Abstract
Both major forms of diabetes mellitus (DM) involve β-cell destruction and dysfunction. New treatment strategies have focused on replenishing the deficiency of β-cell mass common to both major forms of diabetes by islet transplantation or β-cell regeneration. The pancreas, not the liver, is the ideal organ for islet regeneration, because it is the natural milieu for islets. Since islet mass is known to increase during obesity and pregnancy, the concept of stimulating pancreatic islet regeneration in vivo is both rational and physiologic. This paper proposes a novel approach in which non-viral gene therapy is targeted to pancreatic islets using ultrasound targeted microbubble destruction (UTMD) in a non-human primate model (NHP), the baboon. Treated baboons received a gene cocktail comprised of cyclinD2, CDK, and GLP1, which in rats results in robust and durable islet regeneration with normalization of blood glucose, insulin, and C-peptide levels. We were able to generate important preliminary data indicating that gene therapy by UTMD can achieve in vivo normalization of the intravenous (IV) glucose tolerance test (IVGTT) curves in STZ hyperglycemic-induced conscious tethered baboons. Immunohistochemistry clearly demonstrated evidence of islet regeneration and restoration of β-cell mass.
Introduction
There are 1.5 million Americans with type 1 diabetes mellitus (T1DM),Citation1 wherein severe insulin deficiency is caused by >95% reduction in β-cell mass.Citation2,Citation3 Insulin replacement remains standard therapy but does not restore normal glucose homeostasis. Therefore, a major goal in T1DM is restoration of β-cell mass. Two main pathways have been proposed for expansion of β-cell mass—regeneration of existing β cells or β cell neogenesis.Citation4-Citation7 Recent studiesCitation8,Citation9 showed that cell cycle regulators (cyclinD1/D2) are essential for normal adult β cell and islet growth. Beta-cell proliferation has been induced in isolated islets by adenoviral gene transfer of cyclinD2/CDK4 or cyclinD1/CDK6.Citation10,Citation11 Cell cycle regulation is an integral mechanism for maintaining postnatal β-cell mass.Citation12,Citation13 Unfortunately, β cell replication in T1DM may be limited by severe β-cell deficiency. We previously reported that targeted gene delivery of cyclinD2/CDK4/GLP-1 to the pancreas by ultrasound-targeted microbubble destruction (UTMD) resulted in durable islet regeneration and reversal of diabetes in streptozotocin-induced rats.Citation14 Using an established tether system in baboons,Citation15 we now report results from a pilot study to determine if islet regeneration by UTMD gene therapy is feasible in a primate with close genetic similarity to humans.
Results
and show the morphology of pancreatic islets using triple immunofluorescent staining. Panels A–D show staining with anti-insulin (red), anti-glucagon (green), and dapi (blue) for the cell nucleus. In the normal baboon, the β cells dominate islet structure. Interestingly, many single α cells are scattered throughout the pancreas () and not just around islet periphery as demonstrated in rodents. In DsRed-treated controls, STZ destroyed almost all β cells, but islet-like structures containing large clusters of α cells are seen (). After UTMD pBIP3.1-GLP-1/CyclinD2/CDK4 gene therapy at low dose, the α cell clusters or islets also contained insulin-producing cells or cells co-expressing insulin and glucagon. In high power images (), there are cells co-expressing insulin, glucagon, and amylase, which is consistent with adult pancreatic progenitor cells, and which we have also observed in rodents. In addition, there were isolated insulin-producing cells within the pancreatic parenchyma, particularly along ducts (). After UTMD pBIP3.1-GLP-1/CyclinD2/CDK4 gene therapy at high dose, the appearance of insulin-producing cells within islets was more prominent than the ones with low-dose UTMD administration (). Beta-cell fraction (%) was 1.68%, 0.05%, 0.48%, and 1.05% in normal baboon, DsRed control, low-dose, and high-dose gene therapy, respectively (, bottom). This was associated with restoration of normal IVGTT in the high-dose gene therapy baboon relative to DsRed control baboon (). Also, the IVGTT curves clearly show that blood glucose, C-peptide, and insulin were recovered in the treated baboon, but not in control (). demonstrates that the insulin-producing cells stained positive with Ki-67, demonstrating cellular proliferation. Transgenic DsRed gene showed evidence of tissue-specific expression in both α cells and β cells after UTMD gene delivery (). RT-PCR also showed that DsRed cDNA was abundantly detected in the pancreas and spleen (scanty amounts) but not in liver, kidney, skeletal muscle, or heart (, bottom). Our previous data demonstrated that this insulin gene promoter also worked in α cells; luciferase gene under insulin gene promoter driving had 43% of activities in α cells compared with β cells.Citation21 further shows transgenic GLP-1 gene expressed in pancreatic β cells in UTMD gene therapy baboons.
Figure 1. Confocal microscopic images of insulin (red), glucagon (green), and Dapi (blue). (A) Baboon ID#11692, normal baboon pancreas; (B) Baboon ID#18175, STZ plus UTMD pBIP3.1-DsRed reporter gene; (C) Baboon ID#26104, STZ plus UTMD-pBIP3.1-cyclinD2/CDK4/GLP-1 genes (12 mg of plasmid); (D) Baboon ID#20105, STZ plus UTMD-pBIP3.1-cyclinD2/CDK4/GLP-1 genes (20 mg of plasmid); scale bar is 120 µm. (E) The lower panel displays the percentage of β-cell area in total pancreatic area (%).
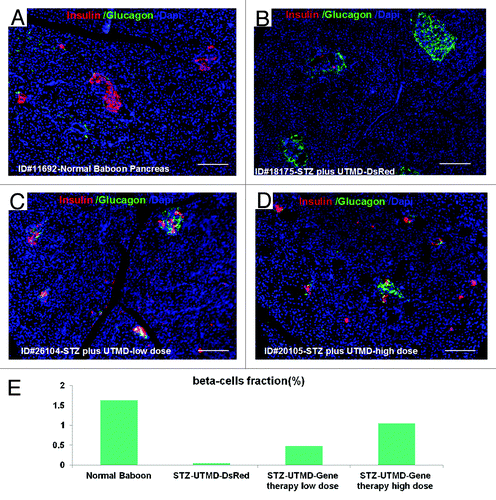
Figure 2. The confocal images of triple staining for insulin, amylase, and glucagon (A) Baboon ID#11692, normal baboon pancreas; (B) Baboon ID#18175, STZ plus UTMD pBIP3.1-DsRed reporter gene; (C) Baboon ID#26104, STZ plus UTMD-pBIP3.1-cyclinD2/CDK4/GLP-1 genes (12 mg of plasmid); (D) Baboon ID#20105, STZ plus UTMD-pBIP3.1-cyclinD2/CDK4/GLP-1 genes (20 mg of plasmid); scale bar is 40 µm.
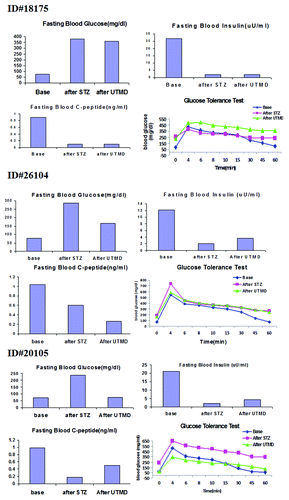
Figure 3. Fasting plasma glucose, insulin, C-peptide concentrations, and plasma glucose concentrations of intravenous glucose tolerance tests (IVGTT).
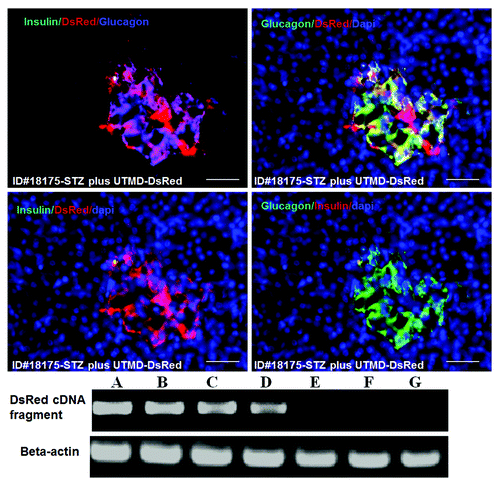
Figure 4. Confocal microscopic images of Ki-67, a marker of proliferation. The high-power images show that insulin-producing cells are proliferating in the pancreas of baboon ID#20105(STZ plus UTMD-pBIP3.1-cyclinD2/CDK4/GLP-1 genes (20 mg of plasmid); scale bar is 25 µm.
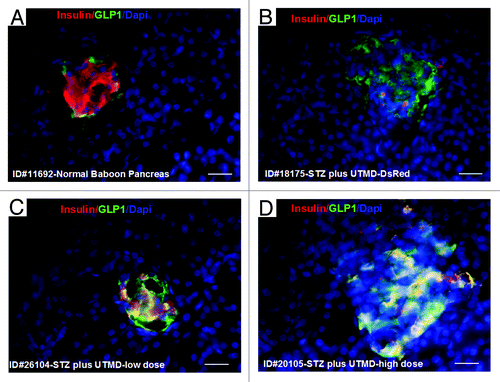
Figure 5. Upper panels are confocal microscopic images showing transgenic expression of DsRed reporter gene in Baboon ID#18175 pancreas. Insulin cells are virtually absent. DsRed colocalizes with glucagon in α-cell clusters/islets. Scale bar is 50 µm. Lower panel is RT-PCR for DsRed cDNA in Baboon ID#18175: (A), pancreatic head; (B), pancreatic middle; (C), pancreatic tail; (D) spleen; (E) liver; (F) heart; (G) skeletal muscle.
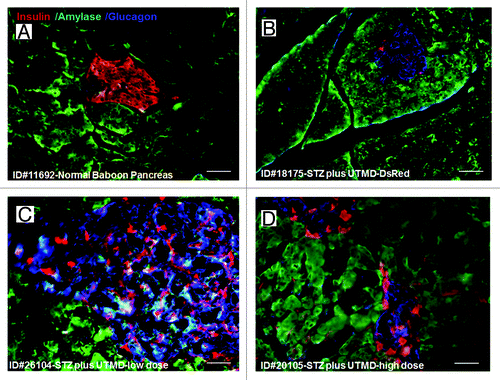
Figure 6. Transgenic expression of GLP1 in pancreatic islets, scale bar is 40 µm. (A) Baboon ID#11692, normal baboon pancreas; (B) Baboon ID#18175, STZ plus UTMD pBIP3.1-DsRed reporter gene; (C) Baboon ID#26104, STZ plus UTMD-pBIP3.1-cyclinD2/CDK4/GLP-1 genes (12 mg of plasmid); (D) Baboon ID#20105, STZ plus UTMD-pBIP3.1-cyclinD2/CDK4/GLP-1 genes (20 mg of plasmid).
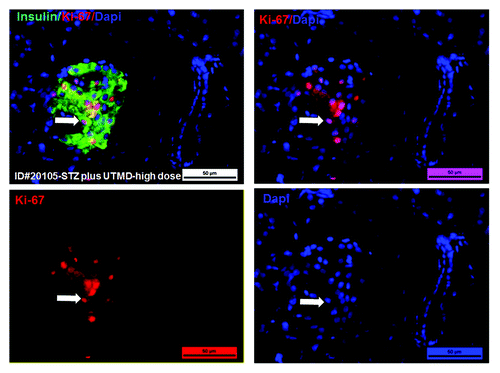
Discussion
We used UTMD to direct gene therapy to the pancreas in order to reproduce the normal physiologic secretion of insulin into the portal circulation, where it acts on the liver to suppress hepatic glucose production without causing systemic hyperinsulinemia. A combination of CyclinD2, CDK4, and GLP-1 genes successfully induced islet regeneration and restoration of β-cell mass. Physiologically, this was accompanied by improvement of the glucose tolerance test in our treated baboons. Although this was designed as a pilot study to test feasibility and acquire preliminary data, to our knowledge it is the first report to demonstrate that gene therapy can successfully reverse STZ induced-hyperglycemia in a non-human primate. Previous studies in rodents from our groupCitation14 and othersCitation22 found that α cells are a resource for recovering β-cell mass. The present study suggests that this occurs in STZ hyperglycemic-induced conscious tethered baboons and, thus, supports the concept that “alpha cells beget beta cells”.Citation23,Citation24 It is not clear how the origin or formation of pancreatic α cell-dominant islets occurs after STZ injection. Recently Accili D and his colleaguesCitation25 reported that adult mature pancreatic β cells were dedifferentiated into pancreatic progenitor-like cells and then proliferated into α cells because of metabolic stress or inflammation. Our data from rodents and nonhuman primates suggest that gene therapy can re-differentiate these α cells into functioning β cells rather than replicating pre-existing β cells.
However, further work is needed to confirm the mechanism and cellular origin underlying islet regeneration in primates. It remains possible that the effects of STZ influence the results and different findings would be found in a naturally occurring form of diabetes. Nevertheless, the results of this pilot study are encouraging and suggest that therapeutic islet regeneration is not merely a phenomenon that occurs in lower order mammals such as rodents.
In conclusion, we were able to achieve islet regeneration through UTMD gene therapy in STZ hyperglycemic-induced conscious tethered baboons, with increase in β-cell mass and improvement of plasma glucose, insulin, and C-peptide without administering viruses. Although targeted gene therapy has been used experimentally to treat diabetes in mice and rats, these models are limited by their small size, brief life span, potential for spontaneous regeneration of the pancreas, and differences in maintenance of glucose metabolism/islet function vs. high-order vertebrates. Thus, the present finding of in vivo re-differentiation of pre-existing α cells into functioning β cells by targeted gene therapy in baboons offers strong potential to be translated to humans.
Materials and Methods
Animal protocols and UTMD
This study was performed in accordance with the recommendations in the Guide for the Care and Use of Laboratory Animals of the National Institutes of Health. The protocol was approved by the Institutional Animal Care and Use Committees of the Texas Biomedical Research Institute and Baylor Research Institute (Assurance Number: 10–4233). The study approval ID is IACUC 1251PC. After an overnight fast (~12 h), each baboon was sedated with ketamine hydrochloride (10 mg/kg i.m.) before arrival in the procedure room. Endotracheal intubation was performed using disposable cuffed tubes (6.5–8.0 mm diameter) under direct laryngoscopic visualization, and all the animals were supported with 98–99.5% FiO2 by a pressure-controlled ventilator, which was adjusted, as necessary, to keep the oxygen saturation >95%. The maintenance of anesthesia consisted of an inhaled isofluorane (0.5–1.5%) and oxygen mix.
We developed a comprehensive standardized protocol to induce diabetes in baboons using STZ. We utilize 4 baboons in this study. One baboon, otherwise healthy (ID 11692), scheduled to have euthanasia, matched with the weight and age of the animals chosen for the study, was opportunistically identified to harvest pancreatic tissue at the time of necropsy to be considered as normal control for pancreas staining. After animal selection and acclimation, 3 baboons were selected (age = 6–16 y and weight = 26–34 kg) based on maintenance of food intake, general attitude toward study technical staff, study veterinarian, and comfort level with presence of technical staff in bay. Animal acclimation to technician presence was undertaken prior to the procedure start date. All selected animals had an arterial and venous tether catheter placed under isofluorane anesthesia. A continuous intravenous saline 0.9% infusion at a rate of 30 ml/kg of body weight/day was administered 48 h before and after STZ (Zanosar) administration to prehydrate the baboons and reduce potential nephrotoxic effects. All baboons received STZ (administered over 5 min) at a dose ranging from 80–150 mg/kg. In the 72 h after STZ induction, frequent blood samples were drawn to measure the plasma glucose concentration, and 50% dextrose was infused at a rate designed to maintain the plasma glucose concentration between 80–125 mg/dl. After 72 h the glucose infusion was stopped, and diabetes was confirmed by the persistence of fasting hyperglycemia. On day 4 following STZ an IVGTT (0.5 mg/kg of 50% dextrose administered over 2 min) was performed, with glucose concentrations measured every 5–10 min for 60 min. A fasting plasma glucose level of >200 mg/dl in combination with stimulated C-peptide levels of less than 0.4 ng/ml on the day of the IVGTT was considered to be indicative of diabetes. All animals became diabetic 3 d after STZ administration. UTMD was performed 12 d after STZ in all animals. We administered a DsRed reporter gene to baboon ID 18175, plasmid DNA containing a low-dose (12 mg of plasmids) gene cocktail with a combination of cyclin D2, CDK4 and GLP-1 to baboon ID 26104, and the same gene cocktail to baboon ID 20105 with a high-dose of plasmisds (20 mg). Euthanasia was performed 21 d after UTMD prior to collecting blood to measure fasting plasma glucose, insulin, C-peptide and performing an IVGTT. Pancreas, liver, spleen, kidney, skeletal muscle, and heart were harvested for pathology and histology studies.
Microbubble solutions were intravenously infused over 5 min via pump (Genie, Kent Scientific). Three-milliliters microbubble solution containing a dosage of 12 mg of DsRed reporter gene plasmid diluted with 3 ml phosphate-buffered solution (PBS) was administered to baboons ID 18175. Three-ml microbubble solution containing a dosage of 12 mg of plasmid diluted with 3 ml phosphate-buffered solution (PBS) was administered to baboons ID 26104. Five-ml microbubble containing a dosage of 20 mg of plasmid diluted with 5 ml PBS were administered to baboon ID 20105. During the infusion, ultrasound was directed to the pancreas using a commercially available ultrasound transducer (S3, Sonos 5500, Philips Ultrasound) with slow manual scanning back and forth from the tail to the head of the pancreas during microbubble perfusion. Ultrasound was applied in ultraharmonic mode (transmit 1.3 MHz/receive 3.6 MHz) at a mechanical index of 1.4. Four bursts of ultrasound were triggered to every fourth end-systole by electrocardiogram using a delay of 45–70 ms after the peak of the R wave. We previously demonstrated that these settings were optimal for plasmid delivery by UTMD using this instrument. Bubble destruction was visually apparent on ultrasound images of the pancreas of all baboons.
Plasmids constructs
Baboon insulin gene promoter construction was performed according to Papio hamadryas insulin gene sequences information.Citation16 Baboon genomic DNA was extracted from Papio hamadryas peripheral blood with a QIAamp Blood kit (Qiagen Inc). Baboon insulin gene promoter primers forward: 5-AGCGCTTAAT GTGGAAAGTG GGCC-3, reversed: 5-CTCGAGGGCA GAAGGACAGT GACC-3. PCR amplification of baboon genomic DNA was performed using forward and reverse primers containing either Eco47III-XhoI restriction site, respectively. The PCR products were subcloned into the pDsRed1–1 reporter vector (Clonetech). Human cyclinD2Citation17 and CDK4Citation18 cDNA (Addgene Inc) and GLP-1 cDNA with furin cutting sites (method of Kim, et al.Citation19) were subcloned to the baboon insulin gene promoter driving vector. Plasmid digestion, subcloning, ligation, isolation, and purification were performed by standard procedures and sequenced to confirm that no artifactual mutations were present.
RNA isolation and RT–PCR analysis
Total RNA was isolated from 100 mg of harvested pancreas using RNA purification kit (Qiagen), according to the manufacturer’s instructions and reverse-transcribed using Superscript III RT (Invitrogen). RT–PCR analysis was performed on an ABI 7700 Sequence Detector (Applied Biosystems). CDNA DsRed PCR primer sequences were forward: 5′-ATGCGCTTCA AGGTGCGCAT; reverse: 5′- AAGGACAGCT TCTTGTA.
Manufacture of plasmid-containing lipid-stabilized microbubbles
Lipid-stabilized microbubbles were prepared, as previously described, in our laboratory.Citation14 Briefly, 3 ml of liposome solution containing DPPC (1,2-dipalmitoyl-sn-glycero-3-phosphatidylcholine, Sigma) 2.5 mg/ml; DPPE (1,2-dipalmitoyl-sn-glycero-3-phosphatidylethanolamine, Sigma) 0.5 mg/ml; and 10% glycerol was mixed with 12 mg of plasmid dissolved in 300 μl of lipefectamine 2000 (Invitrogen). Aliquots of 1 ml of this phospholipid-plasmid solution were placed in 2.0 ml clear vials; the remaining headspace was filled with the perfluoropropane gas (Air Products, Inc). Each vial was incubated at 4 °C for 30 min and then mechanically shaken for 30 s by a dental amalgamator (Vialmix™, Bristol-Myers Squibb Medical Imaging). Three vials microbubble solution were transferred into a 10-ml syringe and diluted with 3 ml of PBS for one baboon IV perfusion.
Immunohistochemistry
Tissue samples were fixed in 4% paraformaldehyde and 20% sucrose overnight at 4 °C, and Cryostat sections 5–8 µm in thickness were further fixed with acetone (−20 °C) for 5 min and quenched for 5 min with10 mM glycine in PBS. Sections were then rinsed in PBS 3 times, and permeabilized with 0.5% Triton X-100 in PBS for 10 min. Sections were blocked with 10% goat serum at 37 °C for 1 h and washed with PBS 3 times. The primary antibodies (guinea pig anti-insulin antibody, 1:100 dilution; rabbit anti-cyclinD2, rabbit anti-CDK4, rabbit anti-GLP-1, rabbit anti- Ki-67 from Abcam Inc, mouse anti-insulin antibody, 1:500 dilution, mouse anti-glucagon, 1:1000, Sigma; rabbit anti-glucagon, 1:500, mouse anti-Amylase, 1:50, mouse anti-C-peptide, 1:250, Santa Cruz Biotech) were added and incubated for 2 h at RT. After washing with PBS 3 times for 5 min, the secondary antibody (Sigma) anti-guinea pig Ig G-conjugated with Texas Red; anti-mouse IgG conjugated with FITC; anti-rabbit IgG conjugated with Cy5) (1:500 dilution in block solution) was added. Dapi (1:5000 dilution, Invitrogen).
Beta-cell fraction
Beta-cell fraction was evaluated by the method of Terauchi, et al.Citation20 Sections of baboon pancreas were immunostained with monoclonal guinea pig anti-insulin (1:100 dilution) and a second antibody, goat anti-guinea pig IgG-FITC (1:250 dilution). The total areas of islet β cells and whole pancreas in slides were measured using NIH ImageJ software (NIH). The areas of β cells and pancreas were determined by counting 6 consecutive slides each piece and total 3 pieces (head, middle, and tail) of whole baboon pancreas tissue in respective groups.
Data analysis
Data analysis was performed with Statview software (SAS). The results are expressed as the mean ± one standard deviation. Differences were analyzed by repeated measures ANOVA with Fisher post-hoc test and considered significant at P < 0.05.
Disclosure of Potential Conflicts of Interest
No potential conflicts of interest relevant to this article were reported.
Acknowledgments
This work was supported by the Mark Shepherd endowment of the Baylor Foundation. This study was conducted using facilities constructed with support from the Research Facilities Improvement Program under grant number C06 RR (numbers 014578, 013556, 015456, 017515) from the National Center for Research Resources and with support from National Institutes of Health grants PO1 HL028972 and P51 RR013986.
P.A.G., C.S., B.R.A., C.A.G., and D.R.A. planned baboon experiments, which were performed by C.S., B.R.A., R.B., V.V.S., F.P.A., G.N.E.J., C.A.H.E., C.J., and H.P.T. The manuscript was written by P.A.G., C.S., and B.R.A.
P.A.G. serves as the guarantor of this work.
References
- Huang ES, Basu A, O’Grady M, Capretta JC. Projecting the future diabetes population size and related costs for the U.S. Diabetes Care 2009; 32:2225 - 9; http://dx.doi.org/10.2337/dc09-0459; PMID: 19940225
- Butler PC, Meier JJ, Butler AE, Bhushan A. The replication of β cells in normal physiology, in disease and for therapy. Nat Clin Pract Endocrinol Metab 2007; 3:758 - 68; http://dx.doi.org/10.1038/ncpendmet0647; PMID: 17955017
- Meier JJ. Beta cell mass in diabetes: a realistic therapeutic target?. Diabetologia 2008; 51:703 - 13; http://dx.doi.org/10.1007/s00125-008-0936-9; PMID: 18317728
- Bonner-Weir S, Weir GC. New sources of pancreatic beta-cells. Nat Biotechnol 2005; 23:857 - 61; http://dx.doi.org/10.1038/nbt1115; PMID: 16003374
- Dor Y, Brown J, Martinez OI, Melton DA. Adult pancreatic beta-cells are formed by self-duplication rather than stem-cell differentiation. Nature 2004; 429:41 - 6; http://dx.doi.org/10.1038/nature02520; PMID: 15129273
- Zhou Q, Brown J, Kanarek A, Rajagopal J, Melton DA. In vivo reprogramming of adult pancreatic exocrine cells to beta-cells. Nature 2008; 455:627 - 32; http://dx.doi.org/10.1038/nature07314; PMID: 18754011
- Levine F, Itkin-Ansari P. β-cell Regeneration: neogenesis, replication or both?. J Mol Med (Berl) 2008; 86:247 - 58; http://dx.doi.org/10.1007/s00109-007-0259-1; PMID: 17922102
- Kushner JA. Beta-cell growth: an unusual paradigm of organogenesis that is cyclin D2/Cdk4 dependent. Cell Cycle 2006; 5:234 - 7; http://dx.doi.org/10.4161/cc.5.3.2399; PMID: 16410729
- He LM, Sartori DJ, Teta M, Opare-Addo LM, Rankin MM, Long SY, Diehl JA, Kushner JA. Cyclin D2 protein stability is regulated in pancreatic beta-cells. Mol Endocrinol 2009; 23:1865 - 75; http://dx.doi.org/10.1210/me.2009-0057; PMID: 19628581
- Cozar-Castellano I, Takane KK, Bottino R, Balamurugan AN, Stewart AF. Induction of beta-cell proliferation and retinoblastoma protein phosphorylation in rat and human islets using adenovirus-mediated transfer of cyclin-dependent kinase-4 and cyclin D1. Diabetes 2004; 53:149 - 59; http://dx.doi.org/10.2337/diabetes.53.1.149; PMID: 14693709
- Fiaschi-Taesch N, Bigatel TA, Sicari B, Takane KK, Salim F, Velazquez-Garcia S, Harb G, Selk K, Cozar-Castellano I, Stewart AF. Survey of the human pancreatic beta-cell G1/S proteome reveals a potential therapeutic role for cdk-6 and cyclin D1 in enhancing human beta-cell replication and function in vivo. Diabetes 2009; 58:882 - 93; http://dx.doi.org/10.2337/db08-0631; PMID: 19136653
- Georgia S, Bhushan A. Beta cell replication is the primary mechanism for maintaining postnatal beta cell mass. J Clin Invest 2004; 114:963 - 8; http://dx.doi.org/10.1172/JCI200422098; PMID: 15467835
- Zhong L, Georgia S, Tschen SI, Nakayama K, Nakayama K, Bhushan A. Essential role of Skp2-mediated p27 degradation in growth and adaptive expansion of pancreatic beta cells. J Clin Invest 2007; 117:2869 - 76; http://dx.doi.org/10.1172/JCI32198; PMID: 17823659
- Chen S, Shimoda M, Chen J, Matsumoto S, Grayburn PA. Transient overexpression of cyclin D2/CDK4/GLP1 genes induces proliferation and differentiation of adult pancreatic progenitors and mediates islet regeneration. Cell Cycle 2012; 11:695 - 705; http://dx.doi.org/10.4161/cc.11.4.19120; PMID: 22373529
- Coelho AMJ Jr., Carey KD. A social tethering system for nonhuman primates used in laboratory research. Lab Anim Sci 1990; 40:388 - 94; PMID: 2166866
- Chavez AO, Lopez-Alvarenga JC, Tejero ME, Triplitt C, Bastarrachea RA, Sriwijitkamol A, Tantiwong P, Voruganti VS, Musi N, Comuzzie AG, et al. Physiological and molecular determinants of insulin action in the baboon. Diabetes 2008; 57:899 - 908; http://dx.doi.org/10.2337/db07-0790; PMID: 18174524
- Baker GL, Landis MW, Hinds PW. Multiple functions of D-type cyclins can antagonize pRb-mediated suppression of proliferation. Cell Cycle 2005; 4:330 - 8; http://dx.doi.org/10.4161/cc.4.2.1485; PMID: 15684604
- van den Heuvel S, Harlow E. Distinct roles for cyclin-dependent kinases in cell cycle control. Science 1993; 262:2050 - 4; http://dx.doi.org/10.1126/science.8266103; PMID: 8266103
- Choi S, Oh S, Lee M, Kim SW. Glucagon-like peptide-1 plasmid construction and delivery for the treatment of type 2 diabetes. Mol Ther 2005; 12:885 - 91; http://dx.doi.org/10.1016/j.ymthe.2005.03.039; PMID: 16039908
- Terauchi Y, Takamoto I, Kubota N, Matsui J, Suzuki R, Komeda K, Hara A, Toyoda Y, Miwa I, Aizawa S, et al. Glucokinase and IRS-2 are required for compensatory β cell hyperplasia in response to high-fat diet-induced insulin resistance. J Clin Invest 2007; 117:246 - 57; http://dx.doi.org/10.1172/JCI17645; PMID: 17200721
- Chai R, Chen S, Ding J, Grayburn PA. Efficient, glucose responsive and islet-specific transgene expression by a modified rat insulin promoter. Gene Ther 2009; 16:1202 - 9; http://dx.doi.org/10.1038/gt.2009.114; PMID: 19727136
- Collombat P, Xu X, Ravassard P, Sosa-Pineda B, Dussaud S, Billestrup N, Madsen OD, Serup P, Heimberg H, Mansouri A. The ectopic expression of Pax4 in the mouse pancreas converts progenitor cells into alpha and subsequently beta cells. Cell 2009; 138:449 - 62; http://dx.doi.org/10.1016/j.cell.2009.05.035; PMID: 19665969
- Habener JF, Stanojevic V. Alpha cells come of age. Trends Endocrinol Metab 2013; 24:153 - 63; http://dx.doi.org/10.1016/j.tem.2012.10.009; PMID: 23260869
- Habener JF, Stanojevic V. α-cell role in β-cell generation and regeneration. Islets 2012; 4:188 - 98; http://dx.doi.org/10.4161/isl.20500; PMID: 22847495
- Talchai C, Xuan S, Lin HV, Sussel L, Accili D. Pancreatic β cell dedifferentiation as a mechanism of diabetic β cell failure. Cell 2012; 150:1223 - 34; http://dx.doi.org/10.1016/j.cell.2012.07.029; PMID: 22980982