Abstract
We recently reported that mouse embryonic stem cells (ESCs) in S/G2 are more efficient at reprogramming somatic cells than ESCs at other stages of the cell cycle. We also provided evidence that DNA replication is induced in the nuclei of somatic partners upon fusion with ESC partners, and showed that this was critical for their conversion toward a pluripotent state.Citation1 Here we have used counterflow centrifugal elutriation to enrich for ESCs at different cell cycle phases, so as to examine in detail the properties of S/G2 phase cells. This revealed that the replication and organization of DAPI-intense heterochromatin in ESCs is unusual in two respects. First, replication of heterochromatin occurred earlier during S phase and was associated with precocious H3S10 phosphorylation. Second, heterochromatin protein 1 α (HP1α), which invariably marks DAPI-intense and H3K9me3-enriched pericentromeric domains in mouse somatic cells,Citation2 was not necessarily associated with these H3K9me3-enriched domains in undifferentiated ESCs. These data, which complement recent replication timingCitation3 and electron spectroscopic imaging (ESI) analyses,Citation4 suggest that heterochromatin is atypical in ESCs. Interestingly, as these unusual features were rapidly acquired by somatic nuclei upon ESC fusion-mediated reprogramming, our results suggest that fundamental changes in cell cycle structure and heterochromatin dynamics may be important for conferring pluripotency.
Introduction
Undifferentiated mouse ESCs have an unusual cell cycle structure in which the Gap phases G1 and G2 are truncated, and a large proportion of cells are in DNA synthesis (S) phase, as compared with somatic cells.Citation5,Citation6 Similar properties are also seen in vivo among the transient population of pluripotent cells within the inner cell massCitation7 and in vitro among induced pluripotent stem (iPS) cell lines derived from fibroblasts.Citation5,Citation8 Although the significance of this altered cell cycle structure is not really known, recent reports have suggested that many proteins that regulate cell cycle stage transitions, including geminin, are expressed aberrantly in ESCs,Citation9,Citation10 and that any disruption of this unusual cell cycle profile can promote ESC differentiation.Citation11 The likely importance of this altered cell cycle structure for maintaining pluripotency is underscored by evidence that pluripotent cellsCitation12 and ESCs lose this unusual profile upon differentiation,Citation13-Citation16 and, conversely, that somatic cells regain it when reprogrammed.Citation17,Citation18
We recently used a biophysical separation method to fractionate mouse ESCs according to size and cell cycle stage.Citation19 Using this approach, we showed that ESCs in late S/G2 are particularly effective at reprogramming somatic cells in experimental heterokaryons formed with lymphocytes or fibroblasts.Citation1 ESCs in late S phase and G2 were shown to induce DNA replication in a high proportion of somatic nuclei following cell fusion and, in addition, contained slightly elevated levels of Sox2 and Oct4 proteins. As both features might be relevant to reprogramming, we examined other features of S and G2 that might contribute to pluripotency and the enhanced reprogramming of cell cycle-enriched ESCs. Using elutriated ESCs samples and ESC-derived somatic heterokaryons, together with antibodies that label distinct epigenetic modifications, here we show that heterochromatin structure and dynamics are unusual in undifferentiated ESCs, and that reprogrammed somatic nuclei acquire this atypical heterochromatin after fusion with ESCs.
Results
The ESC cell cycle is characterized by relatively short G1 and G2 phases and an unusual S phase
Pluripotent mouse ESCs contain a high proportion of cells in S phase and have truncated G1 and G2 phases, as compared with differentiated somatic cells.Citation6 This is evident in the histogram profiles of unsynchronized E14tg2A ESCs (E14) stained with propidium iodide (PI) to estimate DNA content, as compared with somatic murine B cells (). Typically, undifferentiated ESC lines contained between 40% and 55% of cells in S phase (43% in this example). In contrast, relatively few cells were detected G1 (29%) as compared with the majority (69%) of murine B cells. Fractionation of ESCs according to cell size and density, which is a reliable correlate of cell cycle stage, was accomplished using counterflow centrifugal elutriation (Materials and Methods), where single-cell suspensions of at least 250 million cells were loaded into an elutriation chamber and centrifuged at constant speed. Fractions collected at increasing flow rates (6–17 ml/min) were examined by PI staining and FACS analysis to estimate the cell cycle composition. The results of a typical elutriation experiment of E14 ESCs are shown in . Here, fractions 8, 12, and 16 showed a selective enrichment of ESCs in G1, S, and G2/M, respectively.
Figure 1. Cell cycle profiles of E14 ESCs, murine B cells, and separation of E14 ESCs using counterflow centrifugal elutriation. (A) Cell cycle distribution profiles of unsynchronized populations of E14tg2A (E14) ESCs and mouse B cells. DNA content was measured by FACS after staining with propidium iodide (PI). Typical percentage estimates of G1, S, and G2/M phases of the cell cycle are shown for each cell type. (B) Cell cycle distribution profiles of fractions of E14 ESCs enriched in G1, S, and G2 phase cells obtained by counterflow centrifugal elutriation are shown, together with representative enrichment percentages for each cell cycle stage. DNA content was measured by FACS after staining with propidium iodide (PI). Fraction numbers reflect flow rate. (C) Confocal images showing the temporal order of S-phase progression in E14 ESCs, assessed by BrdU incorporation patterns (green). Early S phase (stage 1) is characterized by a fine diffuse labeling of multiple euchromatic sites that increase in number and intensity by stage 2. Stage 3 (a–c*) is distinguished by foci representing replicating peri-centromeric and then centromeric heterochromatin, predominantly at the nuclear periphery (early stage 3, a) and later also around the nucleolus (mid stage 3, b). ESCs specifically show a pronounced increase in foci at late stage 3 compared with differentiated somatic cells (c*). Later in S phase (stage 4 and stage 5), large constitutive heterochromatic domains are evident. The percentage of cells undergoing each stage in a typical unsynchronized population of E14 ESCs shown below. Scale bar, 5 μm. (D) Confocal image of a representative heterokaryon (human B x mouse ESC E14) pulse-labeled with BrdU for 45 min, 24 h after cell fusion and then stained to reveal BrdU (green) and DNA (DAPI, blue). The arrow indicates the human B partner undergoing replication resembling the atypical BrdU incorporation pattern shown in Figure 1C, c* above. Scale bars, 5 μm.Citation1
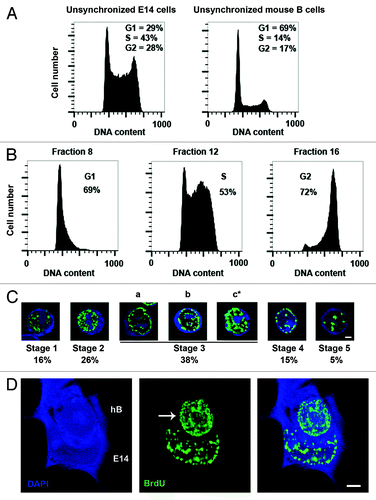
Using a series of elutriated fractions with gradually increasing flow rates to span S phase, ESCs at different stages of S phase were confirmed and ordered into a temporal sequence by labeling with the thymidine analogs BrdU or EdU (100 μM applied as a 45 min pulse, as described in “Materials and Methods”).Citation1,Citation20 Cells representing early stages of S phase in which euchromatic sites were selectively labeled and distributed as a fine (stage 1) or particulate (stage 2) nuclear haze were abundant (16% and 26% of S phase cells, respectively, ). Cells representing late stages of S phase, in which constitutive heterochromatin was labeled and distributed as large peripheral or central foci (stages 4 and 5, ), were also evident in these fractions. Pericentric and centromeric heterochromatin, which generally replicates in the middle of S phase,Citation21 characterized cells in stage 3, where BrdU foci were distributed at the nuclear periphery and around nucleoli. The size and intensity of labeled foci generally increased as ESCs progressed through mid S phase. We noticed, however, an unusually increased number of bright foci and a relatively intense pattern of labeling among cells late in stage 3 (, c*). This pattern was not seen among similar cell cycle fractions obtained from somatic cells, including B lymphocytes or fibroblasts, but is characteristic of all other mouse and human ESCs we have tested to date (not shown). The pronounced labeling of distributed heterochromatic sites in mid-S phase therefore appears to be an unusual feature of ESC replication. At present the basis of this atypical nucleotide incorporation pattern is not known. It is possible that it reflects increased DNA repair or that a subset of chromatin that is late replicating in differentiated cells replicates earlier in ESCs, in agreement with previous reports.Citation22,Citation23 To determine whether this unusual replication profile is likely to be relevant for the pluripotent state, we asked whether somatic cells show altered mid-S (stage 3c*) labeling upon reprogramming.
Somatic nuclei acquire an atypical mid S phase replication profile after cell fusion-mediated pluripotent reprogramming
The dominant reprogramming of somatic nuclei following fusion with ESCs requires DNA replication by the somatic partner within the newly formed heterokaryons.Citation1 Since S phase progression in ESCs appears different from that in somatic cells, and since it has been reported that the re-establishment of ESC-like S phase by somatic cells might be a barrier to successful reprogramming,Citation23 we examined DNA replication by somatic nuclei in heterokaryons 6 to 48 h after their formation by cell fusion. Human B cells were fused in a 1:1 ratio with E14 ESCs, and the resulting heterokaryons were pulse labeled for 45 min with BrdU at 6, 24, or 48 h after cell fusion (see “Materials and Methods”). Reprogramming in heterokaryons is relatively rare, and we have previously estimated that probably no more than 10% of the correctly formed cell bivalents go on to stably express human pluripotency markers such as SSEA4.Citation24 We therefore used confocal microscopy and BrdU pulse labeling to examine human nuclei undergoing DNA synthesis within human B × mouse ESC heterokaryons. Mouse nuclei were discriminated on the basis of DAPI-punctate labeling, and a large number of heterokaryons (>150) containing S-phase human nuclei were examined. We found that among heterokaryons labeled with BrdU 24 h post-fusion, a small but reproducible number of cells showed a pattern of BrdU incorporation in the human B partner that resembled the unusual mid-S stage 3 pattern observed in mouse ESCs. In these human nuclei, intense labeling and additional sites of BrdU incorporation were evident, as highlighted in green in (human nucleus arrowed). We noted that a similar number of heterokaryons displayed this pattern at around 24 h post-fusion, as had previously been estimated to be undergoing reprograming on the basis of human SSEA4 expression.Citation24
H3S10Ph appears precociously during early S phase in ESCs
These results suggested that the early replication of heterochromatic domains in ESCs, as well as in reprogrammed somatic cells, might be an important aspect (or correlate) of pluripotency. To investigate this possibility further, we used antibodies against different histone modifications to better characterize the epigenetic landscape of ESCs during the cell cycle. To help visualize heterochromatin, E14 cells expressing HP1α-GFP were used for these analyses (see “Materials and Methods”). HP1α-GFP distribution in these ESCs was indistinguishable from that of endogenous HP1α proteins, as indicated by co-incident staining of GFP and anti-HP1α antibody (Fig. S1A). HP1α-GFP ESCs were fractionated by counterflow centrifugal elutriation, pulse labeled with EdU (100 μM, 45 min) to distinguish S phase stages, and then co-stained with antibodies to specific histone modifications, such as phosphorylated histone H3 at Serine 10 (H3S10Ph),Citation25 before being examined by confocal microscopy. Initial studies showed that H3S10Ph was a prominent marker of somatic cells and ESCs during mitosis, where it was seen to coat condensing chromosomes prior to cell division (, red, fourth panels). Interestingly, H3S10Ph was also detected at discrete sites in early S phase in E14 samples (, second panel) and this was selective for ESCs, since H3S10Ph marked only later stages of S phase in mouse B cells (, third panel). This suggested that H3S10Ph might be precociously loaded onto ESC chromatin early in DNA synthesis phase.
Figure 2. H3S10Ph is loaded onto chromatin precociously in S phase in E14 ESCs. (A) Confocal images of Murine B cells and (B) E14 ESCs showing EdU (white), H3S10Ph (red) and DAPI (blue) at G1, early S phase, late S phase, and metaphase stages of the cell cycle. H3S10Ph is present during early S phase in E14 ESCs (B, second panel, stage 2), prior to the replication of centromeric heterochromatin, which occurs from stage 3 onwards. Both murine B cells and E14 ESCs contain increasing amounts of H3S10Ph on chromatin in late S phase (A and B, third panels). H3S10Ph coats the chromatids by metaphase (A and B, fourth panels). Scale bars, 2 μm.
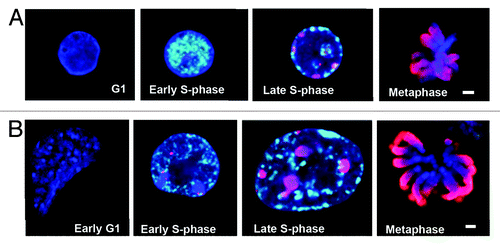
To confirm and extend these results, we carefully monitored H3S10Ph labeling throughout all stages of the ESC cell cycle using the same approach. As illustrated in , H3S10Ph was present at all but the very earliest stages of S phase in ESCs and often highlighted DAPI-dense heterochromatin. H3S10Ph could be detected at replicating heterochromatic sites in cells as early as stage 2 of S phase, and we estimated that approximately 5% of E14-HP1α-GFP cells in stage 3 had large amounts of H3S10Ph at heterochromatic sites, while the remainder showed lower levels of H3S10Ph. As cells progressed to stage 4, replicating centromeric heterochromatin was heavily labeled with H3S10Ph in E14 ES cells, as well as in murine B cells (data not shown). Later, intense H3S10Ph labeling was prominent on the condensing chromosome arms in ESCs late in G2 phase, and entirely coated chromosomes at mitosis and during telophase (red, , row 4), after displacement of HP1α. H3S10Ph signal intensity declined during telophase, and by early G1 of the next cell cycle, only residual levels were detected (, row 5).
Figure 3. H3S10Ph is loaded onto chromatin during S phase in E14-HP1α-GFP ESCs Confocal images of E14-HP1α-GFP ESCs showing the changing distributions of H3S10Ph (red) and HP1α (green) during cell cycle progression. (A) EdU incorporation patterns (white) characterize the progression of S phase (A, rows 1–5). (B) The changing distribution of H3S10Ph from G2 to the following G1 stage is shown (B, rows 1–5). DNA was stained with DAPI (blue). No H3S10Ph was detected during G1 and very early S phase (A, first row). Small foci of H3S10Ph appeared during the first half of S phase, including stage 2 (A, second row) and early stage 3 (A, third row), prior to the replication of centromeric heterochromatin, in approximately 10% of the S phase-enriched population of E14 ESCs. The H3S10Ph foci typically increase in size during stage 3 (A, fourth row) and spread to cover the centromeric heterochromatin during stages 4 and 5 (A, fifth row). H3S10Ph staining increases to cover the chromosome arms as chromosomes begin to condense during G2 (B, first row), and the signal increases in intensity during late G2 (B, second row). The chromosome arms are completely covered by H3S10Ph at metaphase when H3S10Ph signal intensity is maximal (B, third row). H3S10Ph intensity declines during telophase (B, fourth row) leaving few remnants on the chromatin at the start of the next G1 phase (B, fifth row). HP1α-GFP is enriched at heterochromatin during S phase and G1 (A, rows 1–5, B, fifth row) and is redistributed away from heterochromatin during late G2 phase (co-incident with chromosome condensation, B, first and second rows), and is not associated with chromatin during cell division (B, third and fourth rows). HP1α becomes concentrated at centromeric heterochromatin in early G1 (row 10). Scale bars, 5 μm.
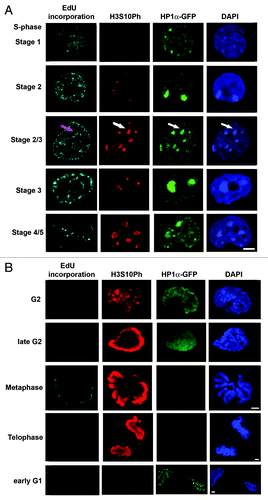
Changes in HP1α dynamics in ESCs
One of the surprising aspects of this observation was that precocious loading of H3S10Ph at centromeric heterochromatin in ESCs did not appear to precipitate the dissociation of HP1α, at least at the global scale offered by this analysis. It has been proposed that HP1α localizes to heterochromatin by binding to the tri-methylated tails of histone H3 at Lysine 9 (H3K9me3).Citation26-Citation28 It has also been shown that Aurora B-mediated phosphorylation of the adjacent H3 serine 10 residues displaces HP1 binding from mitotic heterochromatin and forms part of a binary “methyl/phospho” switch that is critical for proper cell division in yeastCitation29 and mammalian cells.Citation30 In mammals, recruitment of the AuroB/AIM-1 kinase complex to HP1α sites in heterochromatin is reported to occur in G2 and is important for the G2/M transition.Citation31-Citation34 Our data revealed that H3S10Ph was detected at DAPI-dense, HP1α-marked areas of chromatin in S phase in ESCs (, row 3, white arrows). Loading at heterochromatic centromeric clusters and other, smaller foci of DAPI-intense chromatin was observed in both murine B cells and E14 ESCs, and a greater number of small H3S10Ph foci that did not co-localize with DAPI-intense chromatin were observed in ESCs compared with B cells (not shown). We observed that among populations of murine B cells labeled with EdU and H3S10Ph, loading of H3S10Ph appeared to occur only after replication of heterochromatin (i.e., during late S phase). However, in a subpopulation (10%) of E14 ESCs, acquisition of H3S10Ph occurred earlier in S phase and was present at DAPI-bright heterochromatin co-incident with or before DNA replication (, third row, pink arrow).
Altered epigenetic landscape of heterochromatin in ESCs
In somatic cells, HP1α is enriched at all centromeric and pericentromeric heterochromatin domains throughout interphase.Citation2 Using E14-HP1α-GFP or E14 ESCs and antibodies to H3K9me3, H3K27me3, HP1α, and H3S10Ph, we noticed that an approximately 10% of unsynchronized ESCs showed reduced levels of HP1α in their nuclei, together with reduced incorporation of HP1α at DAPI-intense nuclear regions. In a small proportion of ESCs (1–3%), no enrichment of HP1α at centromeric clusters was seen, and the level of HP1α protein in these cells was either low or negligible. This subset of cells was not restricted to G2 phase, being present in G1 and S phase fractions (; exemplified in ). Interestingly, heterochromatic foci that lacked or showed reduced amounts of HP1α always contained high levels of H3K9me3 (, white arows).
Table 1. The percentage of cells with reduced or absent centromeric HP1α does not change significantly during cell cycle progression
Figure 4. Some heterochromatic foci in E14 ESCs lack HP1α while retaining the HP1α recruiting modification trimethylated H3K9. (A) Confocal image showing a group of E14 ESCs in which anti-H3K9me3 (red) is concentrated at all centromeric heterochromatic foci (DAPI-intense, blue). Some cells lack concentrated HP1α-GFP at some (bottom right hand cell) or all of the heterochromatic centromeric foci (arrows, merged image). The cells show no evidence of DNA condensation, i.e., they have not reached the stage of G2 when HP1α is ejected from the heterochromatin. (B) Confocal image of a group of E14-HP1α-GFP ESCs in which HP1α-GFP (green) and Nanog (red) are shown. Heterochromatic centromeric foci appear DAPI-intense (blue). The central cell lacks HP1α and yet contains a high level of Nanog protein (red), indicating that it has not differentiated during culture. (C) Confocal image of E14-HP1α-GFP ESCs during S phase, showing anti-H3S10Ph (red) at replicating (EdU positive, white) heterochromatic (DAPI-intense, blue) foci lacking concentrated HP1α-GFP (green). (D) Confocal images of E14 ESCs (top row) and mouse B cells (bottom row) stained with anti-trimethylated H3K27 (red) and DAPI (blue). Twenty percent of unsynchronized E14 ESCs contain bright foci of trimethylated H3K27, most often associated with heterochromatin (DAPI-intense, blue). Such foci were not observed in somatic murine B cells. Scale bars, 5 μm.
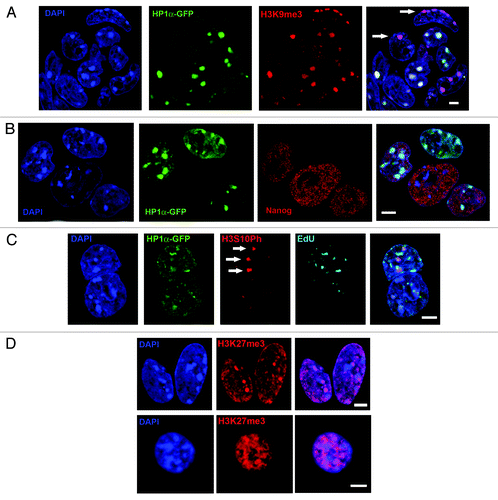
Importantly, we excluded that ESCs showing altered HP1α were not partially differentiated cells by co-staining with Nanog, a marker associated with pluripotencyCitation35,Citation36 (). In addition, E14 cells stained directly with anti-HP1α showed a similar variability in binding at H3K9me3-rich sites as observed using HP1α-GFP (not shown). This effectively rules out the possibility that variation in HP1α binding relates to the HP1α–GFP construct. We also noted that different heterochromatic foci within an individual ESC often showed variable HP1α binding (exemplified in , lower right cell) and that cells with altered HP1α levels did not show any sign of chromosome condensation or doubled chromosome content, and were therefore not likely to be in G2 (when HP1α dissociation from heterochromatin is thought to initiate).Citation31,Citation32
Other reports have suggested that despite the appearance of DAPI-bright regions in ESC nuclei, heterochromatin may be unusually folded or de-compacted,Citation4,Citation37 perhaps explaining the lack of HP1α binding. However, we observed that H3S10Ph correctly marked newly replicated chromatin in S phase ESCs despite reduced levels of HP1α at these regions (, white arrows). Staining of elutriated ESCs with antibodies to euchromatin-associated histone modifications, such as acetylated H3K9 and H4K16, did not reveal any altered cell cycle dynamics of these markers as compared with murine B cells, at least at the resolution provided by confocal microscopy (Fig. S1B). In the case of H3K27me3, an epigenetic modification associated with facultative heterochromatinCitation38 and bivalent chromatinCitation39 in ES cells, we observed bright foci of H3K27me3 signal in around 20% of asynchronous ESCs and noted that several small foci were often associated with DAPI-dense heterochromatin, which was not seen in mouse B cells (). Taken together, these results suggest the organization composition and dynamics of heterochromatin are atypical in ESCs as compared with their somatic counterparts.
Discussion
Mouse ESCs have an unusual cell cycle structure and contain a large proportion of cells in DNA synthesis (S) phase. The progression of S phase in mouse ESCs is also unusual, in that a large proportion of the genome replicates earlier in ESCs than in somatic cells.Citation22 This was observed here as an increased number of foci visible in mid-S phase following a short pulse labeling with thymidine analogs. In addition, it appears that H3S10Ph loading onto chromatin, which initiates precociously in the first half of S phase at DAPI-intense/heterochromatic regions in ESCs, can also be incorporated into heterochromatin that has yet to be replicated. In contrast, loading of H3S10Ph onto heterochromatin in somatic mouse B cells occurs later in S phase, following its replication. It is formally possible that the precocious replication of heterochromatin in ESCs, together with the precocious loading of H3S10Ph, could account for the altered dynamics of HP1α distribution that we report here. A similar number of cells with equivalently altered HP1α dynamics were, however, observed in the elutriated G1 fraction of E14-HP1α-GFP cells co-stained with anti-H3S10Ph and EdU (to allow contaminating S phase cells to be excluded from the analysis), indicating that the cause of HP1α exclusion from heterochromatin was not always dependent on the presence of precociously loaded H3S10Ph.
Differences in the expression of cell cycle regulators in ESCs as compared with somatic cells have been reported. Geminin, for example, is restricted to G2 in somatic cells, yet is expressed throughout the cell cycle in ESCs.Citation9 Here, the observed phosphorylation of H3S10 during early S phase in ESCs indicates that additional cell-cycle-associated modifications may also be differently regulated in ESCs. We previously reported that ESCs in S phase/G2 were more efficient at reprogramming somatic cells in heterokaryons (formed by fusing ESCs with somatic cells), and that the early induction of replication in the somatic nucleus within the heterokaryon was required for successful reprogramming.Citation1 Here, we additionally show that the somatic nucleus undergoing reprogramming within a heterokaryon acquires some of the unusual characteristics of S phase shown by ESCs, including additional sites of nucleotide incorporation during stage 3 of S phase. This may be important, as it has been suggested that reversion of the progression of S phase to a more ESC-like state may be a barrier to reprogramming.Citation23
It has been reported that the structure of heterochromatin in ESCs is less compact and more dynamic and accessible than that in somatic cells.Citation37,Citation40,Citation41 In E14 cells examined by confocal microscopy, centromeric heterochromatin appeared to be distributed in a similar way to that seen in somatic cells (i.e., clusters are scattered along the nuclear periphery and around the nucleolus during interphase). The clustered centromeric and peri-centromeric heterochromatin appeared DAPI-intense and contained high levels of H3K9Me3, the recruiter of HP1.Citation2 We found a significant proportion of cells (approximately 10%) containing low levels of HP1α and, in some cases, no enrichment of HP1α at some or all of their H3K9Me3-positive heterochromatic foci. A similar proportion of such cells were observed at each stage of the cell cycle, in both E14 and E14-HP1α-GFP cells, suggesting that the lack of HP1α could not be attributed to either its displacement in G2, nor mis-expression of the HP1α-GFP construct. In addition, approximately 20% of ESCs show intense foci of H3K27Me3 associated with heterochromatin. H3K27Me3 is associated with facultative heterochromatinCitation38 in somatic cells and with bivalent chromatinCitation39 in ESCs, and appears as a fine “haze” distributed throughout the nucleoplasm in mouse B cells. We observed no differences in the apparent distributions of most euchromatin-associated histone modifications,Citation42 such as H3K9Ac or H4K16Ac, in mouse B cells and ESCs. Taken together, these observations indicate selective differences in the structure and behavior of heterochromatin in ESCs that are acquired by somatic cells upon pluripotent reprogramming. Our studies also exemplify how counterflow centrifugal elutriation can be used to investigate cell cycle biology in ESCs in a way that doesn’t precipitate their differentiation or death. This is of increasing importance if we are to decipher how the epigenome of ESCs is regulated during cell cycle progression, and which features of the epigenome are critical for the successful dominant reprogramming of somatic cells by ESCs.
Materials and Methods
Cell culture
E14Tg2A Hprt−/− mouse ESCs (E14) and Abelson-transformed mouse B cells were cultured as described previously.Citation24
Construction of the E14-HP1α-GFP cell line
HP1α-GFP cDNA was cloned into chicken β-actin promoter-driven expression vector pCAGIPuroCitation43 and was used to stably transfect E14 murine ES cells using Mouse ES Cell Nucleofector® Kit (Lonza). Puromycin (1 µg/ml) was added in ES cell media after 24 h after transfection, and stable colonies were obtained following 6–7 d of selection.
Counterflow centrifugal elutriation
Counterflow centrifugal elutriation was performed using JE-5.0 elutriator system (Beckman Coulter) in combination with MasterFlex peristaltic pump (Cole-Parmer Instrument), as described.Citation1,Citation19
Immunofluorescence, FISH, EdU, and BrdU detection
Immunofluorescence analysisCitation44 was performed using rabbit polyclonal to H3K9me3 (1:200, Upstate Biotechnology 07–442), H3K27me3 (1:100, Upstate Biotechnology 05–851), H3K9Ac (1:100, Upstate Biotechnology 06–942), H4K16Ac (1:100, Millipore 07–329), Nanog (Cosmo-Bio REC RCAB0001P), mouse H3S10Ph (1:200, Millipore 06–570) and HP1α (1:100, Chemicon MAB3584), and secondary anti-rabbit or anti-mouse AlexaFluor antibodies at 1:400, Molecular Probes). BrdU incorporation and detection was performed in low-light conditions as described.Citation20 Heterokaryons were grown on gelatinized coverslips for 1 d, pulse labeled with 100 μM BrdU added to the media for 45 min, and fixed with 2% paraformaldehyde in PBS for 20 min at room temperature. Blocking and washing was performed as described.Citation44 BrdU-FITC (1:4, Becton-Dickinson 347583) was applied for 30 min before post-fixation with EGS, final washes, and mounting in Vector shield containing 0.5 mg/ml DAPI (Sigma D9542). EdU (100 μM) was incorporated as a 45 min pulse label before cell fixation, and the Click-iT kit (Invitrogen) was used for EdU detection according to manufacturer’s instructions. Coverslips were subsequently washed with PBS, mounted in Vector Shield containing 0.5 mg/ml DAPI and were stored in the dark at 4 °C.
Abbreviations: | ||
ESC | = | embryonic stem cell |
BrdU | = | 5-Bromo-2’-deoxyuridine |
EdU | = | 5-Ethynyl-2’-deoxyuridine |
PI | = | propidium iodide |
DAPI | = | 4’,6-Diamidino-2-phenylindole dihydrochloride |
Additional material
Download Zip (223.8 KB)Disclosure of Potential Conflicts of Interest
No potential conflicts of interest were disclosed.
Acknowledgments
This work was funded by the Medical Research Council, UK (KEB, HB, AGF) and the European Research Council (AGF and JS-R). We thank Bas van Dijk for his contribution to the experimental work.
Supplemental Materials
Supplemental materials may be found here: www.landesbioscience.com/journals/cc/article/26223
References
- Tsubouchi T, Soza-Ried J, Brown K, Piccolo FM, Cantone I, Landeira D, Bagci H, Hochegger H, Merkenschlager M, Fisher AG. DNA synthesis is required for reprogramming mediated by stem cell fusion. Cell 2013; 152:873 - 83; http://dx.doi.org/10.1016/j.cell.2013.01.012; PMID: 23415233
- Wreggett KA, Hill F, James PS, Hutchings A, Butcher GW, Singh PB. A mammalian homologue of Drosophila heterochromatin protein 1 (HP1) is a component of constitutive heterochromatin. Cytogenet Cell Genet 1994; 66:99 - 103; http://dx.doi.org/10.1159/000133676; PMID: 8287692
- Takebayashi S-I, Dileep V, Ryba T, Dennis JH, Gilbert DM. Chromatin-interaction compartment switch at developmentally regulated chromosomal domains reveals an unusual principle of chromatin folding. Proc Natl Acad Sci U S A 2012; 109:12574 - 9; http://dx.doi.org/10.1073/pnas.1207185109; PMID: 22807480
- Fussner E, Ahmed K, Dehghani H, Strauss M, Bazett-Jones DP. Changes in chromatin fibre density as a marker for pluripotency. Cold Spring Harb Symp Quant Biol 2011; 75:245 - 9; http://dx.doi.org/10.1101/sqb.2010.75.012
- White J, Dalton S. Cell cycle control of embryonic stem cells. Stem Cell Rev 2005; 1:131 - 8; http://dx.doi.org/10.1385/SCR:1:2:131; PMID: 17142847
- Tsubouchi T, Fisher AG. Reprogramming and the pluripotent stem cell cycle. Curr Top Dev Biol 2013; 104:223 - 41; http://dx.doi.org/10.1016/B978-0-12-416027-9.00007-3; PMID: 23587243
- Hindley C, Philpott A. The cell cycle and pluripotency. Biochem J 2013; 451:135 - 43; http://dx.doi.org/10.1042/BJ20121627; PMID: 23535166
- Ghule PN, Medina R, Lengner CJ, Mandeville M, Qiao M, Dominski Z, Lian JB, Stein JL, van Wijnen AJ, Stein GS. Reprogramming the pluripotent cell cycle: restoration of an abbreviated G1 phase in human induced pluripotent stem (iPS) cells. J Cell Physiol 2011; 226:1149 - 56; http://dx.doi.org/10.1002/jcp.22440; PMID: 20945438
- Yang VS, Carter SA, Hyland SJ, Tachibana-Konwalski K, Laskey RA, Gonzalez MA. Geminin escapes degradation in G1 of mouse pluripotent cells and mediates the expression of Oct4, Sox2, and Nanog. Curr Biol 2011; 21:692 - 9; http://dx.doi.org/10.1016/j.cub.2011.03.026; PMID: 21497086
- Stead E, White J, Faast R, Conn S, Goldstone S, Rathjen J, Dhingra U, Rathjen P, Walker D, Dalton S. Pluripotent cell division cycles are driven by ectopic Cdk2, cyclin A/E and E2F activities. Oncogene 2002; 21:8320 - 33; http://dx.doi.org/10.1038/sj.onc.1206015; PMID: 12447695
- Koledova Z, Kafkova LR, Calabkova L, Krystof V, Dolezel P, Divoky V. Cdk2 inhibition prolongs G1 phase progression in mouse embryonic stem cells. Stem Cells Dev 2010; 19:181 - 94; http://dx.doi.org/10.1089/scd.2009.0065; PMID: 19737069
- Calegari F, Huttner WB. An inhibition of cyclin-dependent kinases that lengthens, but does not arrest, neuroepithelial cell cycle induces premature neurogenesis. J Cell Sci 2003; 116:4947 - 55; http://dx.doi.org/10.1242/jcs.00825; PMID: 14625388
- Bar-On O, Shapira M, Skorecki K, Hershko A, Hershko DD. Regulation of APC/C (Cdh1) ubiquitin ligase in differentiation of human embryonic stem cells. Cell Cycle 2010; 9:1986 - 9; http://dx.doi.org/10.4161/cc.9.10.11727; PMID: 20473026
- Calder A, Roth-Albin I, Bhatia S, Pilquil C, Lee JH, Bhatia M, Levadoux-Martin M, McNicol J, Russell J, Collins T, et al. Lengthened G1 phase indicates differentiation status in human embryonic stem cells. Stem Cells Dev 2013; 22:279 - 95; http://dx.doi.org/10.1089/scd.2012.0168; PMID: 22827698
- Koledova Z, Krämer A, Kafkova LR, Divoky V. Cell-cycle regulation in embryonic stem cells: centrosomal decisions on self-renewal. Stem Cells Dev 2010; 19:1663 - 78; http://dx.doi.org/10.1089/scd.2010.0136; PMID: 20594031
- Orford KW, Scadden DT. Deconstructing stem cell self-renewal: genetic insights into cell-cycle regulation. Nat Rev Genet 2008; 9:115 - 28; http://dx.doi.org/10.1038/nrg2269; PMID: 18202695
- Ruiz S, Panopoulos AD, Herrerías A, Bissig KD, Lutz M, Berggren WT, Verma IM, Izpisua Belmonte JC. A high proliferation rate is required for cell reprogramming and maintenance of human embryonic stem cell identity. Curr Biol 2011; 21:45 - 52; http://dx.doi.org/10.1016/j.cub.2010.11.049; PMID: 21167714
- Singh AM, Dalton S. The cell cycle and Myc intersect with mechanisms that regulate pluripotency and reprogramming. Cell Stem Cell 2009; 5:141 - 9; http://dx.doi.org/10.1016/j.stem.2009.07.003; PMID: 19664987
- Banfalvi G. Cell cycle synchronization of animal cells and nuclei by centrifugal elutriation. Nat Protoc 2008; 3:663 - 73; http://dx.doi.org/10.1038/nprot.2008.34; PMID: 18388949
- Azuara V, Brown KE, Williams RRE, Webb N, Dillon N, Festenstein R, Buckle V, Merkenschlager M, Fisher AG. Heritable gene silencing in lymphocytes delays chromatid resolution without affecting the timing of DNA replication. Nat Cell Biol 2003; 5:668 - 74; http://dx.doi.org/10.1038/ncb1006; PMID: 12833066
- O’Keefe RT, Henderson SC, Spector DL. Dynamic organization of DNA replication in mammalian cell nuclei: spatially and temporally defined replication of chromosome-specific alpha-satellite DNA sequences. J Cell Biol 1992; 116:1095 - 110; http://dx.doi.org/10.1083/jcb.116.5.1095; PMID: 1740468
- Hiratani I, Ryba T, Itoh M, Rathjen J, Kulik M, Papp B, Fussner E, Bazett-Jones DP, Plath K, Dalton S, et al. Genome-wide dynamics of replication timing revealed by in vitro models of mouse embryogenesis. Genome Res 2010; 20:155 - 69; http://dx.doi.org/10.1101/gr.099796.109; PMID: 19952138
- Ryba T, Hiratani I, Sasaki T, Battaglia D, Kulik M, Zhang J, Dalton S, Gilbert DM. Replication timing: a fingerprint for cell identity and pluripotency. PLoS Comput Biol 2011; 7:e1002225; http://dx.doi.org/10.1371/journal.pcbi.1002225; PMID: 22028635
- Pereira CF, Terranova R, Ryan NK, Santos J, Morris KJ, Cui W, Merkenschlager M, Fisher AG. Heterokaryon-based reprogramming of human B lymphocytes for pluripotency requires Oct4 but not Sox2. PLoS Genet 2008; 4:e1000170; http://dx.doi.org/10.1371/journal.pgen.1000170; PMID: 18773085
- Hendzel MJ, Wei Y, Mancini MA, Van Hooser A, Ranalli T, Brinkley BR, Bazett-Jones DP, Allis CD. Mitosis-specific phosphorylation of histone H3 initiates primarily within pericentromeric heterochromatin during G2 and spreads in an ordered fashion coincident with mitotic chromosome condensation. Chromosoma 1997; 106:348 - 60; http://dx.doi.org/10.1007/s004120050256; PMID: 9362543
- Jacobs SA, Taverna SD, Zhang Y, Briggs SD, Li J, Eissenberg JC, Allis CD, Khorasanizadeh S. Specificity of the HP1 chromo domain for the methylated N-terminus of histone H3. EMBO J 2001; 20:5232 - 41; http://dx.doi.org/10.1093/emboj/20.18.5232; PMID: 11566886
- Nakayama J, Rice JC, Strahl BD, Allis CD, Grewal SI. Role of histone H3 lysine 9 methylation in epigenetic control of heterochromatin assembly. Science 2001; 292:110 - 3; http://dx.doi.org/10.1126/science.1060118; PMID: 11283354
- Bannister AJ, Zegerman P, Partridge JF, Miska EA, Thomas JO, Allshire RC, Kouzarides T. Selective recognition of methylated lysine 9 on histone H3 by the HP1 chromo domain. Nature 2001; 410:120 - 4; http://dx.doi.org/10.1038/35065138; PMID: 11242054
- Grzenda A, Leonard P, Seo S, Mathison AJ, Urrutia G, Calvo E, Iovanna J, Urrutia R, Lomberk G. Functional impact of Aurora A-mediated phosphorylation of HP1γ at serine 83 during cell cycle progression. Epigenet Chromatin. 2013; 6:21; http://dx.doi.org/10.1186/1756-8935-6-21
- Fischle W, Tseng BS, Dormann HL, Ueberheide BM, Garcia BA, Shabanowitz J, Hunt DF, Funabiki H, Allis CD. Regulation of HP1-chromatin binding by histone H3 methylation and phosphorylation. Nature 2005; 438:1116 - 22; http://dx.doi.org/10.1038/nature04219; PMID: 16222246
- Hirota T, Lipp JJ, Toh BH, Peters JM. Histone H3 serine 10 phosphorylation by Aurora B causes HP1 dissociation from heterochromatin. Nature 2005; 438:1176 - 80; http://dx.doi.org/10.1038/nature04254; PMID: 16222244
- Terada Y. Aurora-B/AIM-1 regulates the dynamic behavior of HP1alpha at the G2-M transition. Mol Biol Cell 2006; 17:3232 - 41; http://dx.doi.org/10.1091/mbc.E05-09-0906; PMID: 16687578
- Dormann HL, Tseng BS, Allis CD, Funabiki H, Fischle W. Dynamic regulation of effector protein binding to histone modifications: the biology of HP1 switching. Cell Cycle 2006; 5:2842 - 51; http://dx.doi.org/10.4161/cc.5.24.3540; PMID: 17172865
- Lomberk G, Bensi D, Fernandez-Zapico ME, Urrutia R. Evidence for the existence of an HP1-mediated subcode within the histone code. Nat Cell Biol 2006; 8:407 - 15; http://dx.doi.org/10.1038/ncb1383; PMID: 16531993
- Mitsui K, Tokuzawa Y, Itoh H, Segawa K, Murakami M, Takahashi K, Maruyama M, Maeda M, Yamanaka S. The homeoprotein Nanog is required for maintenance of pluripotency in mouse epiblast and ES cells. Cell 2003; 113:631 - 42; http://dx.doi.org/10.1016/S0092-8674(03)00393-3; PMID: 12787504
- Chambers I, Colby D, Robertson M, Nichols J, Lee S, Tweedie S, Smith A. Functional expression cloning of Nanog, a pluripotency sustaining factor in embryonic stem cells. Cell 2003; 113:643 - 55; http://dx.doi.org/10.1016/S0092-8674(03)00392-1; PMID: 12787505
- Ahmed K, Dehghani H, Rugg-Gunn P, Fussner E, Rossant J, Bazett-Jones DP. Global chromatin architecture reflects pluripotency and lineage commitment in the early mouse embryo. PLoS One 2010; 5:e10531; http://dx.doi.org/10.1371/journal.pone.0010531; PMID: 20479880
- Rougeulle C, Chaumeil J, Sarma K, Allis CD, Reinberg D, Avner P, Heard E. Differential histone H3 Lys-9 and Lys-27 methylation profiles on the X chromosome. Mol Cell Biol 2004; 24:5475 - 84; http://dx.doi.org/10.1128/MCB.24.12.5475-5484.2004; PMID: 15169908
- Jørgensen HF, Giadrossi S, Casanova M, Endoh M, Koseki H, Brockdorff N, Fisher AG. Stem cells primed for action: polycomb repressive complexes restrain the expression of lineage-specific regulators in embryonic stem cells. Cell Cycle 2006; 5:1411 - 4; http://dx.doi.org/10.4161/cc.5.13.2927; PMID: 16855402
- Meshorer E, Misteli T. Chromatin in pluripotent embryonic stem cells and differentiation. Nat Rev Mol Cell Biol 2006; 7:540 - 6; http://dx.doi.org/10.1038/nrm1938; PMID: 16723974
- Meshorer E, Yellajoshula D, George E, Scambler PJ, Brown DT, Misteli T. Hyperdynamic plasticity of chromatin proteins in pluripotent embryonic stem cells. Dev Cell 2006; 10:105 - 16; http://dx.doi.org/10.1016/j.devcel.2005.10.017; PMID: 16399082
- Struhl K. Histone acetylation and transcriptional regulatory mechanisms. Genes Dev 1998; 12:599 - 606; http://dx.doi.org/10.1101/gad.12.5.599; PMID: 9499396
- Niwa H, Masui S, Chambers I, Smith AG, Miyazaki J. Phenotypic complementation establishes requirements for specific POU domain and generic transactivation function of Oct-3/4 in embryonic stem cells. Mol Cell Biol 2002; 22:1526 - 36; http://dx.doi.org/10.1128/MCB.22.5.1526-1536.2002; PMID: 11839818
- Terranova R, Pereira CF, Du Roure C, Merkenschlager M, Fisher AG. Acquisition and extinction of gene expression programs are separable events in heterokaryon reprogramming. J Cell Sci 2006; 119:2065 - 72; http://dx.doi.org/10.1242/jcs.02945; PMID: 16638804