled from microtubules and associated molecules. Chromosomes attach to microtubules in prometaphase and become aligned at the equator of the spindle in metaphase. Chromosome segregation occurs in anaphase, which in most cells comprises two complementary mechanisms.Citation3,Citation4 In anaphase-A, chromosomes move toward spindle poles as a result of shortening spindle microtubules. In anaphase-B, spindle poles move further apart, in turn, further separating the chromosomes. Two new cells are established around the two newly forming nuclei during cytokinesis. Whereas the mechanism of chromosome segregation has been the subject of intense study in somatic cells,Citation5,Citation6 and more recently, also in oocytes, how the spindle contrives to segregate the chromosomes during anaphase in the mammalian embryo is unknown.
An important feature of pre-implantation development is that cell divisions take place without intervening cell growth, such that cell volume approximately halves with each subsequent division. Experiments in Xenopus and C. elegans embryos suggest that this decreasing cell size has a profound effect upon spindle architecture, smaller cell volume resulting in shorter metaphase spindlesCitation7,Citation8 and a reduced anaphase-B spindle elongation.Citation9 Recent experiments suggest that a similar cell-size to spindle-length relationship exists in metaphase mouse embryos.Citation10 How anaphase is coordinated in mammalian embryos, and whether cell size influences its mechanism, is unknown.
Observation of cell division in real-time in live cells is paramount to understanding the mechanisms of chromosome segregation. For example, live time-lapse imaging recently led to significant advances in understanding several aspects of chromosome segregation in mammalian oocytes, such as the mechanism of spindle assembly,Citation11-Citation13 chromosome alignment,Citation12,Citation14 control of spindle length,Citation15 the mechanism of anaphase chromosome segregationCitation16 and clues as to why chromosome segregation may go awry in oocytes from older mothers.Citation17-Citation19 Here we present a detailed analysis of continuous 4-dimensional fluorescence-confocal time-lapse movies of microtubules and chromosomes in mouse embryos developing from one-cell stage through to blastocyst. The data provide the first information on the mechanism of chromosome segregation in a mammalian embryo. Unexpectedly, our analyses uncover a significant shift in the cellular approach to segregating chromosomes that occurs between the first and second embryonic mitoses.
Results
Analysis of mitosis in embryos using continuous live 4D imaging
To examine mitosis in mammalian embryos, we analyzed continuous 4D time-lapse z-stack imaging data sets of in vitro fertilized embryos expressing mRFP1-tagged Histone-2B (H2B::mRFP1, to label chromosomes) and EGFP-tagged α-tubulin (microtubules) progressing from the pronucleate (one-cell) stage to the blastocyst stage (). Embryos imaged in this manner have previously been shown to develop to term following transfer to pseudo-pregnant mothers, revealing little or no deleterious effect of the imaging protocol upon embryo development.Citation20 We first used these movies to assess the durations of embryonic mitosis. The overall duration of mitosis, defined as the time from nuclear envelope breakdown (NEBD) to chromosome decondensation and the formation of two new nuclei following cytokinesis, gradually decreased from the first mitosis (1–2 cell division; 123+/−9 min) to the fourth mitosis (at which point embryos were compacted morulae; 52+/−3 min). The precise times at which mitotic cell cycle milestones were passed were readily observable by examining the chromatin structure (Fig. 1Ba), and so we assessed the relative durations of prophase/prometaphase (from NEBD until the formation of a metaphase plate), metaphase and anaphase-telophase (ending at the time of chromosome decondensation). Although each of these phases shortened with succeeding mitoses, a substantial reduction of metaphase duration had the most prominent influence upon overall duration of mitosis (Fig. 1Bb), consistent with the previous observation that metaphase is longer in the first mitosis than the second.Citation21,Citation22 The mean duration of metaphase was greater in blastocysts than morula, and the variation in metaphase duration recorded in blastocysts was larger. Thus, the phases of mitosis are prolonged in the one-cell embryo, and establishment of shorter cell cycle durations occurs gradually over the first several cell divisions.
Figure 1. Continuous 4D time-lapse imaging of embryo development for analysis of mitosis. (A) Example of an embryo progressing from the one-cell stage through to blastocyst. Images shown are of the same embryo at the times stated. Images are maximum intensity z-projections of all 51 z-plane acquisitions. The example shown is from a 3.75 min acquisition data set. (B) Use of the data sets to record mitotic cell cycle stage duration throughout pre-implantation development. (a) shows an example of a blastomere from a two-cell embryo progressing through mitosis. The headings describe the stage of the cell cycle of the cell indicated by an arrow. Metaphase is defined here as the time from the establishment of a coherent metaphase plate until the onset of anaphase. (b) shows average durations of the three major phases of mitosis. Note the progressive shortening of cell cycle stage durations during the first several cell cycles. Note also subsequent increase in the duration of metaphase that occurs in blastocysts. (c) shows the same data expressed as a proportion of mitosis attributable to each cell cycle stage; 13 to 39 embryos were analyzed per developmental stage. Error bars indicate SEM.
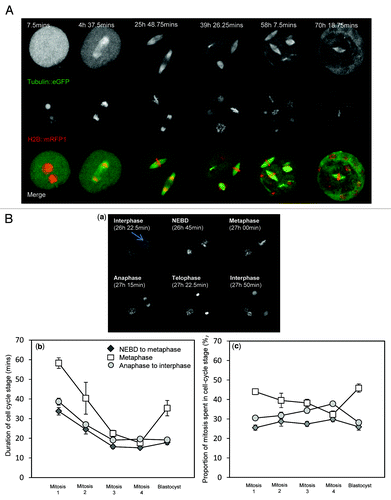
Metaphase spindle length scales with cell size from the two-cell stage onwards
We set out to analyze spindle function and chromosome segregation in embryos and first examined the relationship between spindle length and cell size. Recent studies suggest a strong relationship between cell size and spindle length during early development.Citation8,Citation10 Measurement of spindle length and cell size in live mouse embryos revealed that, from the two-cell stage onwards, there was a progressive decrease in spindle length as pre-implantation development progressed and cell size decreased (). This is consistent with the notion that cell size dictates spindle length during early development. Whereas spindle poles were in relatively close apposition with plasmalemma in metaphase in later embryos, spindle poles were typically ~10 µm or more away from the plasmalemma in two-cell embryos, and over 20 µm away in one-cell embryos. Although we cannot exclude the possibility that interactions between the spindle poles and plasmalemma contribute to spindle length regulation at these earlier stages, we note that astral microtubules that might be responsible for this are sparse or absent in early mouse embryos.Citation15 We conclude that cell size dictates spindle length in later developmental stages, when the cell is small, but that at earlier stages, where cell volume is large, a maximum spindle length is attained.
Figure 2. Metaphase spindle length scales with cell size from the two-cell stage onwards. Analysis of spindle length at developmental stages indicated. Images to the right illustrate the measurements made, with colored lines matching the colors of data points. Cell diameter was measured along the axis of the spindle as illustrated. All spindles analyzed were parallel to the coverslip, as determined by examining sequential z-sections. Notice that in later developmental stages, spindle length decreases with decreasing cell size. Note, also, however, that the metaphase spindle in the one-cell embryo is substantially smaller than that of the two-cell embryo and, as such, does not fit the otherwise apparent relationship between cell size and spindle length. Nuclear diameter was calculated as the average of two orthogonal diameters in the z-projection image. The two data points for nuclear diameter at the one-cell stage are measurements of the larger and smaller pronucleus. Data points represent 14–25 spindles per cell cycle stage. Error bars indicate standard deviation, since SEM was negligible. Different letters indicate significant differences of spindle length at p < 0.01, ANOVA.
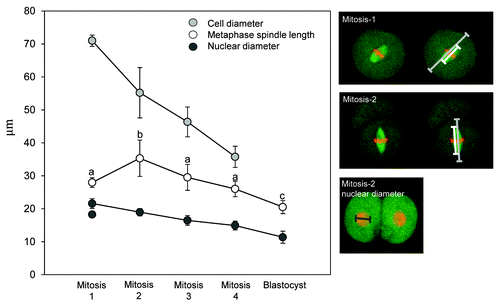
Strikingly, however, we found that metaphase spindles in one-cell embryos were only 28.0+/−0.3 µm in length and, thus, substantially shorter than in two-cell stage embryos (35.3+/−1.3 µm; ANOVA, Tukeys, p < 0.01), despite the larger diameter and volume of the one-cell embryo (). To determine whether this unexpected observation held true for other subcellular structures, we measured nuclear diameter, which is proportional to cellular volume in many systems.Citation23,Citation24 Nuclear diameter scaled with cellular size throughout pre-implantation development, the larger of the two pronuclei in one-cell embryos being bigger than the nucleus of the two-cell embryo (). Thus, whereas spindle length is a function of cell size in later pre-implantation embryos, the spindle is substantially shorter during the first mitosis compared with the second. This unexpected observation alludes to distinct spindle length regulation in the one-cell embryo.
Anaphase-B spindle elongation is restrained during the first mitosis
To further analyze the relationship between cell size and spindle length, we examined anaphase-B spindle elongation. The extent of anaphase-B spindle elongation is an important parameter of cell division, as it determines the final separation distance of the chromosomes and the location of the new nuclei. Anaphase spindle elongation was pronounced in the second and later mitoses, proceeding until spindle poles were in close apposition with the plasmalemma (). As a result, chromosomes were typically adjacent to the plasmalemma at telophase (see ). The consistent close apposition of the spindle poles and chromosomes with the plasmalemma at telophase implies that the cell boundary serves to set the extent of spindle elongation. In accordance with this, mid-late anaphase spindles frequently appeared buckled, as if spindle elongation continued even after the arrival of the poles at the plasmalemma (). Chromosome decondensation took place at the cortex (), and establishment of a central nucleus was achieved by a subsequent slow inward migration of the nucleus. In direct contrast, in the one-cell embryo, anaphase spindle elongation stopped when the spindle was 44.5+/−0.7 µm in length, with spindle poles a substantial distance away from the plasmalemma (; mean pole-plasmalemma distance ~18 µm). Instead, the telophase chromosomes were positioned approximately at the center of the forming two-cell blastomere, where DNA decondensation occurred (). Thus, the extent of anaphase-B is determined by cell diameter from the second mitosis onwards, and we suggest that the distal plasmalemma serves as a physical barrier that helps to curtail spindle elongation. In contrast, anaphase-B in the one-cell embryo is precociously restrained to generate a relatively short telophase spindle that places chromosomes at the center of the forming blastomeres.
Figure 3. The extent of anaphase-B spindle elongation is a function of cell size from the two-cell stage onwards. (A) Examples of analysis of spindle length and cell diameter during mitosis in early development. As previously, cell diameter is measured along the same axis as the spindle, and the spindles were confirmed as parallel to the coverslip. Note that spindle extension is modest in mitosis-1, such that spindle length remains substantially shorter than the length of the cell along the same axis as the spindle (highlighted with red arrows). In contrast, in later mitoses, anaphase spindle extension causes the spindle to assume a length similar to that of the cell diameter (highlighted with blue arrows). Black arrows indicate the onset of anaphase, as identified using H2B::RFP images. (B) Illustrative example images. (a) A spindle at maximal telophase extension at the end of mitosis-1. Note that the decondensing chromosomes are approximately at the center of the forming daughter cells. In some cases, the nucleus subsequently migrated toward the plasmalemma before re-positioning at the cell center. (b) Images of embryos undergoing the second mitosis. Notice in the left panel that spindle poles are in close apposition with the plasmalemma in mid-anaphase, and the spindle appears buckled (arrow). Note in the right-hand panel that the telophase chromosomes are subsequently placed close to the plasmalemma in the cell cortex (arrows). See also for a further example. (c) An embryo in which one blastomere has just completed the third mitosis. Arrowheads indicate newly formed nuclei. Notice that the nuclei are at the extreme opposite sides of the two daughter cells. (C) Analysis of cell diameter and maximal spindle length throughout pre-implantation development. Note that the final extent of chromosome segregation is closely related to cell size from the second mitosis onwards, but not in the first mitosis; 16 to 19 embryos were analyzed per experimental stage. Error bars indicate standard deviation; SEM was negligible. There is no significant difference in telophase spindle length between mitosis-1 and mitosis-3, whereas spindle lengths are significantly different from each other at all other stages; p < 0.01, ANOVA. (D) Telophase spindle length presented as a proportion of the telophase cell diameter. Note that the telophase spindle is substantially shorter than the diameter of the cell in mitosis-1, but extends to ~80% of the cell diameter in subsequent mitoses.
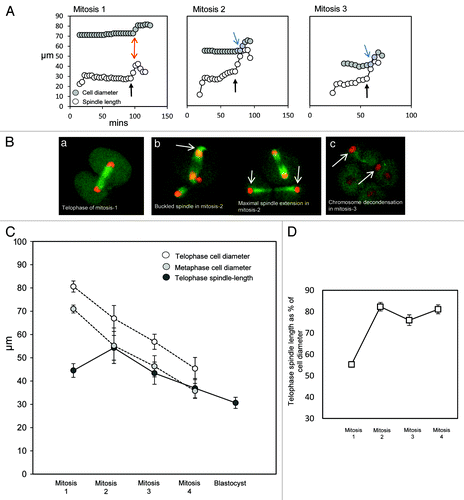
Chromosome segregation comprises simultaneous anaphase-A and anaphase-B
Next we sought to analyze the mechanism of anaphase in developing embryos. In most cell types, chromosome segregation is a combined result of anaphase-A poleward chromosome motion and anaphase-B spindle elongation. In some settings, including early Drosophila embryos,Citation25,Citation26 anaphase-B spindle elongation occurs only after anaphase-A is complete. In contrast, anaphase-B precedes anaphase-A in mouse eggs,Citation16 and the two mechanisms occur simultaneously in some other cell types.Citation3 Moreover, some developmental systems segregate chromosomes in a manner that apparently dispenses with a conventional anaphase-A or -B.Citation27 Thus, the relative contribution of anaphase-A and anaphase-B in any given system has to be established empirically.
To establish the temporal regulation of anaphase in embryos, we measured spindle length and chromosome separation throughout chromosome segregation in blastomeres from every stage of pre-implantation development (). The maximum velocity of chromosome motion in anaphase ranged from 1.6+/−0.03 µm/min in mitosis-1 to 2.3+/−0.1 µm/min in mitosis-3, velocities approximately similar to those in cultured mammalian cellsCitation28 and early C. elegans embryos.Citation9 At all developmental stages, chromosome segregation comprised a shortening of chromosome-pole distance (anaphase-A) and a marked spindle elongation (anaphase-B), which occurred simultaneously in all cases (see ). To analyze the developmental regulation of anaphase, we calculated the velocities of anaphase-A chromosome movement and anaphase-B spindle elongation throughout pre-implantation development. Strikingly, both anaphase components occur substantially slower in one-cell embryos compared with two-cell embryos (). As a result, the overall velocity of anaphase chromosome motion in the first mitosis is significantly less than in the second (ANOVA, Tukeys; p < 0.01).
Figure 4. Chromosome segregation is a result of simultaneous anaphase-A and anaphase-B throughout development. (A) Example images of anaphase in the second mitosis. Note that chromosomes are segregated as a combined result of chromosomes moving toward the spindle poles, and spindle elongation, which occur simultaneously. (B) Graphical representations of anaphase, highlighting the timings of anaphase-A poleward chromosome motion and anaphase-B spindle elongation. Note that at all developmental stages, there is a significant spindle elongation (gray) and a movement of chromosomes toward the poles (black), and that these occur simultaneously. White boxes indicate condensed chromatin that has not yet arranged into a coherent metaphase plate, circles indicate a metaphase plate. (C) Analysis of the velocities of poleward chromosome motion (anaphase-A) and anaphase spindle elongation (anaphase-B). Note that both anaphase components are substantially slower in the first mitosis compared with the second mitosis; 10 to 19 mitoses were analyzed at each developmental stage. Error bars are SEM.
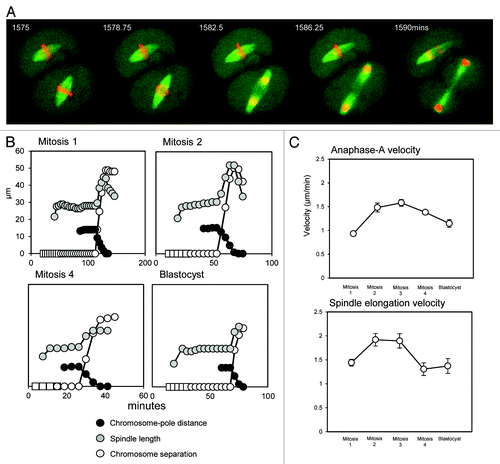
Discussion
Here we exploited high temporal and spatial resolution 4D data sets of chromosome and microtubule dynamics during early development to present some of the first mechanistic information on chromosome segregation in mammalian embryos. The study underscores the power of real-time imaging in understanding the dynamics of early development, corroborating and extending the notions of spindle-length to cell-size scaling during early development and differential durations of cell cycle phases. The high acquisition rate and image quality of the data sets has allowed us to analyze the dynamics of anaphase in a mammalian embryo for the first time. Notably, our analyses reveal that mouse embryo spindles employ a conventional anaphase that, unlike Drosophila embryos or the mouse egg, comprises simultaneous anaphase-A and anaphase-B. The data thus serve as a blueprint for future molecular dissections of chromosome segregation in embryos. Unexpectedly, however, the data reveal differences between the first and subsequent mitoses in all parameters examined, including metaphase spindle length regulation, the speed and extent of anaphase spindle elongation and the velocity of anaphase-A chromosome motion. Each of these is substantially reduced in the one-cell embryo in comparison to subsequent divisions, with the overall effect of reducing the extent of chromosome separation. As a result, new nuclei form close to the cell center in the newly forming two-cell embryo. Later divisions, on the other hand, employ a more rapid and extensive spindle elongation, which causes the greatest possible degree of chromosome separation at telophase, placing the decondensing chromosomes in the distal cortices. This shift in spindle length regulation in early development is summarized in .
Figure 5. Summary: A shift in spindle length regulation after the first mitosis. Cartoon depicting key differences between the first and subsequent mitoses. In the first mitosis, metaphase spindle length is constrained (top left), causing the spindle to be substantially smaller than its container. In contrast, later spindles adopt steady-state metaphase lengths more similar to the size of their cell. Anaphase spindle elongation in the one-cell embryo is constrained (bottom left), such that chromosomes are delivered to the center of the forming daughter cell. In contrast, in later anaphases, the spindle extends to the entire cell length, causing chromosomes to be segregated to the greatest possible extent, being delivered to the distal cortex.
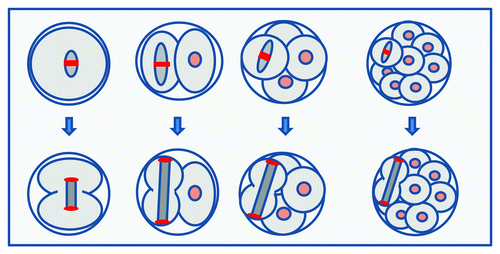
The physiological benefit of this shift in spindle length regulation remains to be addressed. The fusiform shape of the spindle and its elongation in anaphase is generally considered beneficial to the fidelity of chromosome segregation; a substantial distance between the two sister chromosome complements at the time of cytokinesis should decrease the possibility of lagging anaphase chromosomes being lost to the wrong cell.Citation29 It is therefore perhaps intuitive that the later embryo prioritizes maximal spindle elongation to minimize segregation errors that would impact the genome of the developing individual. Why the one-cell embryo has elected to forego this safety measure is mysterious. One possibility relates to the role of the anaphase/telophase spindle midzone in directing the cleavage furrow at cytokinesis. A short spindle midzone in anaphase/telophase might facilitate accurate positioning of the central spindle complex, thereby ensuring accurate placement of cytokinetic furrow and reducing the risk of an unequal cell division that would be hazardous with cells of such large volume.Citation30-Citation33 Control of furrow positioning and formation in early mammals remains to be investigated, though it is noteworthy that inhibition of the Src kinase Fyn impacts the first mitotic cleavage.Citation34 Equally intriguing is the physiological benefit of the prolonged temporal duration of mitosis in the first few embryonic divisions. It is noteworthy that the first mitosis, which is especially prolonged, segregates chromosomes from two pronuclei, as opposed to a single nucleus (see ), which may increase the demands upon nuclear envelope breakdown machinery or increase the geometric difficulty of correctly aligning chromosomes. Future live imaging of kinetochores, as was recently performed in oocytes,Citation14 will be useful for clarifying the finer details of metaphase chromosome alignment mechanisms in embryos.
The molecular mechanism of the switch in spindle length regulation between the first and second mitosis remains to be established. Similar to the one-cell embryo, the metaphase spindle in the egg is small relative to the available cytoplasmic space, and anaphase-B is relatively minor.Citation16 An attractive hypothesis, therefore, is that factors that restrain spindle lengthening in the egg persist in the maternal cytoplasm throughout the first mitosis. A similar argument has been used to explain the prolonged metaphase in the first mitosis.Citation21,Citation22 In this scenario, the first wave of embryonic genome activation during interphase at the two-cell stage may underpin the shift in spindle behavior.Citation35,Citation36 The identities of factors involved are unclear. It is noteworthy, however, that unlike in somatic cells, metaphase spindle-length regulation is critically dependent upon the microtubule-sliding motor kinesin-5 both in one-cell embryo and two-cell embryos.Citation15 This implies that microtubule dynamics, and in particular, a balanced rate of poleward microtubule motion and microtubule disassembly at spindle poles,Citation37-Citation39 play a dominant role in spindle length regulation in early embryos.Citation15 It is noteworthy that in some systems, spindle length is sensitive to the activity of microtubule depolymerizing catalysts, such as kinesin-13s and kinesin-8, whose influence upon the spindle is subject to regulation at several levels.Citation40,Citation41 It is tempting to speculate that modification of MT depolymerization between the first two mitoses may fine-tune the extent to which kinesin-5 can maintain pole separation. Refinement of the balance of microtubule polymerization, depolymerization and poleward sliding dynamics, presents a very plausible explanation for the differential length regulation in the first two mitoses. Finally, it is noteworthy that several recent reports have documented a spindle-shaped cage of actin that plays a key role in spindle positioning in mammalian oocytes.Citation42-Citation44 Although actin perturbation had little impact upon chromosome segregation in meiosis-II in mouse eggs,Citation16 actin may modulate spindle morphology, including spindle length in embryos of Xenopus laevis.Citation45 The role of actin in spindle positioning and chromosome segregation in mammalian embryos remains to be elucidated.
Understanding the mechanism of chromosome segregation in the mammalian embryo is of significant importance, given the high rates of chromosome segregation error observed in human embryos in the IVF clinic. Recent studies in mammalian oocytes emphasize that the mechanism of chromosome segregation in such specialized settings cannot be extrapolated from traditional model systems. Our analysis reveals that the same is true in the embryo, where chromosome segregation has apparently adopted stage-specific specializations which likely reflect the unique environment of the reductive cleavages of pre-implantation development.
Materials and Methods
Data sets
Generation of the imaging data are described previously.Citation20 Briefly, pronucleate (one-cell stage) embryos were generated by IVF using sperm from BDF1 males and metaphase-II eggs from superovulated BDF1 females. H2B::mRFP1 and α-tubulin::EGFP were expressed in one-cell embryos by microinjection of mRNA, using piezo pulses to pierce the plasmalemma. For imaging, embryos were cultured in CZB medium in a temperature- and atmosphere-controlled chamber (Tokai-Hit), in 5% CO2 balanced with air. Imaging was performed using a CSU10 Yokagawa Nipkow disk spinning disk confocal system, mounted on an Olympus IX-71 Inverted microscope, using a 20x objective lens (UPlanApo 20 × NA = 0.8). Embryos were analyzed from experiments with imaging frequencies of 3.75, 7.5 and 15 min. Embryos examined using this protocol are capable of generating live offspring.Citation20 Fifty-one z-plane images were acquired at each time point.
Data analysis
Analysis was performed using ImageJ software. All images shown are z-plane maximum intensity projections. For all measurements of spindle length and chromosome separation distances, individual z-slices were examined to ensure that only cells whose spindle remained parallel to the coverslip were measured, in order to report distances and lengths accurately. Data were analyzed from experimental data sets with image acquisition periods of 3.75, 7.5 or 15 min. Measurements of velocity and duration were made exclusively from 3.75 min and 7.5 min data sets, whereas measurements of metaphase and telophase spindle length were possible from all data sets. Imaging frequency had no effect upon any of the parameters investigated, and all results and trends reported were consistent across all data sets. The total number of cells analyzed for any given measurement is stated in figure legends. All velocities are calculated using the maximum distance moved in a given 7.5 min period.
Disclosure of Potential Conflicts of Interest
No potential conflicts of interest were disclosed.
References
- Fragouli E, Wells D. Aneuploidy in the human blastocyst. Cytogenet Genome Res 2011; 133:149 - 59; http://dx.doi.org/10.1159/000323500; PMID: 21252488
- Harper JC, Pergament E, Delhanty JD. Genetics of gametes and embryos. Eur J Obstet Gynecol Reprod Biol 2004; 115:Suppl 1 S80 - 4; http://dx.doi.org/10.1016/j.ejogrb.2004.01.018; PMID: 15196722
- Maiato H, Lince-Faria M. The perpetual movements of anaphase. Cell Mol Life Sci 2010; 67:2251 - 69; http://dx.doi.org/10.1007/s00018-010-0327-5; PMID: 20306325
- Ris H. The anaphase movement of chromosomes in the spermatocytes of the grasshopper. Biol Bull 1949; 96:90 - 106; http://dx.doi.org/10.2307/1538001; PMID: 18108895
- Compton DA. Spindle assembly in animal cells. Annu Rev Biochem 2000; 69:95 - 114; http://dx.doi.org/10.1146/annurev.biochem.69.1.95; PMID: 10966454
- Walczak CE, Cai S, Khodjakov A. Mechanisms of chromosome behaviour during mitosis. Nat Rev Mol Cell Biol 2010; 11:91 - 102; PMID: 20068571
- Greenan G, Brangwynne CP, Jaensch S, Gharakhani J, Jülicher F, Hyman AA. Centrosome size sets mitotic spindle length in Caenorhabditis elegans embryos. Curr Biol 2010; 20:353 - 8; http://dx.doi.org/10.1016/j.cub.2009.12.050; PMID: 20137951
- Wühr M, Chen Y, Dumont S, Groen AC, Needleman DJ, Salic A, et al. Evidence for an upper limit to mitotic spindle length. Curr Biol 2008; 18:1256 - 61; http://dx.doi.org/10.1016/j.cub.2008.07.092; PMID: 18718761
- Hara Y, Kimura A. Cell-size-dependent spindle elongation in the Caenorhabditis elegans early embryo. Curr Biol 2009; 19:1549 - 54; http://dx.doi.org/10.1016/j.cub.2009.07.050; PMID: 19682904
- Courtois A, Schuh M, Ellenberg J, Hiiragi T. The transition from meiotic to mitotic spindle assembly is gradual during early mammalian development. J Cell Biol 2012; 198:357 - 70; http://dx.doi.org/10.1083/jcb.201202135; PMID: 22851319
- Schuh M, Ellenberg J. Self-organization of MTOCs replaces centrosome function during acentrosomal spindle assembly in live mouse oocytes. Cell 2007; 130:484 - 98; http://dx.doi.org/10.1016/j.cell.2007.06.025; PMID: 17693257
- Breuer M, Kolano A, Kwon M, Li CC, Tsai TF, Pellman D, et al. HURP permits MTOC sorting for robust meiotic spindle bipolarity, similar to extra centrosome clustering in cancer cells. J Cell Biol 2010; 191:1251 - 60; http://dx.doi.org/10.1083/jcb.201005065; PMID: 21173113
- Dumont J, Petri S, Pellegrin F, Terret ME, Bohnsack MT, Rassinier P, et al. A centriole- and RanGTP-independent spindle assembly pathway in meiosis I of vertebrate oocytes. J Cell Biol 2007; 176:295 - 305; http://dx.doi.org/10.1083/jcb.200605199; PMID: 17261848
- Kitajima TS, Ohsugi M, Ellenberg J. Complete kinetochore tracking reveals error-prone homologous chromosome biorientation in mammalian oocytes. Cell 2011; 146:568 - 81; http://dx.doi.org/10.1016/j.cell.2011.07.031; PMID: 21854982
- Fitzharris G. A shift from kinesin 5-dependent metaphase spindle function during pre-implantation development in mouse. Development 2009; 136:2111 - 9; http://dx.doi.org/10.1242/dev.035089; PMID: 19465601
- FitzHarris G. Anaphase B precedes anaphase A in the mouse egg. Curr Biol 2012; 22:437 - 44; http://dx.doi.org/10.1016/j.cub.2012.01.041; PMID: 22342753
- Lane SI, Yun Y, Jones KT. Timing of anaphase-promoting complex activation in mouse oocytes is predicted by microtubule-kinetochore attachment but not by bivalent alignment or tension. Development 2012; 139:1947 - 55; http://dx.doi.org/10.1242/dev.077040; PMID: 22513370
- Lister LM, Kouznetsova A, Hyslop LA, Kalleas D, Pace SL, Barel JC, et al. Age-related meiotic segregation errors in mammalian oocytes are preceded by depletion of cohesin and Sgo2. Curr Biol 2010; 20:1511 - 21; http://dx.doi.org/10.1016/j.cub.2010.08.023; PMID: 20817533
- Chiang T, Duncan FE, Schindler K, Schultz RM, Lampson MA. Evidence that weakened centromere cohesion is a leading cause of age-related aneuploidy in oocytes. Curr Biol 2010; 20:1522 - 8; http://dx.doi.org/10.1016/j.cub.2010.06.069; PMID: 20817534
- Yamagata K, Suetsugu R, Wakayama T. Long-term, six-dimensional live-cell imaging for the mouse pre-implantation embryo that does not affect full-term development. J Reprod Dev 2009; 55:343 - 50; http://dx.doi.org/10.1262/jrd.20166; PMID: 19305125
- Kubiak JZ, Chesnel F, Richard-Parpaillon L, Bazile F, Pascal A, Polanski Z, et al. Temporal regulation of the first mitosis in Xenopus and mouse embryos. Mol Cell Endocrinol 2008; 282:63 - 9; http://dx.doi.org/10.1016/j.mce.2007.11.023; PMID: 18178304
- Sikora-Polaczek M, Hupalowska A, Polanski Z, Kubiak JZ, Ciemerych MA. The first mitosis of the mouse embryo is prolonged by transitional metaphase arrest. Biol Reprod 2006; 74:734 - 43; http://dx.doi.org/10.1095/biolreprod.105.047092; PMID: 16382027
- Hara Y, Kimura A. Cell-size-dependent control of organelle sizes during development. Results Probl Cell Differ 2011; 53:93 - 108; http://dx.doi.org/10.1007/978-3-642-19065-0_5; PMID: 21630142
- Jorgensen P, Tyers M. How cells coordinate growth and division. Curr Biol 2004; 14:R1014 - 27; http://dx.doi.org/10.1016/j.cub.2004.11.027; PMID: 15589139
- Brust-Mascher I, Civelekoglu-Scholey G, Kwon M, Mogilner A, Scholey JM. Model for anaphase B: role of three mitotic motors in a switch from poleward flux to spindle elongation. Proc Natl Acad Sci USA 2004; 101:15938 - 43; http://dx.doi.org/10.1073/pnas.0407044101; PMID: 15522967
- Brust-Mascher I, Scholey JM. Microtubule flux and sliding in mitotic spindles of Drosophila embryos. Mol Biol Cell 2002; 13:3967 - 75; http://dx.doi.org/10.1091/mbc.02-05-0069; PMID: 12429839
- Dumont J, Oegema K, Desai A. A kinetochore-independent mechanism drives anaphase chromosome separation during acentrosomal meiosis. Nat Cell Biol 2010; 12:894 - 901; http://dx.doi.org/10.1038/ncb2093; PMID: 20729837
- Mayr MI, Hümmer S, Bormann J, Grüner T, Adio S, Woehlke G, et al. The human kinesin Kif18A is a motile microtubule depolymerase essential for chromosome congression. Curr Biol 2007; 17:488 - 98; http://dx.doi.org/10.1016/j.cub.2007.02.036; PMID: 17346968
- Salmon ED, Cimini D, Cameron LA, DeLuca JG. Merotelic kinetochores in mammalian tissue cells. Philos Trans R Soc Lond B Biol Sci 2005; 360:553 - 68; http://dx.doi.org/10.1098/rstb.2004.1610; PMID: 15897180
- Dechant R, Glotzer M. Centrosome separation and central spindle assembly act in redundant pathways that regulate microtubule density and trigger cleavage furrow formation. Dev Cell 2003; 4:333 - 44; http://dx.doi.org/10.1016/S1534-5807(03)00057-1; PMID: 12636915
- Glotzer M. Cleavage furrow positioning. J Cell Biol 2004; 164:347 - 51; http://dx.doi.org/10.1083/jcb.200310112; PMID: 14757750
- von Dassow G, von. Concurrent cues for cytokinetic furrow induction in animal cells. Trends Cell Biol 2009; 19:165 - 73; http://dx.doi.org/10.1016/j.tcb.2009.01.008; PMID: 19285868
- Glotzer M. The molecular requirements for cytokinesis. Science 2005; 307:1735 - 9; http://dx.doi.org/10.1126/science.1096896; PMID: 15774750
- Levi M, Maro B, Shalgi R. Fyn kinase is involved in cleavage furrow ingression during meiosis and mitosis. Reproduction 2010; 140:827 - 34; http://dx.doi.org/10.1530/REP-10-0312; PMID: 20841362
- Hamatani T, Carter MG, Sharov AA, Ko MS. Dynamics of global gene expression changes during mouse pre-implantation development. Dev Cell 2004; 6:117 - 31; http://dx.doi.org/10.1016/S1534-5807(03)00373-3; PMID: 14723852
- Li L, Zheng P, Dean J. Maternal control of early mouse development. Development 2010; 137:859 - 70; http://dx.doi.org/10.1242/dev.039487; PMID: 20179092
- Goshima G, Scholey JM. Control of mitotic spindle length. Annu Rev Cell Dev Biol 2010; 26:21 - 57; http://dx.doi.org/10.1146/annurev-cellbio-100109-104006; PMID: 20604709
- Dumont S, Mitchison TJ. Force and length in the mitotic spindle. Curr Biol 2009; 19:R749 - 61; http://dx.doi.org/10.1016/j.cub.2009.07.028; PMID: 19906577
- Rogers GC, Rogers SL, Sharp DJ. Spindle microtubules in flux. J Cell Sci 2005; 118:1105 - 16; http://dx.doi.org/10.1242/jcs.02284; PMID: 15764594
- Ems-McClung SC, Walczak CE. Kinesin-13s in mitosis: Key players in the spatial and temporal organization of spindle microtubules. Semin Cell Dev Biol 2010; 21:276 - 82; http://dx.doi.org/10.1016/j.semcdb.2010.01.016; PMID: 20109574
- Howard J, Hyman AA. Microtubule polymerases and depolymerases. Curr Opin Cell Biol 2007; 19:31 - 5; http://dx.doi.org/10.1016/j.ceb.2006.12.009; PMID: 17184986
- Schuh M, Ellenberg J. A new model for asymmetric spindle positioning in mouse oocytes. Curr Biol 2008; 18:1986 - 92; http://dx.doi.org/10.1016/j.cub.2008.11.022; PMID: 19062278
- Azoury J, Lee KW, Georget V, Rassinier P, Leader B, Verlhac MH. Spindle positioning in mouse oocytes relies on a dynamic meshwork of actin filaments. Curr Biol 2008; 18:1514 - 9; http://dx.doi.org/10.1016/j.cub.2008.08.044; PMID: 18848445
- Yi K, Unruh JR, Deng M, Slaughter BD, Rubinstein B, Li R. Dynamic maintenance of asymmetric meiotic spindle position through Arp2/3-complex-driven cytoplasmic streaming in mouse oocytes. Nat Cell Biol 2011; 13:1252 - 8; http://dx.doi.org/10.1038/ncb2320; PMID: 21874009
- Woolner S, O’Brien LL, Wiese C, Bement WM. Myosin-10 and actin filaments are essential for mitotic spindle function. J Cell Biol 2008; 182:77 - 88; http://dx.doi.org/10.1083/jcb.200804062; PMID: 18606852