Abstract
Retinal ganglion cell (RGC) degeneration is an important cause of visual impairment, and results in part from microglia-mediated inflammation. Numerous experimental studies have focused on identifying drug targets to rescue these neurons. We recently showed that KV1.1 and KV1.3 channels are expressed in adult rat RGCs and that siRNA-mediated knockdown of either channel reduces RGC death after optic nerve transection. Earlier we found that KV1.3 channels also contribute to microglial activation and neurotoxicity; raising the possibility that these channels contribute to neurodegeneration through direct roles in RGCs and through inflammatory mechanisms. Here, RGC survival was increased by combined siRNA-mediated knockdown of KV1.1 and KV1.3 in RGCs, but survival was much greater when knockdown of either channel was combined with intraocular injection of a KV1.3 channel blocker (agitoxin-2 or margatoxin). After axotomy, increased expression of several inflammation-related molecules preceded RGC loss and, consistent with a dual mechanism, their expression was differentially affected when channel knockdown in RGCs was combined with KV1.3 blocker injection. KV1.3 blockers reduced activation of retinal microglia and their tight apposition along RGC axon fascicles after axotomy, but did not prevent their migration from the inner plexiform to the damaged ganglion cell layer. Expression of several growth factors increased after axotomy; and again, there were differences following blocker injection compared with RGC-selective channel knockdown. These results provide evidence that KV1.3 channels play important roles in apoptotic degeneration of adult RGCs through cell-autonomous mechanisms mediated by channels in the neurons, and non-autonomous mechanisms mediated by microglia and inflammation.
Introduction
Information is encoded by retinal ganglion cells (RGCs) before transmission to higher brain centers. RGC death underlies several important visual neuropathies (e.g., glaucoma, diabetic retinopathy) and is thus an important therapeutic target. The optic nerve transection model in adult rats has been used extensively to assess the potential of targeting growth factors and apoptosis-related molecules (reviewed in ref. Citation1–Citation4). Using this model, we recently showed that depleting either KV1.1 or KV1.3 channels from RGCs by injecting channel-specific siRNAs into the cut optic nerve stump greatly reduced RGC degeneration.Citation5 While this approach ensures that only the channels in RGCs are affected, it is not clinically feasible. In contrast, when drugs are injected into the vitreous chamber of the eye, they exit by hydraulic and vascular clearance, allowing sustained drug entry into the retina and actions on multiple cell types.Citation6
Inflammatory processes associated with activated microglia are important contributors to RGC death.Citation7–Citation9 While microglia are in a relatively ‘resting’ state in the healthy adult retina, they become ‘activated’ following damage by mechanical injury, ischemia, infection or in autoimmune disorders. Microglial activation is complex; some functions such as phagocytosis and production of growth factors can contribute to repair after CNS injury, while others (e.g., overproduction of pro-inflammatory and oxidative molecules) can exacerbate damage. Consequently, microglial activation is an important, albeit complicated target for reducing neurodegeneration, including RGC loss in retinal neuropathies.
One potential therapeutic target is KV1.3; a voltage-gated K+ channel that is highly expressed in microglia, where it contributes to superoxide production and their neurotoxic capacity in vitro.Citation10 KV1.3 channels are also expressed in RGCs, and we recently found that siRNA-mediated depletion of KV1.3 (or KV1.1) from RGCs substantially increases their survival after optic nerve transection.Citation5 KV1.1 knockdown increased the anti-apoptotic gene, Bcl-XL, while KV1.3 knockdown reduced the pro-apoptotic genes, caspase-3, caspase-9 and Bad. The present study was designed to address the possibility that RGC rescue involves a dual mechanism; direct protection of RGCs, and effects on growth factors and inflammation.
Results
Inflammation and the microglial response after optic nerve transection.
illustrates the structure of the healthy adult rat retina (), and the distribution of retinal ganglion cells and microglia before and after optic nerve transection. Microglia were very sparse in the outer retina (e.g., in the outer plexiform layer; ), and were absent from the outer nuclear and inner nuclear layers (not shown). The inner plexiform layer () contained a dense array of microglia with the highly ramified morphology typical of resting cells. Immediately below the ganglion cell layer (), ramified microglia were distributed between the many somata of Fluorogold-labeled retinal ganglion cells (RGCs). In contrast, the healthy nerve fiber layer () contained only sparse OX-42 labeled cells with a round or amoeboid morphology. In these images of the healthy retina, numerous RGC cell bodies can be seen, along with several Fluorogold-labeled axon bundles running radially toward the optic disc.
By 14 days after axotomy, the distribution of microglia had dramatically changed (). In retinas that had been injected with saline at the time of axotomy and four days later (controls), microglia had migrated to the nerve fiber layer (NFL). They were less ramified than in the healthy retina, and most were aligned with the fascicles of degenerating RGC axons. Very few Fluorogold-labeled RGCs remained (see below), which is consistent with the time-course of neurodegeneration previously observed in this model.Citation11–Citation13 Phagocytic microglia containing apoptotic Fluorogold labeled RGCs were also clearly visible under these conditions (, inset).
We next examined effects on microglia of two potent KV1.3-blocking peptides (agitoxin-2, AgTx-2; margatoxin, MgTx), which we previously found inhibits microglial activation in vitroCitation10 and RGC degeneration in vivo.Citation5 We note that KV channel blockers are not as selective as siRNA-mediated channel knockdown; AgTx-2 blocks KV1.1 and KV1.3, while MgTx blocks KV1.2 and KV1.3.Citation14 For comparison, we also used α-dendrotoxin (α-DTx), a potent blocker of KV1.2 channels (and less effective blocker of KV1.1 and KV1.3), which failed to inhibit microglial activation or rescue RGCs in our earlier studies.Citation5,Citation10 Consistent with our recent quantitative study,Citation5 the images in show more RGC survival after intraocular injection of either AgTx-2 () or MgTx (). There were several morphological indications of reduced microglial activation; they were more scattered, more ramified and less aligned with the axon bundles. Of note, many microglia were present in the NFL; thus, blocking KV1.3 channels did not prevent microglial migration into the damaged layer, but reduced their association with damaged axons. α-dendrotoxin (α-DTx) did not affect microglial morphological changes or alignment with RGC axon bundles (compare and I). As a further control, AgTx-2 injection into the naive retina did not affect the microglial appearance or distribution (not shown).
Expression of several genes was quantified by real-time RT-PCR to monitor the retinal response to optic nerve transection. Several markers of microglial and astrocyte activation, and neuron remodelling were significantly upregulated at seven days after axotomy (); a time chosen to correspond with the maximal rate of RGC apoptosis in this model.Citation11–Citation13 Indications of microglial activation were the ∼10-fold increase in expression of MHC class II—a molecule that increases when antigen-presenting cells (including microglia) are activated; and ∼2-fold increase in complement receptor 3—a surface receptor for immune complexes that is labeled by the OX-42 antibody. Consistent with the observed constitutive expression of CR3, microglia in both the healthy and damaged retina were labelled with OX-42 (see below). After axotomy, there was a ∼3 fold increase in expression of glial fibrillary acidic protein (GFAP), which is upregulated in reactive astrocytes. The ∼1.7 fold increase in the synaptic protein, synaptophysin, is consistent with an earlier observation that surviving RGCs have expanded dendritic trees.Citation15 Further indications of inflammation () were the increased expression of TNFα and the IL1-receptor antagonist (IL-1ra); and cFos, which can be upregulated by TNFα in the injured CNS.Citation16
KV blockers and RGC-specific knockdown differentially affect growth factor expression.
While RGC-specific channel knockdown addresses the cellautonomous role of KV channels in these neurons, intraocular injection of drugs can affect channels in other cell types. In , transcript expression was quantified for several genes known to be involved in retinal responses to damage.Citation3,Citation17–Citation19 When mRNA levels were monitored in whole-retina samples at seven days (), most axotomy-induced changes were small, but significant increases were seen in nerve growth factor (NGF), brain-derived neurotrophic factor (BDNF), epidermal growth factor (EGF) and ciliary neurotrophic factor (CNTF). As before,Citation5 channel-specific siRNAs were compared with a control siRNA directed against firefly luciferase. The outcome of RGC-specific KV1.1 knockdown differed from KV1.3 knockdown. KV1.3 siRNA treatment decreased expression of glial-derived neurotrophic factor (GDNF) and basic fibroblast growth factor (bFGF; also known as FGF2 or FGFβ), while KV1.1 knockdown decreased EGF and increased CNTF. In addition, effects of the two channel blockers differed from each other () and from channel knockdown in RGCs (compare with ). MgTx significantly increased (bFGF) expression several-fold and decreased neurturin (NRTN) levels, while AgTx-2 did not affect expression of any of the growth factors examined. These disparate effects might be mediated through the additional block of KV1.2 channels by MgTx.
Combining channel knockdown with KV1.3 blockade differentially affects inflammatory gene expression and RGC survival.
Expression of several inflammatory genes () was compared after siRNA-mediated channel knockdown in RGCs alone, versus combined knockdown and intraocular AgTx-2 injection. After KV1.1 knockdown, there was an increase in retinal TNFα, IL-1β and TGFβ regardless of whether AgTx-2 was injected; however, only the combined treatment increased cFos and IL-1ra. KV1.3 knockdown from RGCs did not affect expression of the inflammatory genes; whereas, combining this with AgTx-2 injection increased TNFα, IL-1ra and TGFβ. The most striking change was a 4- to 5-fold increase in expression of the anti-inflammatory molecule, IL-1ra, after intraocular injection of AgTx-2, but no change after cell-specific knockdown of either KV1.1 or KV1.3 from RGCs.
If RGC degeneration involves both cell-autonomous and non-autonomous mechanisms, then additive neuroprotection is expected following combined treatment with a KV1.3 blocker and channel knockdown. Results in show additive or supra-additive rescue of RGCs. For comparison, in the healthy rat retina, RGC densities (cells/mm2; n = 6) were: 2,446 ± 68 (inner eccentricity), 2,501 ± 42 (middle) and 2,208 ± 109 (outer eccentricity). At 14 days after optic nerve transection, fewer than 10% of the RGCs remained; i.e., 198 ± 12 cells/mm2 at the inner eccentricity, 206 ± 14 in the middle and 178 ± 12 at the outer eccentricity. Representative confocal images of surviving RGCs in flatmounted retinas are shown (), along with the summarized RGC densities measured at inner, middle and outer retinal eccentricities (). By 14 days after axotomy, >90% of the RGCs had degenerated if control siRNA was injected into the cut nerve stump ( and E). Of note, all the treatments significantly increased RGC survival. Combined knockdown of KV1.1 and KV1.3 from RGCs ( and E) increased survival ∼3-fold to 600–700 cells/mm2; however, this is only slightly higher than survival following knockdown of either channel alone;Citation5 i.e., ∼550 cells/mm2 after KV1.1 depletion (KV1.1–922 siRNA) and ∼600 cells/mm2 after KV1.3 depletion (KV1.3–1169 siRNA). Much more survival was seen when injection of a KV blocker was combined with either KV1.1 or KV1.3 knockdown. For instance, AgTx-2 injection combined with KV1.1–922 or KV1.3–1169 siRNA () increased RGC survival to ∼1,400 cells/mm2 (); ∼2-fold higher than combined knockdown of both channels. This result is also notable because AgTx-2 blocks KV1.1 and KV1.3 channels, and it demonstrates that AgTx-2 is not just acting on RGCs.
Discussion
There are several salient findings in this study. (i) After optic nerve transection, intraocular injection of agitoxin-2, a potent blocker of KV1.3 channels, reduced microglial activation and expression of several inflammatory genes in the damaged retina. This provides the first evidence that identified KV channels contribute to inflammation in the adult mammalian central nervous system in vivo, and specifically, in the retina. (ii) Retinal expression of several growth factors was upregulated after axotomy. Intraocular injection of MgTx increased retinal b-FGF; whereas, RGC-specific depletion of KV1.3 from RGCs decreased GDNF and b-FGF levels. AgTx-2 injection did not affect growth factor levels. (iii) Injecting AgTx-2 increased cFos, TGFβ, IL-1β, IL-1ra and TNFα beyond any effects of KV1.1 or KV1.3 channel knockdown in RGCs. (iv) Combining siRNA-mediated knockdown of either KV1.1 or KV1.3 with intraocular injection of AgTx-2 or MgTx provided much greater RGC rescue; up to 55% of RGCs survived at 14 days after optic nerve transection. Together with our recent study,Citation5 the present results provide evidence that KV channels are functionally linked to RGC degeneration through two distinct mechanisms: directly through cell-autonomous RGC death, and indirectly through non-neuronal cells, most likely by blocking KV1.3 channels in microglia.
Microglia in the healthy adult mammalian retina are present in a thin, densely packed array in the inner plexiform layerCitation20 (reviewed in ref. Citation7, Citation9, Citation20 and Citation21). Their processes are in close proximity to RGCs and displaced amacrine cells, and their highly ramified morphology is similar to ‘resting’ microglia elsewhere in the healthy CNS. In contrast, the normal ganglion cell layer contains only a small number of non-ramified macrophage-like cells; often called ‘perivascular microglia’. After optic nerve transection, microglia migrate to the ganglion cell layer, where they contribute to RGC degeneration. At seven days after axotomy, microglial activation and inflammation was apparent, as judged by upregulation of complement receptor-3 (CR3), a molecule involved in phagocytosis; MHC class II, which is necessary for antigen presentation; TNFα, a proinflammatory cytokine; IL-1ra, an antiinflammatory protein that antagonizes the effects of IL-1β; and cFos, a transcription factor involved in many intracellular signalling events. TNFα, IL-1β and IL-1ra have been shown to have direct causative or preventative roles in RGC degeneration following injury.Citation4,Citation22–Citation24 Because the entire retina was sampled, detectable changes imply large and/or wide-spread responses. If cell-specific changes occurred, they would be underestimated because microglia, astrocytes and RGCs each contribute a small proportion of total retinal cells. For instance, RGCs comprise only ∼7% of the cells in the healthy retina.Citation25
When microglia activate they undergo dramatic morphological changes; their processes retract, cell bodies appear to enlarge, and they become amoeboid and mobile. Consistent with an earlier study,Citation20 at 14 days after axotomy, microglia were aligned with RGC axon fascicles in the nerve fiber layer, and did not appear to be as ramified as in the healthy retina. We previously found that blocking KV1.3 channels in LPS-activated microglia reduced their ability to produce superoxide and kill neurons in vitro; the signaling pathway was not identified but p38 MAPK activation did not appear to be involved.Citation10 RGC death was reduced after axotomy when AgTx-2 or MgTx was injected into the eye,Citation5 and the microglia remained ramified, much like resting microglia seen in the present study. The blocker profile in both studies suggests that KV1.3 is the main molecular target for reducing RGC degeneration. AgTx-2 blocks KV1.1 and KV1.3 channels, MgTx blocks KV1.3 and KV1.2; but α-DTX, which potently inhibits KV1.2 channels, had no effect.
Together, our two studies show that RGC rescue after injecting AgTx-2 or MgTx is not solely due to channel block in either microglia or RGCs, and might implicate other glial or neuronal cell types. RGC somata contain KV1.1 and KV1.3 proteins, and RGC-specific knockdown of either channel increased survival to ∼25% of normal.Citation5 Depleting both KV1.1 and KV1.3 was about as neuroprotective (700–750 RGCs/mm2 survived; present study) as previously seen after injecting either MgTx (700–750 cells/mm2) or AgTx-2 (∼550 cells/mm2).Citation5 In contrast, combining channel knockdown with injection of either AgTx-2 or MgTx rescued about twice as many RGCs (∼1,400 cells/mm2; present study). Considering that <10% of RGCs survived in untreated retinas at 14 days after axotomy, the survival of ∼60% of RGCs with the most effective combination (AgTx-2 injection, plus knockdown of KV1.1 or KV1.3) is striking.
During the acute phase of inflammation after CNS damage, activated microglia can produce numerous inflammatory molecules (reviewed in ref. Citation8), but the consequences are not fully understood. For instance, TGFβ, IL-1β and TNFα from activated microglia play prominent roles in processes that are thought to contribute to neuron death (reviewed in ref. Citation26). Consistent with a role for inflammation in RGC degeneration after injury, administering IL-1ra was neuroprotective following retinal ischemia or optic nerve injury.Citation23,Citation24 However, cytokine functions are often pleiotropic, and there is evidence that TNFα and IL-1β can improve RGC survival after axotomy. In RGCs, TNFα downregulated a K+ current,Citation4 and IL-1β altered Na+ and K+ currents and activated the PI3 kinase pathway.Citation22 Both treatments reduced RGC death, but this rescue was not abolished by IL-1raCitation22 and it was concluded that IL-1β exerted direct neuroprotective effects, independent of effects on microglia.Citation27 We monitored change in mRNA levels, and while we do not know to what degree the protein levels changes, our observation that IL-1ra expression was increased by AgTx-2 treatment (but not KV1.1 or KV1.3 depletion from RGCs) is consistent with IL-1ra counteracting the pro-inflammatory effects of IL-1β, while permitting the direct anti-apoptotic effects of IL-1β in injured RGCs. TGFβ regulates RGC apoptosis during development,Citation28 and we found that AgTx-2 injection increased TGFβ expression; however, adenoviral-mediated expression of TGFβ did not improve RGC survival in an earlier study.Citation29 Elevated nitric oxide can contribute to RGC degeneration in this model.Citation30 We did not observe changes in iNOS expression but it is likely that localized or transient changes were missed when we sampled the entire damaged retina. That is, Mueller glia constitutively express iNOS, which is regulated during phases of light and dark adaptation, and because these cells are large and distributed throughout the inner and outer retina, localized changes in iNOS in the damaged ganglion cell layer might not be detected. In addition, it is possible that elevated expression of IL-1ra prevented the upregulation of iNOS that follows increased IL-1β expression.
Our finding of additive or supra-additive rescue of RGCs by injecting a KV blocker along with depleting either KV1.1 or KV1.3 in RGCs points to additional indirect mechanisms of neuroprotection. Microglia can produce neurotrophic factors and microglial activation depends on functional KV1.3 channels.Citation10 MgTx injection, but not RGC-specific KV1.1 or KV1.3 depletion, increased bFGF, a growth factor previously found to rescue RGCs.Citation31 Interestingly, AgTx-2, which also blocks KV1.1 and KV1.3, did not affect bFGF expression, suggesting that increased bFGF levels might result from the additional block of KV1.2 by MgTx, independent of KV1.1 or KV1.3 channels.
Our observation that KV1.3 channel blockers reduce RGC degeneration in vivo has broader implications. Not only do KV1.3 channels contribute to activation of microglia,Citation10 which are often the first cells to respond to CNS injury or disease, but KV1.3 is also expressed in some neurons. Thus, KV1.3 inhibition might be a useful approach for controlling inflammation in other retinal and CNS disorders, and might also be directly neuroprotective. Some potent peptidyl inhibitors of KV1.3 have been tested in animal models, without evidence of toxicity (reviewed in ref. Citation14), and even poorly selective KV1-family blockers (e.g., 4-aminopyridine) are being tested in human clinical trials for multiple sclerosis and spinal cord injury, apparently without intolerable side effects.
Materials and Methods
Optic nerve transection, siRNA-mediated channel knockdown and KV blocker injection.
These procedures were carried out in accordance with guidelines from the Canadian Council on Animal Care. For detailed methods and references please see our recent publication.Citation5 In brief, to observe retinal ganglion cells (RGCs) in the normal retina, Fluorogold was injected into the superior colliculus, allowed to undergo retrograde transport for 24 h, and then the retina was fixed. For optic nerve transection, a 1 mm section of the nerve was removed from within 1.5 mm of the back of the eye; RGCs were labeled by applying Fluorogold to the freshly cut optic nerve stump, which was retrogradely transported to their somata. KV1.1 or KV1.3 channels were selectively depleted from RGCs by injecting 10 ng of a channel-specific siRNA into the nerve stump. The siRNA sequences and pseudonyms were: 5′-CCU UGU GUA UCA UCU GGU U-3′ (KV1.1–922); and 5′-CCA TGA CAA CTG TCG GTT A-3′ (KV1.3–1169). Control injections consisted of an irrelevant Cy3-labeled siRNA (Upstate, Charlottesville, VA) directed against a non-mammalian protein, firefly luciferase: 5′-CGU ACG CGG AAU ACU UCG A-3′. We previously showedCitation5 that these siRNAs were not toxic, and their effectiveness was demonstrated using immunohistochemistry in transverse retinal sections; i.e., there was specific knockdown of the salient KV1 channel from RGCs without effects on channels in other retinal cells.
KV channel blockers were administered by intraocular injection at the time of axotomy and again at four days, the time at which RGC apoptosis begins in this model (reviewed in refs. Citation1–Citation3 and Citation19). Three microliters of saline were injected, containing 50 µM margatoxin (MgTx) or agitoxin-2 (AgTx-2) or α-dendrotoxin (α-DTx). For details about these blockers, see ref. Citation14. We previously showed that these blocker concentrations had no adverse effects on the rats following intraocular injection.Citation5 Control injections consisted of saline alone; i.e., 100 mM NaCl, 10 mM TRIS, 1 mM EDTA, 0.1% BSA, pH 7.5. Rats were briefly anaesthetized with isofluorane, and the vitreous chamber was injected at the time of optic nerve transection and again four days later. Injections were made with a glass micropipette posterior to the limbus, being careful to avoid damaging anterior ocular structures or puncturing the lens, which evokes release of growth factors. To assess combined responses to a channel blocker plus an siRNA, comparisons were made between control siRNA and a channel-specific siRNA delivered to the optic nerve stump.
Immunohistochemistry.
Locations and morphologies of microglia were monitored in normal retinas and five days after optic nerve transection. Whole mounts were prepared from fixed retinas and labeled overnight at 4°C with a mouse monoclonal antibody against complement receptor 3 (OX-42; Serotec, Oxford, UK) at a 1:250 dilution in phosphate-buffered saline (PBS) containing 3% normal goat serum and 0.3% Triton X-100. Retinas were then washed with PBS (3×, 15 min each) and incubated for 4 h at room temperature with an affinity-purified Alexa 488-conjugated goat-anti-mouse secondary antibody (Jackson ImmunoResearch, West Grove, PA), diluted in PBS. After washing with PBS (3×, 15 min each), retinas were flat-mounted with 1:1 glycerol:PBS and stored at 4°C. For each assay, the concentration of antibodies, fluorescence intensity gain, and pinhole and neutral density filters were kept constant. There was no staining when the primary antibody was omitted (not shown), and the black regions within each image in show the lack of non-specific OX-42 staining.
Real-time quantitative RT-PCR.
Changes in gene transcript expression were monitored by quantitative RT-PCR (qRT-PCR) in whole retinas harvested seven days after axotomy, as before.Citation5 Gene-specific primers () were designed using the ‘Primer3Output’ program (HUhttp://frodo.wi.mit.edu/cgi-bin/primer3/primer3_www.cgiUH). RNA was isolated (RNeasy; Qiagen, Inc., Mississauga, Ontario) after degrading any contaminating DNA with DNaseI (0.1 U/mL, 15 min, 37°C; Amersham Biosciences, Baie d'Urfe, Quebec). Total RNA (2 µg) was reverse transcribed in 20 µL volume using 200 U of SuperScriptII RNase H-reverse transcriptase, with 0.5 mM dNTPs (Invitrogen, Carlsbad, CA) and 0.5 µM oligo dT (Sigma-Aldrich, St. Louis, MO). Amplification was performed on an ABI PRISM 7900 Sequence Detection System (PE Biosystems, Foster City, CA) at 95°C for 10 min, followed by 40 cycles at 95°C for 15 s, 55°C for 15 s and 72°C for 30 s. For each gene, ‘no-template’ and ‘no-amplification’ controls were negative, and melt curves showed a single peak, confirming specific amplification. The threshold cycle (CT) for each gene was determined and normalized against the housekeeping gene, hypoxanthine guanine phosphoribosyl transferase (HPRT-1). Significant differences between experimental treatments were identified by ANOVA, followed by Fisher's Mean Interval Comparisons.
Quantifying RGC survival after axotomy.
As before,Citation5 we quantified RGC survival at 14 days post-axotomy. Rats were sacrificed by an overdose of anaesthetic (7% chloral hydrate), their eyes were enucleated, the cornea and lens removed, and the remaining eye cups fixed (1.5 h, room temperature) in 4% paraformaldehyde in PBS containing 2% sucrose. The eye cup was washed with PBS for 15 min, and the neural retina was dissected away from the sclera and flat-mounted in 1:1 glycerol:PBS. The density of surviving Fluorogold-labeled RGCs was counted from confocal micrographs (Zeiss LSM 510). Because RGC density varies somewhat across the retina, we used a standard procedure, wherein cells in flat-mounted retinas were counted in a 70,000 µm2 area at three retinal eccentricities (inner, middle, outer; see cartoon in ) from each quadrant of the retina, for a total of 12 fields of cells per retina.
Abbreviations
RGC | = | retinal ganglion cell |
GCL | = | ganglion cell layer |
NFL | = | nerve fiber layer |
IPL | = | inner plexiform layer |
INL | = | inner nuclear layer |
ONL | = | outer nuclear layer |
CNS | = | central nervous system |
BDNF | = | brain derived neurotrophic factor |
CNTF | = | ciliary neurotrophic factor |
GDNF | = | glial-cell line derived neurotrophic factor |
NGF | = | nerve growth factor |
bFGF | = | basic fibroblast growth factor |
IGF | = | insulin-like growth factor |
EGF | = | epidermal growth factor |
NRTN | = | neurturin |
iNOS | = | inducible nitric oxide synthase |
IL-1β | = | interleukin-1-beta |
IL-1ra | = | interleukin-1 receptor antagonist |
TNFα | = | tumor necrosis factor-alpha |
TGFβ | = | transforming growth factor-beta |
CR3 | = | complement receptor 3 |
MHC Class II | = | major histocompatibility complex class 2 |
GFAP | = | glial fibrillary acidic protein |
HPRT-1 | = | hypoxanthine phosphoribosyltransferase 1 |
AgTx-2 | = | agitoxin-2 |
MgTx | = | margatoxin |
α-DTX | = | alpha-dendrotoxin |
KV | = | voltage gated potassium channel subunit |
KV1.1 | = | voltage gated potassium channel family 1 member 1 |
KV1.2 | = | voltage gated potassium channel family 1 member 2 |
KV1.3 | = | voltage gated potassium channel family 1 member 3 |
Caspase-3 | = | cysteine-aspartic protease-3 |
Caspase-9 | = | cysteine-aspartic protease-9 |
Bad | = | Bcl-2-associated death promoter |
BclxL | = | B-cell lymphoma-extra large |
Figures and Tables
Figure 1 Inflammation and the microglial response after optic nerve transection. (A) Schematic showing the layers and neural types in the normal adult retina. The nerve fiber layer (NFL) is comprised of axons of retinal ganglion cells (RGCs); the ganglion cell layer (GCL) contains RGC somata; the inner plexiform layer (IPL) includes amacrine cells and processes of RGCs; the inner nuclear layer (IN L) contains bipolar cells; the outer plexiform layer (OPL) contains horizontal cells; and the outer nuclear layer (ONL) contains photoreceptors. (B–E) Representative confocal images from flat mounts of healthy retinas (n = 4 for each treatment), taken from the mid-periphery (see inset in ). RGCs and their axons were retrogradelabeled with Fluorogold (false-colored green); microglia were labeled with OX-42 antibody (red). The level at which each image was taken is indicated by corresponding red letter in (A). Microglia were very sparse in the outer plexiform layer (B). Highly ramified microglia were densely distributed in the inner plexiform layer (C), and adjacent to the GCL (D), where numerous RGC cell bodies can be seen. In the nerve fiber layer (E), only a few amoeboid microglia are seen among the RGC axon fascicles. Scale bar, 50 µm; applies to all parts. (F) Microglia in the NFL at 14 days after axotomy in a saline-injected retina. Some microglia (yellow in the overlay) are labeled with Fluorogold, as a result of phagocytosing apoptotic RGCs (green); the higher-magnification inset shows phagocytosed RGCs inside two microglia. Very few RGC cell bodies remain and their axons are no longer visible. Instead, there are large numbers of microglia, which are much less ramified than in the healthy retina and mainly organized in rows, similar to the RGC axon fascicles in the healthy retina (E). (G–I) Microglia in the NFL (14 days after axotomy), following intraocular KV blocker injections (50 µM) at the time of axotomy and 4 days later. The blockers were: agitoxin-2 (G), margatoxin (H) and α-dendrotoxin (I). (J) Changes in gene expression at 7 days after optic nerve transection, measured by quantitative real-time RT-PCR (for primer sequences, see ). One group of genes represents responses of microglia, complement receptor-3 (CR3) and MHC class II; astrocytes, glial fibrillary acidic protein (GFAP); and neurons, synaptophysin (SYP). The second includes cFos and the inflammation-related genes, TNFα, IL-1β, iNOS, IL-1 receptor antagonist (IL-1ra). For each gene, the relative expression was normalized to the housekeeping gene, HPRT-1, and values are shown as mean ± SEM (n = 6 retinas each), with significant differences (*p < 0.05; **p < 0.01) determined by ANOVA, followed by Fisher's test.
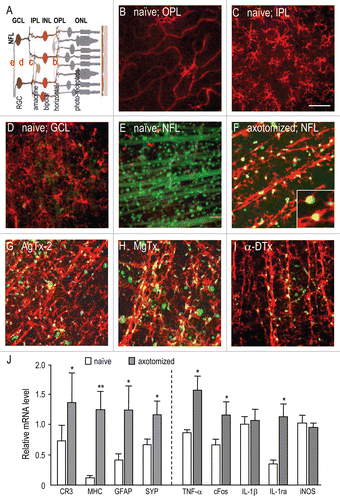
Figure 2 KV block and RGC-specific knockdown differentially affect growth factor expression. Retinal expression of gene transcripts was compared by quantitative real-time RT-PCR at seven days after optic nerve transection. Expression of each gene was normalized to the value in untreated retinas after optic nerve transection (axotomized). Data are expressed as mean ± SEM (6 retinas each); the statistical significance was determined by ANOVA, followed by Fisher's test. (A) Effects of siRNA-mediated depletion of either KV1.1 or KV1.3 from RGCs only. Statistical comparisons between healthy retinas (naïve) and axotomized retinas are indicated (*p < 0.05), as are differences from retinas treated with control siRNA directed against firefly luciferase (#p < 0.05). (B) Two intraocular injections of 50 µM AgTx-2 or MgTx were given: one at the time of axotomy and another four days later. Comparisons are between the KV channel blockers and control (saline-injected) retinas (#p < 0.05, ##p < 0.01).
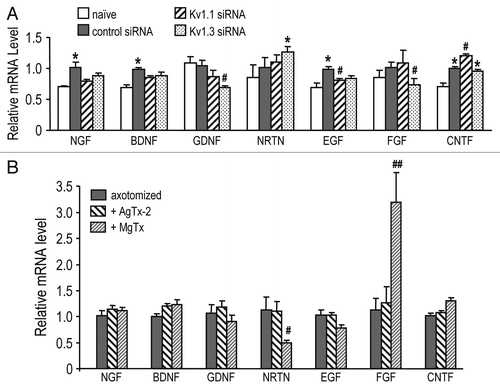
Figure 3 Combining channel knockdown with KV1.3 blockade differentially affects inflammatory gene expression. Retinal expression of gene transcripts was assessed by quantitative real-time RT-PCR at seven days after optic nerve transection. shows the primer sequences used to detect these inflammation-related genes. Freshly cut nerve stumps were immediately injected with siRNA against KV1.1 or KV1.3, and compared with a control siRNA against firefly luciferase (as for ). When used, two intraocular injections of 50 µM AgTx-2 were given: one at the time of axotomy and another four days later (as for ). Expression of each gene was normalized to the value in axotomized retinas injected with the control siRNA. Data are expressed as mean ± SEM (six retinas each). The statistical significance was determined by AN OVA, followed by Fisher's test: *p < 0.05 indicates values that are significantly different from controls; #p < 0.05 indicates that the combination of the siRNA and AgTx-2 was significantly more effective than the siRNA alone.
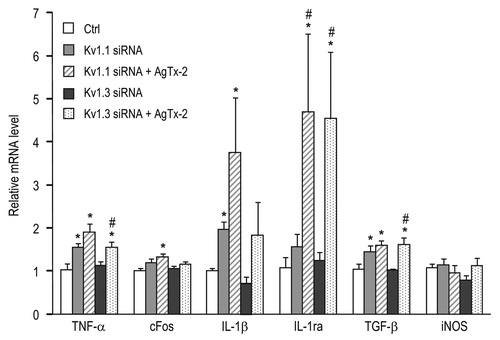
Figure 4 Supra-additive RGC rescue by combined KV block and RGC-specific KV knockdown. (A–D) Representative confocal micrographs from flat-mounted retinas of adult rats at 14 days after axotomy. RGCs were retrograde labeled with Fluorogold applied to the freshly cut optic nerve stump (see Methods). In all examples, siRNA s were injected into the cut nerve stump immediately after optic nerve transection; scale bar, 50 µm in all panels. Few RGCs survived at this time following injection of control siRNA directed against firefly luciferase (A). (B) shows increased RGC survival after injecting combined siRNA s directed against KV1.1 (KV1.1–922) and KV1.3 (KV1.3–1169). Many more RGCs were seen after intraocular injection (on Day 4) of 50 µM agitoxin-2 (AgTx-2) was combined with injection of an siRNA directed against either KV1.1 (KV1.1–922; (C) or KV1.3 (D); KV1.3–1169). (E) Summary of RGC densities 14 days after axotomy. The inset cartoon shows the approximate locations of the 12 fields that were counted from the inner, mid-periphery and outer retinal eccentricities (four fields per site). The values for RGC density are mean ± SEM of four retinas in each experimental group. From left to right, the bars show the treatments: control siRNA against firefly luciferase (as above); an siRNA directed against KV1.1, combined with two different siRNA s directed against KV1.3; the KV1.1 siRNA combined with intraocular injection of 50 µM margatoxin (MgTx) or AgTx-2; one of the KV1.3 siRNA s combined with intraocular injection of 50 µM margatoxin (MgTx) or AgTx-2. The letters above each set of bars represent different statistical comparisons (ANOVA, followed by Fisher's test): (A) p < 0.001 with respect to the control siRNA; (B) p < 0.01 with respect to the combined siRNAs; (C) p < 0.001 with respect to KV1.1–922 or MgTx or AgTx-2 alone; (D) p < 0.001 with respect to KV1.3–1169 or MgTx or AgTx-2 alone.
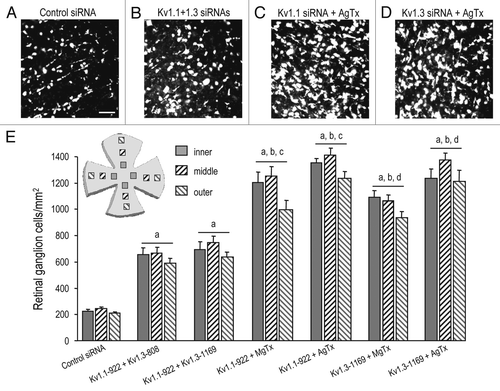
Table 1 Primers used for real-time quantitative real-time RT-PCR
Acknowledgements
We are grateful to Drs. Yimin Wang and Xiaoping Zhu for performing real-time RT-PCR analysis. This work was supported by operating grants to Lyanne C. Schlichter from the Canadian Institutes for Health Research (MT-13657), the Heart and Stroke Foundation, Ontario chapter (HSFO T5546) and the Krembil Scientific Development Seed Fund. Paulo D. Koeberle was supported by a postdoctoral fellowship from HSF Canada.
Addendum to:
References
- Bahr M. Live or let die—retinal ganglion cell death and survival during development and in the lesioned adult CNS. Trends Neurosci 2000; 23:483 - 490
- Koeberle PD, Bahr M. Growth and guidance cues for regenerating axons: where have they gone?. J Neurobiol 2004; 59:162 - 180
- Watanabe M, Sawai H, Fukuda Y. Survival of axotomized retinal ganglion cells in adult mammals. Clin Neurosci 1997; 4:233 - 239
- Diem R, Meyer R, Weishaupt JH, Bahr M. Reduction of potassium currents and phosphatidylinositol 3-kinase-dependent AKT phosphorylation by tumor necrosis factor-α rescues axotomized retinal ganglion cells from retrograde cell death in vivo. J Neurosci 2001; 21:2058 - 2066
- Koeberle PD, Wang Y, Schlichter LC. KV1.1 and KV1.3 channels contribute to the degeneration of retinal ganglion cells after optic nerve transection in vivo. Cell Death Differ 2010; 17:134 - 144
- Missel PJ. Finite and infinitesimal representations of the vasculature: ocular drug clearance by vascular and hydraulic effects. Ann Biomed Eng 2002; 30:1128 - 1139
- Chen L, Yang P, Kijlstra A. Distribution, markers and functions of retinal microglia. Ocul Immunol Inflamm 2002; 10:27 - 39
- Langmann T. Microglia activation in retinal degeneration. J Leukoc Biol 2007; 81:1345 - 1351
- Schuetz E, Thanos S. Microglia-targeted pharmacotherapy in retinal neurodegenerative diseases. Curr Drug Targets 2004; 5:619 - 627
- Fordyce CB, Jagasia R, Zhu X, Schlichter LC. Microglia KV1.3 channels contribute to their ability to kill neurons. J Neurosci 2005; 25:7139 - 7149
- Berkelaar M, Clarke DB, Wang YC, Bray GM, Aguayo AJ. Axotomy results in delayed death and apoptosis of retinal ganglion cells in adult rats. J Neurosci 1994; 14:4368 - 4374
- Koeberle PD, Ball AK. Effects of GDNF on retinal ganglion cell survival following axotomy. Vision Res 1998; 38:1505 - 1515
- Villegas-Perez MP, Vidal-Sanz M, Rasminsky M, Bray GM, Aguayo AJ. Rapid and protracted phases of retinal ganglion cell loss follow axotomy in the optic nerve of adult rats. J Neurobiol 1993; 24:23 - 36
- Wulff H, Zhorov BS. K+ channel modulators for the treatment of neurological disorders and autoimmune diseases. Chem Rev 2008; 108:1744 - 1773
- Germain F, Fernandez E, de la Villa P. Morphometrical analysis of dendritic arborization in axotomized retinal ganglion cells. Eur J Neurosci 2003; 18:1103 - 1109
- Emch GS, Hermann GE, Rogers RC. TNFα-induced c-Fos generation in the nucleus of the solitary tract is blocked by NBQX and MK-801. Am J Physiol Regul Integr Comp Physiol 2001; 281:1394 - 1400
- Isenmann S, Kretz A, Cellerino A. Molecular determinants of retinal ganglion cell development, survival and regeneration. Prog Retinal Eye Res 2003; 22:483 - 543
- Levin LA. Intrinsic survival mechanisms for retinal ganglion cells. Eur J Ophthalmol 1999; 9:12 - 16
- Weishaupt JH, Bahr M. Degeneration of axotomized retinal ganglion cells as a model for neuronal apoptosis in the central nervous system—molecular death and survival pathways. Restor Neurol Neurosci 2001; 19:19 - 27
- Garcia-Valenzuela E, Sharma SC, Pina AL. Multilayered retinal microglial response to optic nerve transection in rats. Mol Vis 2005; 11:225 - 231
- Sobrado-Calvo P, Vidal-Sanz M, Villegas-Perez MP. Rat retinal microglial cells under normal conditions, after optic nerve section, and after optic nerve section and intravitreal injection of trophic factors or macrophage inhibitory factor. J Comp Neurol 2007; 501:866 - 878
- Diem R, Hobom M, Grotsch P, Kramer B, Bahr M. Interleukin-1beta protects neurons via the interleukin-1 (IL-1) receptor-mediated Akt pathway and by IL-1 receptor-independent decrease of transmembrane currents in vivo. Mol Cell Neurosci 2003; 22:487 - 500
- Yoneda S, Tanihara H, Kido N, Honda Y, Goto W, Hara H, Miyawaki N. Interleukin-1beta mediates ischemic injury in the rat retina. Exp Eye Res 2001; 73:661 - 667
- Zhang X, Chintala SK. Influence of interleukin-1 beta induction and mitogen-activated protein kinase phosphorylation on optic nerve ligation-induced matrix metalloproteinase-9 activation in the retina. Exp Eye Res 2004; 78:849 - 860
- Yang J, Tezel G, Patil RV, Wax MB. Flow cytometry for quantification of retrogradely labeled retinal ganglion cells by Fluoro-Gold. Curr Eye Res 2000; 21:981 - 985
- Frank-Cannon TC, Alto LT, McAlpine FE, Tansey MG. Does neuroinflammation fan the flame in neurodegenerative diseases?. Mol Neurodegen 2009; 4:47
- Simi A, Tsakiri N, Wang P, Rothwell NJ. Interleukin-1 and inflammatory neurodegeneration. Biochem Soc Trans 2007; 35:1122 - 1126
- Beier M, Franke A, Paunel-Gorgulu AN, Scheerer N, Dunker N. Transforming growth factor beta mediates apoptosis in the ganglion cell layer during all programmed cell death periods of the developing murine retina. Neurosci Res 2006; 56:193 - 203
- Koeberle PD, Gauldie J, Ball AK. Effects of adenoviral-mediated gene transfer of interleukin-10, interleukin-4 and transforming growth factor-β on the survival of axotomized retinal ganglion cells. Neuroscience 2004; 125:903 - 920
- Koeberle PD, Ball AK. Nitric oxide synthase inhibition delays axonal degeneration and promotes the survival of axotomized retinal ganglion cells. Exp Neurol 1999; 158:366 - 381
- Sievers J, Hausmann B, Unsicker K, Berry M. Fibroblast growth factors promote the survival of adult rat retinal ganglion cells after transection of the optic nerve. Neurosci Lett 1987; 76:157 - 162