Abstract
Recent findings demonstrate that synaptic channels are directly involved in the formation and maintenance of synapses by interacting with synapse organizers. The synaptic channels on the pre- and postsynaptic membranes possess non-conducting roles in addition to their functional roles as ion-conducting channels required for synaptic transmission. For example, presynaptic voltage-dependent calcium channels link the target-derived synapse organizer laminin β2 to cytomatrix of the active zone and function as scaffolding proteins to organize the presynaptic active zones. Furthermore, postsynaptic δ2-type glutamate receptors organize the synapses by forming transsynaptic protein complexes with presynaptic neurexins through synapse organizer cerebellin 1 precursor proteins. Interestingly, the synaptic clustering of AMPA receptors is regulated by neuronal activity-regulated pentraxins, while postsynaptic differentiation is induced by the interaction of postsynaptic calcium channels and thrombospondins. This review will focus on the non-conducting functions of ion-channels that contribute to the synapse formation in concert with synapse organizers and active-zone-specific proteins.
Introduction
A functional nervous system requires the formation and maintenance of synapses. Chemical neurotransmission requires a sensing of action potentials by presynaptic voltage-dependent calcium channels (VDCCs) and the resultant Ca2+ influx into the presynaptic terminal that induces the fusion of synaptic vesicles to presynaptic membranes.Citation1,Citation2 Chemical neurotransmitters released via exocytosis will then bind and open ligand-gated channels in the postsynaptic membrane to modify the excitability of the postsynaptic cell.Citation3–Citation5 Thus, synaptic ion channels must be concentrated in the pre- and postsynaptic membranes for effective synaptic transmission.
The formation and maintenance of synapses require both transynaptic organizing signals and the assembly of intracellular scaffolds. The list of molecules capable of inducing synapses (termed synapse organizers) continues to grow and has been reviewed in detail elsewhere.Citation6–Citation15 Interestingly, recent findings demonstrate that synaptic channels are also directly involved in the formation and maintenance of synapses, in addition to their functional role as ion-conducting channels required for synaptic transmission. The goal of this review is to explore how the non-conducting functions of ion-channel subunits contribute to the formation and maintenance of synapses in concert with extra-cellular synapse organizers and presynaptic active-zone-specific proteins ().
Presynaptic VDCCs and Cytosolic Proteins at the Synapse
Presynaptic localization of VDCCs requires cytosolic domains on the VDCCs (Synprint region, C-terminus domain).Citation16–Citation19 During the early stage of synapse formation, VDCCs and presynaptic active zone proteins are transported to the axon terminals by preassembled protein/vesicle packets.Citation20,Citation21 A unitary assembly of a nascent synapse has been modeled based on these preassembled transport vesicles.Citation20–Citation22 At the presynaptic terminal, VDCCs are strategically located in close proximity to the neurotransmitter release sites, which reduces the delay between Ca2+ entry and synaptic vesicle fusion.Citation23–Citation29 The ultrastructural location of presynaptic VDCCs has been suggested as the paired double rows of 100-Å membrane macromolecules that are identified on the protoplasmic fracture face of freeze-fracture electron micrographs.Citation30–Citation32 Electron tomography analysis revealed large protein complexes at the presynaptic terminal, including the structure called “pegs” that aligned well with the paired double rows of membrane macromolecules.Citation33–Citation35 Thus, it is likely that presynaptic VDCCs form a complex with proteins required for active zone organization and synaptic vesicle fusion.
Few groups have taken a proteomics approach to identify the proteins associated with VDCCs.Citation36–Citation39 The extensive lists of proteins in these VDCC complexes coincide with the macromolecules composing active zone material visualized by electron tomography.Citation33,Citation34,Citation40 VDCC subunits interact with some of these proteins directly, and with other proteins in these lists indirectly. Cytosolic interactions of VDCCs and synaptic proteins (SNAP-25, syntaxin, synaptotagmin) that modulate channel functions are reviewed in Turner et al. in this issue of Channels and elsewhere.Citation41 Furthermore, the organization of the presynaptic protein complex has been reviewed in detail elsewhere.Citation42,Citation43 Thus, the first section of this review will focus on the recently identified direct interactions of VDCC subunits and presynaptic active-zone-specific proteins. Active zones are areas of the presynaptic membrane with electron-dense material where synaptic vesicles fuse.Citation44–Citation46 The so-called cytomatrix of the active zone accumulates specifically at presynaptic active zones and includes Bassoon,Citation47 Bruchpilot,Citation48 CAST/Erc2,Citation49 Munc13,Citation50 Piccolo,Citation51 Rim1,Citation52 and SYD-2.Citation53–Citation56
VDCCα subunits and active zone proteins.
Structural interactions of VDCCα subunits during presynaptic differentiation have been demonstrated in central and peripheral synapses. The Cav2.2 (N-type, α1B,) and Cav1.2 (L-type, α1C) VDCCs, but not Cav1.3 (L-type, α1D), bind directly with the active zone protein Rim through interactions between the cytoplasmic loop connecting domain II–III of VDCC and the C2A or C2B domains of Rim.Citation57 Rim also interacts directly with the VDCCβ subunit, which will be discussed below.Citation58 Furthermore, the Cav2.2 and Cav2.1 (P/Q-type, α1A) VDCCα subunits and Rim1/2 bind directly through interactions between the C-terminus domain of the VDCCα subunit and the central PDZ domain of Rim.Citation59 The Rim PDZ domain is required for maintaining presynaptic Ca2+ channel localization, and the Rim N-terminus primes synaptic vesicles.Citation59
Similarly, Cav1.2 (L-type, α1C) VDCCs, but not Cav1.3 (L-type, α1D), bind directly with the active zone protein Piccolo through interactions between the cytoplasmic loop connecting domain II–III of VDCC and the C2A or C2B domains of Piccolo.Citation60 These interactions were suggested to play roles in insulin granule exocytosis, which seems to serve a similar function as the Rim interaction mentioned above.
The interactions of the VDCC and active zone proteins are conserved in invertebrate synapses as well. At the Drosophila neuromuscular junction, Cacophony (the P/Q-type VDCCα subunit homologue) interacts directly with the active zone-specific protein Bruchpilot (Brp: a CAST/ERC family member).Citation61 In Brp mutants, reduced levels of VDCCs (Cacophony) accumulated at active-zone-like structures at neuromuscular junctions, and specialized active zone structures called T-bars were missing. Thus, some VDCCs can initially accumulate at active zones without Brp, but the VDCC-Brp interaction is responsible for effectively clustering more VDCCs beneath the active zone density.Citation61
VDCCβ subunits and active zone proteins.
Like the α subunits, VDCCβ subunits interact directly with active zone proteins. VDCCβ subunits bind directly with Rim1 through interactions between the center domain of the VDCCβ subunit (containing the Src homology 3 domain, α1-interacting domain and guanylate kinase domain) and the C-terminus domain of Rim1 (including the C2B domain).Citation58,Citation62 Protein complexes consisting of the P/Q-type VDCCα subunit, VDCCβ subunits and Rim1 can be co-sedimented from mouse brain homogenates, demonstrating the formation of triad in vivo. This interaction suppresses the voltage-dependent inactivation of neuronal VDCCs. Together with the enhanced synaptic vesicle docking in the vicinity of VDCCs that is caused by Rim1, this interaction potentiates neurotransmitter release at the presynaptic active zone.
VDCCβ subunits also interact with the active zone proteins Bassoon and CAST/Erc2.Citation63 These active zone proteins are coimmunoprecipitated as triad of the P/Q-type VDCCα subunit, β1b or β4 subunits, and Bassoon or CAST/Erc2.Citation63 This interaction is essential to structurally organize the presynaptic active zones at neuromuscular junctions.Citation63,Citation64 In the absence of functional Bassoon, whole-cell Ca2+ current and Ca2+ influx at the presynaptic microdomain of inner hair cells (primarily reflecting Ca2+ influx at active zones) are reduced.Citation65 These findings suggest that the role of the VDCCβ subunit-Bassoon interaction may be similar to the role of the interaction between the VDCCβ subunit and Rim1.
These interactions between VDCC subunits and active-zone-specific proteins allow for anchoring of the active zone cytomatrix to the presynaptic membrane. Active zone proteins are also known to interact between themselves.Citation66–Citation68 Consistently, Bassoon, Piccolo and Rim are found in protein complexes containing VDCCs in vivo.Citation36,Citation37 Double-knockout mice for P/Q VDCCs and N-type VDCCs have synapses specifically lacking active zones.Citation63 Taken together, these interactions suggest a formation of a macromolecular protein complex on the cytosolic side of the presynaptic VDCC, which is likely to be the active zones visualized as an electron dense projection in the ultrastructural analysis by electron tomography.Citation27,Citation28
Presynaptic VDCCs and Extracellular Synapse Organizers
Two types of VDCCs, the P/Q- and N-types, are concentrated at many presynaptic terminals in the central and peripheral nervous system and play essential roles for synaptic transmission.Citation69–Citation78 A growing number of reports demonstrate that VDCC subunits are involved in organizing presynaptic differentiation jointly with synapse organizers. The second section of this review will focus on the extracellular interaction between presynaptic VDCC subunits and synaptic molecules that is necessary for synapse formation.
P/Q-type VDCCs and laminin β2.
VDCC α subunits are known to have non-conducting functions for presynaptic differentiation at the neuromuscular junction. Presynaptic P/Q- and N-type VDCCs bind directly to laminin β2,Citation64 which is a synapse organizer secreted by postsynaptic muscle cells.Citation79,Citation80 The P/Q-type VDCC utilizes its 11th extracellular loop domain (46 amino acids, excluding the lip domain) to interact with laminin β2 at its C-terminal 20 kDa domain, which includes a leucinearginine-glutamine sequence. This interaction organizes the synaptic vesicle release sites or active zones, at motor nerve terminals.Citation63,Citation64 Furthermore, this interaction is linked to active-zone-specific proteins by VDCCβ subunits, suggesting a mechanism to link synapse organizers to the cytosolic presynaptic proteins (described in section I).Citation63 Single-knockout mice for P/Q-type VDCCs, N-type VDCCs or laminin β2, or double-knockout mice for P/Q-type VDCCs and N-type VDCCs show reduced numbers of active zones.Citation63,Citation64,Citation79 Laminin β2 induces presynaptic differentiation in cultured motor neurons even in the presence of P/Q- and N-type VDCC blockade by agatoxin-IVA and conotoxin-GIVA, providing evidence for the dispensability of the Ca2+ influx into nerve terminals for active zone formation.Citation64 This extracellular interaction of presynaptic VDCCs and synaptogenic molecules organizes the presynaptic differentiation of neuromuscular junctions.
Similar to the neuromuscular junction, laminin β2 is concentrated in the synaptic cleft at the photoreceptor synapses and aid the differentiation of retinal neurons.Citation81,Citation82 Instead of P/Q-type VDCCs, the Cav1.4 (L-type, α1F) VDCC is preferentially expressed in the retina and is concentrated at photoreceptor synapses.Citation83–Citation85 Genetic deletions of these genes in mice causes a dissociation of the ribbons, an active zone structure, from presynaptic membranes at the photoreceptor synapses.Citation83,Citation86 This active zone phenotype suggests that the interaction of presynaptic VDCCs and laminin β2 plays an essential role in organizing active zones at the photoreceptor synapse, similar to the neuromuscular junction.Citation87,Citation88
N-type VDCCs and α-neurexin.
A functional link between N-type VDCCs and presynaptic neurexin has been suggested.Citation89 Accumulation of VDCCs at brainstem synapses was impaired in the triple-knockout mice for synapse organizer α-neurexins.Citation89 Interestingly, this phenotype is specific to N-type VDCCs and P/Q-type VDCCs are not affected. However, a direct physical interaction between N-type VDCCs and neurexins awaits confirmation. The presynaptic proteins CASK and Mint/X11 can link between VDCCs and α-neurexins.Citation90,Citation91 However, the possibility of this interaction is decreased by the fact that knockout mice for CASK demonstrated normal active zones and functional presynaptic VDCCs. Similarly, cultured triple-knockout neurons for Mint1/2/3 showed normal synaptic ultrastructure and a defect of presynaptic function that seems to be attributable to the upregulated Munc18-1.Citation92
In a similar manner, a functional link between postsynaptic L-type VDCCs and the extracellular matrix glycoprotein tenascin-C has been suggested for hippocampal long-term potentiation,Citation88 but the evidence for a direct physical interaction between VDCCs and tenascin-C awaits confirmation.
VDCC α2δ subunit.
The VDCC α2δ subunits play roles in presynaptic differentiation at the Drosophila neuromuscular junction.Citation93,Citation94 Mutant embryos lacking the α2δ-3 subunit have malformed synaptic boutons. This role of the α2δ-3 subunit is independent of the ion-conducting function of the calcium channel complex, and is separate from its role of properly localizing VDCCα subunits at the neuromuscular junctions.Citation93 Any role of synapse organizer for these phenotypes is currently unknown.
Postsynaptic Channels and Extracellular Synapse Organizers
A primary function of postsynaptic ligand-gated ion channels at chemical synapses is to bind neurotransmitters and then open to modify the excitability of the postsynaptic cells. The cytosolic domains of these ligand-gated channels interact with postsynaptic scaffolding proteins to organize the synapse and modify the channel functions, which is detailed in other reviews.Citation95,Citation96 In addition to these interactions, the subunits of postsynaptic channels also show synaptogenic activities or interact with synapse organizers. The third section of this review will focus on extracellular interactions of postsynaptic channel subunits and synapse organizers.
Glutamate receptor δ2 and cerebellin1.
Postsynaptic δ-type glutamate receptors (GluRδ2, GluD2) form transsynaptic protein complexes with presynaptic neurexins through synapse organizer cerebellin 1 precursor proteins (Cbln1).Citation97 GluRδ2 is selectively expressed in Purkinje cells of the cerebellumCitation98,Citation99 and is exclusively localized at parallel fiber-Purkinje cell synapses.Citation100,Citation101 GluRδ2 forms heteromeric channels with AMPA or kainate receptors in vitro, but can exist as a homomeric receptor in vivo.Citation102,Citation103 Importantly, GluRδ2 demonstrates synaptogenic activity in vivo, as demonstrated by knockout mouse studies.Citation104–Citation106 The N-terminal domain of GluRδ2 induces presynaptic differentiation in vitro and in vivo.Citation107,Citation108 Finally, a GluRδ2-null cerebellum shows impaired long-term depression (LTD) of parallel fiber-Purkinje cell synaptic transmission.Citation104
The N-terminal domain of GluRδ2 binds directly to Cbln1,Citation97,Citation107 and Cbln1 also binds directly to neurexins 1β/2β/3β and 1α, containing the S4 splice site.Citation97,Citation109 The Cbln1 knockout mouse shows ataxia and a severe reduction in the number of synapses between Purkinje cells and parallel fibers,Citation97,Citation110 which closely resembles the GluRδ2-null mouse. Strikingly, the synaptic defect in the Cbln1 knockout mouse can be rescued within a day by a single injection of recombinant Cbln1.Citation111 This triad interaction of GluRδ2, Cbln1 and neurexin is essential for synapse formation between parallel fiber and Purkinje cells in cerebellum. This interaction can align postsynaptic channels to the synapse organizer located at the presynaptic terminal.Citation97,Citation109 Cbln1 belongs to the C1q family of proteins; C1q plays a role in synapse elimination,Citation112,Citation113 and its non-channel receptor has been identified recently.Citation114
Glutamate receptors and the neuronal pentraxin family, N-Cadherin, and EphB.
The synaptic clustering of AMPA receptors (GluR1-4 subunits, GluA1-4) is regulated by neuronal activity-regulated pentraxin (Narp).Citation115–Citation118 Narp is a member of the neuronal pentraxin family of calcium-dependent lectins, which includes neuronal pentraxin1 (NP1) and neuronal pentraxin receptor (NPR).Citation118,Citation119 Narp and NP1 are secreted proteins that form heteromeric complexes on the extracellular surface,Citation117 and NPR is a transmembrane protein.Citation118 Of the neuronal pentraxin family members, Narp is the only immediately early gene regulated by synaptic activity.Citation117
The N-terminal domain of the AMPA receptor GluR4 subunit interacts with the pentraxin domain of NP1.Citation117,Citation120 Axonal NP1 and NPR in presynaptic neurons are required to recruit GluR4 to synapses.Citation120 The N-terminal domain of GluR4 is necessary and sufficient for its recruitment to the synapses.Citation120 The Narp knockout mouse shows Narp's requirement for activity-dependent changes in the strength of excitatory inputs onto parvalbumin-expressing interneurons of the hippocampus.Citation121 Triple-knockout mice for neuronal pentraxins indicate that the pentraxins are necessary for early synaptic refinements in the retina and dorsal lateral geniculate nucleus.Citation122
AMPA receptors are rapidly endocytosed in an mGluR1/5 dependent manner.Citation123–Citation125 This endocytosis of the GluR1 subunit requires NRP, which is cleaved by the MMP tumor necrosis factor-alpha converting enzyme (TACE) in an mGluR1/5-dependent fashion.Citation126 The analysis using knockout mice for NRP and TACE blockers showed that NRP and TACE-activity are required for mGluR1/5-dependent LTD in hippocampal and cerebellar synapses.Citation126
Similarly, the N-terminal domain of AMPA receptor subunit GluR2 (GluA2) interacts directly with N-Cadherin and promotes formation and growth of dendritic spines in vitroCitation127 and regulates hippocampal LTD.Citation128 The extracellular domain of the NMDA receptor subunit NR1 (GluN1) interacts directly with tyrosine kinase EphB2 and regulates synapse development.Citation129,Citation130
GABAA receptor and neurexin.
Postsynaptic GABAA receptors interact directly with presynaptic neurexins.Citation131 GABAA receptors can be purified from brain homogenates using immobilized neurexin-2β in a neuroligin-independent manner. A recombinant protein of the extracellular domain of the GABAAα1 receptor binds the extracellular domain of neurexin. Overexpression of neurexins in cultured neurons selectively suppresses GABAergic synaptic transmission without decreasing the number of GABAergic synapses, and this effect is independent of neuroligin. This extracellular interaction suggests a potential mechanism to control the inhibitory synaptic transmissions in the brain. It is interesting to note that an increasing number of synapse organizers and synaptic proteins (Cbln1, α-Dystroglycan, GABAA receptor, LRRTMs, neuroligins) are found to interact directly with neurexins and contribute to the organization of synapses.Citation97,Citation131–Citation137
VDCC α2δ subunit and thrombospondin.
The auxiliary subunit of the VDCC, α2δ subunit, is a receptor for the glial-derived synaptogenic molecule thrombospondin.Citation138 These two proteins bind directly through the interaction between the von Willebrand factor type A domain of the α2δ-1 subunit and the type 2 EGF-like repeats of thrombospondin1-5.Citation138 This interaction promotes the formation of excitatory synapses with postsynaptically silent synapses lacking AMPA receptors in the mammalian central nervous system.Citation138,Citation139 This α2δ-thrombospondin-mediated synapse formation does not require the ion-conducting function of L-, N- or P/Q-type VDCCs.Citation138 Eroglu and colleagues concluded that α2δ is necessary and sufficient postsynaptically for this synaptogenic activity based on their analysis of α2δ-1 overexpression and knockdown in the postsynaptic cells. Thrombospondin induces ultrastructurally normal synapses that are presynaptically active,Citation139 but the involvement of presynaptic α2δ subunits or the identity of the presynaptic receptor for the thrombospondin-induced presynaptic differentiation awaits further study. Interestingly, thrombospondin has been shown to also interact in vitro with the synapse organizer neuroligin, which localizes at the postsynaptic membrane.Citation140 These interactions may cooperate for postsynaptic differentiation.
Knockout mice for the α2δ-2 subunit exhibit morphological abnormalities of Purkinje cell dendrites in the cerebellum.Citation141 Mice lacking the α2δ-4 subunit exhibit a significantly reduced outer plexiform layer, and their intraretinal circuitry and functions are perturbed.Citation142 The phenotypes in these mutant mice suggest roles of the VDCC α2δ subunit in synapse formation, but ultrastructural or immunohistochemical analyses of the synapse in these mutants mice awaits further study. Whether thrombospondin (or other synapse organizers) contributes to the phenotypes in these α2δ mutants remains unclear.
Are there More Extracellular Interactions of Ion Channels at Synapses?
Potentially, additional interactions of synaptic ion channel-synapse organizer remain to be identified. This possibility is supported by several examples of extracellular interactions of ion channel subunits identified outside the synapse. For instance, G-protein-activated inward rectifier K (GIRK) channels interact directly with integrins.Citation143 The arginine-glycine-asparatate (RGD) sequence located in the extracellular domain of GIRK channel binds to integrins. This interaction increases the plasma membrane localization of GIRK channels. Also, the voltage-dependent sodium channel α subunit interacts with the extracellular carbonic anhydrase domain of receptor protein tyrosine phosphatase β (RPTPβ).Citation144 RPTPβ also interacts with the cytosolic domains of the voltage-dependent sodium channel α subunit and β1 subunit. These interactions modulate channel function by the phosphatase activity of RPTPβ. The β1/2 subunits of voltage-dependent sodium channels also interact directly with cell adhesion molecules (connexin-43, contactin, N-cadherin, NrCAM, neurofascin-155, -186, tenascin-C/R) on axons and glia cells.Citation145–Citation150 The diverse functional roles of these homophilic and heterophilic cell adhesions of voltage-dependent sodium channels are reviewed in detail elsewhere.Citation151–Citation153 Genetic interactions of the mechanotransduction channel MEC-4/10 and the extracellular anchor protein MEC-5 through the extracellular link protein MEC-9, or with collagen unc-105 have been suggested, but these await confirmation of direct physical interactions.Citation154–Citation157 These examples show that extracellular interactions of ion channel subunits can be quite diverse and extensive.
Summary and Perspectives
The studies summarized in this review clearly establish non-conducting roles for ion-channel subunits in the formation and maintenance of synapses, in addition to their important role as ion-permeable channels for chemical neurotransmission. Both presynaptic and postsynaptic channel subunits interact with extracellular synapse organizers. Such structural interactions are extended into the cytosolic region by using the ion-channel subunits as scaffolding proteins. These trans-synaptic protein interactions allow alignment of pre- and postsynaptic specialization to achieve effective neurotransmission.
Important questions still remain to elucidate the role of trans-synaptic molecular mechanisms involving ion channel subunits for organizing synapses. First, the initial interaction or the essential interaction to precisely position these synaptic channels at the pre- and postsynaptic sites remains unknown. Recent findings described in this review suggest that the location of these synaptic channels can be modified either from cytosolic side or extracellular side. Second, when or how much is ion-conducting function of channels required for the formation/maturation/maintenance of synapses? The molecular mechanism responsible for the initial phase of synapse formation does not seem to require synaptic activity. Even in the absence of synaptic transmission, morphologically normal synapses can be formed with active zones in knockout mice for choline acetyltransferase or munc18-1, or double-knockout mice for munc13-1/2 or P/Q-, N-type VDCCs.Citation63,Citation158–Citation160 However, activity becomes important later for synaptic elimination and P/Q-type VDCCs plays an essential role at the climbing fiber-Purkinje cell synapse.Citation161 Third, the timing of the synapse transmission control/modification by these transsynaptic interactions is unknown. The extracellular interactions reviewed here can modify both excitatory and inhibitory synapses, but the control of these actions remains unknown. These are just few examples of questions that await further investigation to elucidate synapse formation and maintenance.
Figures and Tables
Figure 1 A schematic diagram of a subset of synaptic channels and interacting proteins. Solid arrows represent interactions and the dotted arrows indicate a functional link. Horizontal double lines show pre- and post-synaptic membranes. The space between these double lines represents the synaptic cleft. The gray triangle depicts the electron dense material of presynaptic active zones detected by electron microscopy. Some protein domains involved in protein-protein interactions are color-coded and listed in the top right box. The size of proteins and the synaptic cleft are not in scale. Abbreviations: cerebellin 1 precursor proteins (Cbln1), GABAA receptor (GABAA), Glutamate receptor (GluR), leucine-rich repeat transmembrane neuronal proteins (LRRTMs), neuronal activity-regulated pentraxin (Narp), neuronal pentraxin1 (NP1), neuronal pentraxin receptor (NPR), NMDA receptor subunit GluN1 (NR1), voltage-dependent calcium channels (VDCC).
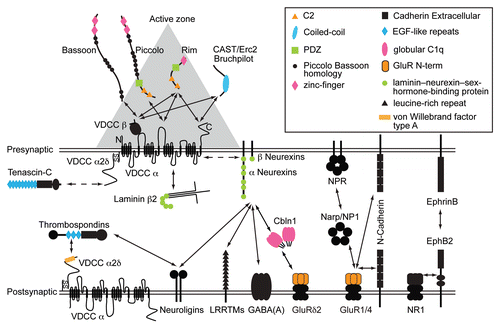
Acknowledgments
I thank Yasuo Mori and Yoshikatsu Kanai for the invitation for this review and Michael A. Fox and Shigeki Kiyonaka for comments on the manuscript. The work in my laboratory is supported by grants from the NIH-NCRR (P20RR024214), Whitehall Foundation, and by the Kansas IDDRC (P30 NICHD HD 002528).
References
- Katz B, Miledi R. A study of synaptic transmission in the absence of nerve impulses. J Physiol 1967; 192:407 - 436
- Katz B, Miledi R. The timing of calcium action during neuromuscular transmission. J Physiol 1967; 189:535 - 544
- Sakmann B, Patlak J, Neher E. Single acetylcholine-activated channels show burst-kinetics in presence of desensitizing concentrations of agonist. Nature 1980; 286:71 - 73
- Anderson CR, Cull-Candy SG, Miledi R. Glutamate and quisqualate noise in voltage-clamped locust muscle fibres. Nature 1976; 261:151 - 153
- Diamond J, Huxley AF. The activation and distribution of GABA and L-glutamate receptors on goldfish Mauthner neurones: an analysis of dendritic remote inhibition. J Physiol 1968; 194:669 - 723
- Siddiqui TJ, Craig AM. Synaptic organizing complexes. Curr Op Neurobiol 2010; 21:1 - 12
- Yamagata M, Sanes JR, Weiner JA. Synaptic adhesion molecules. Curr Opin Cell Biol 2003; 15:621 - 632
- Fox MA, Umemori H. Seeking long-term relationship: axon and target communicate to organize synaptic differentiation. J Neurochem 2006; 97:1215 - 1231
- Sudhof TC. Neuroligins and neurexins link synaptic function to cognitive disease. Nature 2008; 455:903 - 911
- Jin Y. Synaptogenesis. WormBook 2005; 1 - 11
- Sanes JR, Lichtman JW. Development of the vertebrate neuromuscular junction. Ann Rev Neurosci 1999; 22:389 - 442
- Scheiffele P. Cell-cell signaling during synapse formation in the CNS. Ann Rev Neurosci 2003; 26:485 - 508
- Lai KO, Ip NY. Central synapse and neuromuscular junction: same players, different roles. Trends Gene 2003; 19:395 - 402
- Broadie KS, Richmond JE. Establishing and sculpting the synapse in Drosophila and C. elegans. Curr Op Neurobiol 2002; 12:491 - 498
- Collins CA, DiAntonio A. Synaptic development: insights from Drosophila. Curr Op Neurobiol 2007; 17:35 - 42
- Mochida S, Westenbroek RE, Yokoyama CT, Zhong H, Myers SJ, Scheuer T, et al. Requirement for the synaptic protein interaction site for reconstitution of synaptic transmission by P/Q-type calcium channels. Proc Natl Acad Sci USA 2003; 100:2819 - 2824
- Maximov A, Bezprozvanny I. Synaptic targeting of N-type calcium channels in hippocampal neurons. J Neurosci 2002; 22:6939 - 6952
- Bezprozvanny I, Scheller RH, Tsien RW. Functional impact of syntaxin on gating of N-type and Q-type calcium channels. Nature 1995; 378:623 - 626
- Sheng ZH, Rettig J, Takahashi M, Catterall WA. Identification of a syntaxin-binding site on N-type calcium channels. Neuron 1994; 13:1303 - 1313
- Ahmari SE, Buchanan J, Smith SJ. Assembly of presynaptic active zones from cytoplasmic transport packets. Nat Neurosci 2000; 3:445 - 451
- Shapira M, Zhai RG, Dresbach T, Bresler T, Torres VI, Gundelfinger ED, et al. Unitary assembly of presynaptic active zones from Piccolo-Bassoon transport vesicles. Neuron 2003; 38:237 - 252
- Zhai RG, Vardinon-Friedman H, Cases-Langhoff C, Becker B, Gundelfinger ED, Ziv NE, et al. Assembling the presynaptic active zone: a characterization of an active one precursor vesicle. Neuron 2001; 29:131 - 143
- Wachman ES, Poage RE, Stiles JR, Farkas DL, Meriney SD. Spatial distribution of calcium entry evoked by single action potentials within the presynaptic active zone. J Neurosci 2004; 24:2877 - 2885
- Neher E. Vesicle pools and Ca2+ microdomains: new tools for understanding their roles in neurotransmitter release. Neuron 1998; 20:389 - 399
- Stanley EF. Single calcium channels on a cholinergic presynaptic nerve terminal. Neuron 1991; 7:585 - 591
- Stanley EF. The calcium channel and the organization of the presynaptic transmitter release face. Trends Neurosci 1997; 20:404 - 409
- Urbano FJ, Piedras-Renteria ES, Jun K, Shin HS, Uchitel OD, Tsien RW. Altered properties of quantal neurotransmitter release at endplates of mice lacking P/Q-type Ca2+ channels. Proc Natl Acad Sci USA 2003; 100:3491 - 3496
- Cao YQ, Piedras-RenterIa ES, Smith GB, Chen G, Harata NC, Tsien RW. Presynaptic Ca2+ channels compete for channel type-preferring sots in altered neurotransmission arising from Ca2+ channelopathy. Neuron 2004; 43:387 - 400
- Robitaille R, Adler EM, Charlton MP. Strategic location of calcium channels at transmitter release sites of frog neuromuscular synapses. Neuron 1990; 5:773 - 779
- Fukunaga H, Engel AG, Osame M, Lambert EH. Paucity and disorganization of presynaptic membrane active zones in the Lambert-Eaton myasthenic syndrome. Muscle Nerve 1982; 5:686 - 697
- Haydon PG, Henderson E, Stanley EF. Localization of individual calcium channels at the release face of a presynaptic nerve terminal. Neuron 1994; 13:1275 - 1280
- Ellisman MH, Rash JE, Staehelin LA, Porter KR. Studies of excitable membranes. II. A comparison of specializations at neuromuscular junctions and non-junctional sarcolemmas of mammalian fast and slow twitch muscle fibers. J Cell Biol 1976; 68:752 - 774
- Harlow ML, Ress D, Stoschek A, Marshall RM, McMahan UJ. The architecture of active zone material at the frog's neuromuscular junction. Nature 2001; 409:479 - 484
- Nagwaney S, Harlow ML, Jung JH, Szule JA, Ress D, Xu J, et al. Macromolecular connections of active zone material to docked synaptic vesicles and presynaptic membrane at neuromuscular junctions of mouse. J Comp Neurol 2009; 513:457 - 468
- Fukuoka T, Engel AG, Lang B, Newsom-Davis J, Prior C, Wray DW. Lambert-Eaton myasthenic syndrome: I. Early morphological effects of IgG on the presynaptic membrane active zones. Ann Neurol 1987; 22:193 - 199
- Muller C, Haupt A, Bildl W, Schindler J, Knaus H, Meissner M, et al. Quantitative proteomics of the CaV2 channel nano-environments in the mammalian brain. Proc Natl Acad Sci USA 2010; 107:14950 - 14957
- Carlson SS, Valdez G, Sanes JR. Presynaptic calcium channels and α3-Integrins are complexed with synaptic cleft laminins, cytoskeletal elements and active zone components. J Neurochem 2010; 115:654 - 666
- Khanna R, Zougman A, Stanley EF. A proteomic screen for presynaptic terminal N-type calcium channel (Cav2.2) binding partners. J Biochem Mol Biol 2007; 40:302 - 314
- Klemmer P, Smit AB, Li KW. Proteomics analysis of immuno-precipitated synaptic protein complexes. J Proteomics 2009; 72:82 - 90
- Fernandez-Busnadiego R, Zuber B, Maurer UE, Cyrklaff M, Baumeister W, Lucic V. Quantitative analysis of the native presynaptic cytomatrix by cryoelectron tomography. J Cell Biol 2010; 188:145 - 156
- Catterall WA, Few AP. Calcium channel regulation and presynaptic plasticity. Neuron 2008; 59:882 - 901
- Ziv NE, Garner CC. Cellular and molecular mechanisms of presynaptic assembly. Nat Rev Neurosci 2004; 5:385 - 399
- Jin Y, Garner CC. Molecular mechanisms of presynaptic differentiation. Annu Rev Cell Dev Biol 2008; 24:237 - 262
- Couteaux R, Pecot-Dechavassine M. Synaptic vesicles and pouches at the level of “active zones” of the neuromuscular junction. C R Acad Sci Hebd Seances Acad Sci D 1970; 271:2346 - 2349
- Zhai RG, Bellen HJ. The architecture of the active zone in the presynaptic nerve terminal. Physiology 2004; 19:262 - 270
- Rosenmund C, Rettig J, Brose N. Molecular mechanisms of active zone function. Curr Op Neurobiol 2003; 13:509 - 519
- tom Dieck S, Sanmarti-Vila L, Langnaese K, Richter K, Kindler S, Soyke A, et al. Bassoon, a novel zinc-finger CAG/glutamine-repeat protein selectively localized at the active zone of presynaptic nerve terminals. J Cell Biol 1998; 142:499 - 509
- Kittel RJ, Wichmann C, Rasse TM, Fouquet W, Schmidt M, Schmid A, et al. Bruchpilot promotes active zone assembly, Ca2+ channel clustering and vesicle release. Science 2006; 312:1051 - 1054
- Ohtsuka T, Takao-Rikitsu E, Inoue E, Inoue M, Takeuchi M, Matsubara K, et al. Cast: a novel protein of the cytomatrix at the active zone of synapses that forms a ternary complex with RIM1 and munc13-1. J Cell Biol 2002; 158:577 - 590
- Betz A, Ashery U, Rickmann M, Augustin I, Neher E, Sudhof TC, et al. Munc13-1 is a presynaptic phorbol ester receptor that enhances neurotransmitter release. Neuron 1998; 21:123 - 136
- Cases-Langhoff C, Voss B, Garner AM, Appeltauer U, Takei K, Kindler S, et al. Piccolo, a novel 420 kDa protein associated with the presynaptic cytomatrix. Eur J Cell Biol 1996; 69:214 - 223
- Wang Y, Okamoto M, Schmitz F, Hofmann K, Sudhof TC. Rim is a putative Rab3 effector in regulating synaptic-vesicle fusion. Nature 1997; 388:593 - 598
- Yeh E, Kawano T, Weimer RM, Bessereau JL, Zhen M. Identification of genes involved in synaptogenesis using a fluorescent active zone marker in Caenorhabditis elegans. J Neurosci 2005; 25:3833 - 3841
- Sigrist SJ, Schmitz D. Structural and functional plasticity of the cytoplasmic active zone. Curr Op Neurobiol 2010; 21:1 - 7
- Schoch S, Gundelfinger ED. Molecular organization of the presynaptic active zone. Cell Tissue Res 2006; 326:379 - 391
- tom Dieck S, Altrock WD, Kessels MM, Qualmann B, Regus H, Brauner D, et al. Molecular dissection of the photoreceptor ribbon synapse: physical interaction of Bassoon and RIBEYE is essential for the assembly of the ribbon complex. J Cell Biol 2005; 168:825 - 836
- Coppola T, Magnin-Luthi S, Perret-Menoud V, Gattesco S, Schiavo G, Regazzi R. Direct interaction of the Rab3 effector RIM with Ca2+ channels, SNAP-25 and synaptotagmin. J Biol Chem 2001; 276:32756 - 32762
- Kiyonaka S, Wakamori M, Miki T, Uriu Y, Nonaka M, Bito H, et al. RIM1 confers sustained activity and neurotransmitter vesicle anchoring to presynaptic Ca2+ channels. Nat Neurosci 2007; 10:691 - 701
- Kaeser PS, Deng L, Wang Y, Dulubova I, Liu X, Rizo J, et al. RIM proteins tether Ca2+ channels to presynaptic active zones via a direct PDZ-domain interaction. Cell 2011; 144:282 - 295
- Shibasaki T, Sunaga Y, Fujimoto K, Kashima Y, Seino S. Interaction of ATP sensor, cAMP sensor, Ca2+ sensor and voltage-dependent Ca2+ channel in insulin granule exocytosis. J Biol Chem 2004; 279:7956 - 7961
- Fouquet W, Owald D, Wichmann C, Mertel S, Depner H, Dyba M, et al. Maturation of active zone assembly by Drosophila Bruchpilot. J Cell Biol 2009; 186:129 - 145
- Uriu Y, Kiyonaka S, Miki T, Yagi M, Akiyama S, Mori E, et al. Rab3-interacting Molecule g isoforms lacking the Rab3-binding domain induce long-lasting currents but block neurotransmitter vesicle-anchoring in voltage-dependent P/Q-type Ca2+ channels. J Biol Chem 2010; 285:21750 - 21767
- Chen J, Billings SE, Nishimune H. Calcium channels link the muscle-eerived synapse organizer laminin beta2 to Bassoon and CAST/Erc2 to organize presynaptic active zones. J Neurosci 2011; 31:512 - 525
- Nishimune H, Sanes JR, Carlson SS. A synaptic laminin-calcium channel interaction organizes active zones in motor nerve terminals. Nature 2004; 432:580 - 587
- Frank T, Rutherford MA, Strenzke N, Neef A, Pangrsic T, Khimich D, et al. Bassoon and the synaptic ribbon organize Ca2+ channels and vesicles to add release sites and promote refilling. Neuron 2010; 68:724 - 738
- Wang X, Hu B, Zieba A, Neumann NG, Kasper-Sonnenberg M, Honsbein A, et al. A protein interaction node at the neurotransmitter release site: domains of Aczonin/Piccolo, Bassoon, CAST and rim converge on the N-terminal domain of Munc13-1. J Neurosci 2009; 29:12584 - 12596
- Takao-Rikitsu E, Mochida S, Inoue E, Deguchi-Tawarada M, Inoue M, Ohtsuka T, et al. Physical and functional interaction of the active zone proteins, CAST, RIM1 and Bassoon, in neurotransmitter release. J Cell Biol 2004; 164:301 - 311
- Betz A, Thakur P, Junge HJ, Ashery U, Rhee JS, Scheuss V, et al. Functional interaction of the active zone proteins Munc13-1 and RIM1 in synaptic vesicle priming. Neuron 2001; 30:183 - 196
- Mori Y, Friedrich T, Kim MS, Mikami A, Nakai J, Ruth P, et al. Primary structure and functional expression from complementary DNA of a brain calcium channel. Nature 1991; 350:398 - 402
- Starr TV, Prystay W, Snutch TP. Primary structure of a calcium channel that is highly expressed in the rat cerebellum. Proc Natl Acad Sci USA 1991; 88:5621 - 5625
- Catterall WA. Structure and function of neuronal Ca2+ channels and their role in neurotransmitter release. Cell Calcium 1998; 24:307 - 323
- Reid CA, Bekkers JM, Clements JD. Presynaptic Ca2+ channels: a functional patchwork. Trends Neurosci 2003; 26:683 - 687
- Waterman SA. Multiple subtypes of voltage-gated calcium channel mediate transmitter release from parasympathetic neurons in the mouse bladder. J Neurosci 1996; 16:4155 - 4161
- Urbano FJ, Rosato-Siri MD, Uchitel OD. Calcium channels involved in neurotransmitter release at adult, neonatal and P/Q-type deficient neuromuscular junctions (Review). Mol Membr Biol 2002; 19:293 - 300
- Waterman SA. Role of N-, P- and Q-type voltage-gated calcium channels in transmitter release from sympathetic neurones in the mouse isolated vas deferens. Br J Pharmacol 1997; 120:393 - 398
- Williams ME, Brust PF, Feldman DH, Patthi S, Simerson S, Maroufi A, et al. Structure and functional expression of an omega-conotoxin-sensitive human N-type calcium channel. Science 1992; 257:389 - 395
- Fujita Y, Mynlieff M, Dirksen RT, Kim MS, Niidome T, Nakai J, et al. Primary structure and functional expression of the omega-conotoxin-sensitive N-type calcium channel from rabbit brain. Neuron 1993; 10:585 - 598
- Dubel SJ, Starr TV, Hell J, Ahlijanian MK, Enyeart JJ, Catterall WA, et al. Molecular cloning of the alpha-1 subunit of an omega-conotoxin-sensitive calcium channel. Proc Natl Acad Sci USA 1992; 89:5058 - 5062
- Noakes PG, Gautam M, Mudd J, Sanes JR, Merlie JP. Aberrant differentiation of neuromuscular junctions in mice lacking s-laminin/laminin beta2. Nature 1995; 374:258 - 262
- Hunter DD, Shah V, Merlie JP, Sanes JR. A laminin-like adhesive protein concentrated in the synaptic cleft of the neuromuscular junction. Nature 1989; 338:229 - 234
- Libby RT, Champliaud MF, Claudepierre T, Xu Y, Gibbons EP, Koch M, et al. Laminin expression in adult and developing retinae: evidence of two novel CNS laminins. J Neurosci 2000; 20:6517 - 6528
- Hunter DD, Murphy MD, Olsson CV, Brunken WJ. S-laminin expression in adult and developing retinae: a potential cue for photoreceptor morphogenesis. Neuron 1992; 8:399 - 413
- Mansergh F, Orton NC, Vessey JP, Lalonde MR, Stell WK, Tremblay F, et al. Mutation of the calcium channel gene Cacna1f disrupts calcium signaling, synaptic transmission and cellular organization in mouse retina. Hum Mol Genet 2005; 14:3035 - 3046
- Bech-Hansen NT, Naylor MJ, Maybaum TA, Pearce WG, Koop B, Fishman GA, et al. Loss-of-function mutations in a calcium-channel alpha1-subunit gene in Xp11.23 cause incomplete X-linked congenital stationary night blindness. Nature genetics 1998; 19:264 - 267
- Strom TM, Nyakatura G, Apfelstedt-Sylla E, Hellebrand H, Lorenz B, Weber BH, et al. An L-type calcium-channel gene mutated in incomplete X-linked congenital stationary night blindness. Nature genetics 1998; 19:260 - 263
- Libby RT, Lavallee CR, Balkema GW, Brunken WJ, Hunter DD. Disruption of laminin beta2 chain production causes alterations in morphology and function in the CNS. J Neurosci 1999; 19:9399 - 9411
- Dityatev A, Schachner M, Sonderegger P. The dual role of the extracellular matrix in synaptic plasticity and homeostasis. Nat Rev Neurosci 2010; 11:735 - 746
- Evers MR, Salmen B, Bukalo O, Rollenhagen A, Bosl MR, Morellini F, et al. Impairment of L-type Ca2+ channel-dependent forms of hippocampal synaptic plasticity in mice deficient in the extracellular matrix glycoprotein tenascin-C. J Neurosci 2002; 22:7177 - 7194
- Missler M, Zhang W, Rohlmann A, Kattenstroth G, Hammer RE, Gottmann K, et al. Alpha-neurexins couple Ca2+ channels to synaptic vesicle exocytosis. Nature 2003; 423:939 - 948
- Hata Y, Butz S, Sudhof T. CASK: a novel dlg/PSD95 homolog with an N-terminal calmodulin-dependent protein kinase domain identified by interaction with neurexins. J Neurosci 1996; 16:2488 - 2494
- Maximov A, Sudhof TC, Bezprozvanny I. Association of neuronal calcium channels with modular adaptor proteins. J Biol Chem 1999; 274:24453 - 24456
- Atasoy D, Schoch S, Ho A, Nadasy KA, Liu X, Zhang W, et al. Deletion of CASK in mice is lethal and impairs synaptic function. Proc Natl Acad Sci USA 2007; 104:2525 - 2530
- Kurshan PT, Oztan A, Schwarz TL. Presynaptic α2δ-3 is required for synaptic morphogenesis independent of its Ca2+-channel functions. Nat Neurosci 2009; 12:1415 - 1423
- Ly CV, Yao CK, Verstreken P, Ohyama T, Bellen HJ. straightjacket is required for the synaptic stabilization of cacophony, a voltage-gated calcium channel [alpha]1 subunit. J Cell Biol 2008; 181:157 - 170
- Sheng M, Hoogenraad CC. The postsynaptic architecture of excitatory synapses: A more quantitative view. Annu Rev Biochem 2007; 76:823 - 847
- Feng W, Zhang M. Organization and dynamics of PDZ-domain-related supramodules in the postsynaptic density. Nat Rev Neurosci 2009; 10:87 - 99
- Uemura T, Lee SJ, Yasumura M, Takeuchi T, Yoshida T, Ra M, et al. Trans-synaptic interaction of GluRdelta2 and neurexin through Cbln1 mediates synapse formation in the cerebellum. Cell 2010; 141:1068 - 1079
- Araki K, Meguro H, Kushiya E, Takayama C, Inoue Y, Mishina M. Selective expression of the glutamate receptor channel delta 2 subunit in cerebellar Purkinje cells. Biochem Biophys Res Commun 1993; 197:1267 - 1276
- Lomeli H, Sprengel R, Laurie DJ, Kohr G, Herb A, Seeburg PH, et al. The rat delta-1 and delta-2 subunits extend the excitatory amino acid receptor family. FEBS Lett 1993; 315:318 - 322
- Takayama C, Nakagawa S, Watanabe M, Mishina M, Inoue Y. Developmental changes in expression and distribution of the glutamate receptor channel delta2 subunit according to the Purkinje cell maturation. Brain Res Dev Brain Res 1996; 92:147 - 155
- Landsend AS, Amiry-Moghaddam M, Matsubara A, Bergersen L, Usami S, Wenthold RJ, et al. Differential localization of delta glutamate receptors in the rat cerebellum: coexpression with AMPA receptors in parallel fiber-spine synapses and absence from climbing fiber-spine synapses. J Neurosci 1997; 17:834 - 842
- Kohda K, Kamiya Y, Matsuda S, Kato K, Umemori H, Yuzaki M. Heteromer formation of delta2 glutamate receptors with AMPA or kainate receptors. Brain Res Mol Brain Res 2003; 110:27 - 37
- Mayat E, Petralia RS, Wang YX, Wenthold RJ. Immunoprecipitation, immunoblotting and immunocytochemistry studies suggest that glutamate receptor delta subunits form novel postsynaptic receptor complexes. J Neurosci 1995; 15:2533 - 2546
- Kashiwabuchi N, Ikeda K, Araki K, Hirano T, Shibuki K, Takayama C, et al. Impairment of motor coordination, Purkinje cell synapse formation and cerebellar long-term depression in GluR delta2 mutant mice. Cell 1995; 81:245 - 252
- Kurihara H, Hashimoto K, Kano M, Takayama C, Sakimura K, Mishina M, et al. Impaired parallel fiber→Purkinje cell synapse stabilization during cerebellar development of mutant mice lacking the glutamate receptor delta2 subunit. J Neurosci 1997; 17:9613 - 9623
- Takeuchi T, Miyazaki T, Watanabe M, Mori H, Sakimura K, Mishina M. Control of synaptic connection by glutamate receptor [delta]2 in the adult cerebellum. J Neurosci 2005; 25:2146 - 2156
- Matsuda K, Miura E, Miyazaki T, Kakegawa W, Emi K, Narumi S, et al. Cbln1 is a ligand for an orphan glutamate receptor delta2, a bidirectional synapse organizer. Science 2010; 328:363 - 368
- Uemura T, Mishina M. The amino-terminal domain of glutamate receptor delta2 triggers presynaptic differentiation. Biochem Biophy Res Comm 2008; 377:1315 - 1319
- Yuzaki M. Cbln1 and its family proteins in synapse formation and maintenance. Curr Op Neurobiol 2011; 21:1 - 6
- Hirai H, Pang Z, Bao D, Miyazaki T, Li L, Miura E, et al. Cbln1 is essential for synaptic integrity and plasticity in the cerebellum. Nat Neurosci 2005; 8:1534 - 1541
- Ito-Ishida A, Miura E, Emi K, Matsuda K, Iijima T, Kondo T, et al. Cbln1 regulates rapid formation and maintenance of excitatory synapses in mature cerebellar Purkinje cells in vitro and in vivo. J Neurosci 2008; 28:5920 - 5930
- Stevens B, Allen NJ, Vazquez LE, Howell GR, Christopherson KS, Nouri N, et al. The classical complement cascade mediates CNS synapse elimination. Cell 2007; 131:1164 - 1178
- Chu Y, Jin X, Parada I, Pesic A, Stevens B, Barres B, et al. Enhanced synaptic connectivity and epilepsy in C1q knockout mice. Proc Natl Acad Sci USA 2010; 107:7975 - 7980
- Bolliger MF, Martinelli DC, Sudhof TC. The cell-adhesion G protein-coupled receptor BAI3 is a high-affinity receptor for C1q-like proteins. Proc Natl Acad Sci USA 2011; 108:2534 - 2539
- O'Brien RJ, Xu D, Petralia RS, Steward O, Huganir RL, Worley P. Synaptic clustering of AMPA receptors by the extracellular immediate-early gene product Narp. Neuron 1999; 23:309 - 323
- Mi R, Tang X, Sutter R, Xu D, Worley P, O'Brien RJ. Differing mechanisms for glutamate receptor aggregation on dendritic spines and shafts in cultured hippocampal neurons. J Neurosci 2002; 22:7606 - 7616
- Xu D, Hopf C, Reddy R, Cho RW, Guo L, Lanahan A, et al. Narp and NP1 form heterocomplexes that function in developmental and activity-dependent synaptic plasticity. Neuron 2003; 39:513 - 528
- Dodds DC, Omeis IA, Cushman SJ, Helms JA, Perin MS. Neuronal pentraxin receptor, a novel putative integral membrane pentraxin that interacts with neuronal pentraxin 1 and 2 and taipoxin-associated calcium-binding protein 49. J Biol Chem 1997; 272:21488 - 21494
- Tsui CC, Copeland NG, Gilbert DJ, Jenkins NA, Barnes C, Worley PF. Narp, a novel member of the pentraxin family, promotes neurite outgrowth and is dynamically regulated by neuronal activity. J Neurosci 1996; 16:2463 - 2478
- Sia GM, BÈÔque JC, Rumbaugh G, Cho R, Worley PF, Huganir RL. Interaction of the N-terminal domain of the AMPA receptor GluR4 subunit with the neuronal pentraxin NP1 mediates GluR4 synaptic recruitment. Neuron 2007; 55:87 - 102
- Chang MC, Park JM, Pelkey KA, Grabenstatter HL, Xu D, Linden DJ, et al. Narp regulates homeostatic scaling of excitatory synapses on parvalbumin-expressing interneurons. Nat Neurosci 2010; 13:1090 - 1097
- Bjartmar L, Huberman AD, Ullian EM, Renteria RC, Liu X, Xu W, et al. Neuronal pentraxins mediate synaptic refinement in the developing visual system. J Neurosci 2006; 26:6269 - 6281
- Xiao MY, Zhou Q, Nicoll RA. Metabotropic glutamate receptor activation causes a rapid redistribution of AMPA receptors. Neuropharmacol 2001; 41:664 - 671
- Huber KM, Roder JC, Bear MF. Chemical induction of mGluR5- and protein synthesis-dependent long-term depression in hippocampal area CA1. J Neurophysiol 2001; 86:321 - 325
- Snyder EM, Philpot BD, Huber KM, Dong X, Fallon JR, Bear MF. Internalization of ionotropic glutamate receptors in response to mGluR activation. Nature Neurosci 2001; 4:1079 - 1085
- Cho RW, Park JM, Wolff SB, Xu D, Hopf C, Kim JA, et al. mGluR1/5-dependent long-term depression requires the regulated ectodomain cleavage of neuronal pentraxin NPR by TACE. Neuron 2008; 57:858 - 871
- Saglietti L, Dequidt C, Kamieniarz K, Rousset MC, Valnegri P, Thoumine O, et al. Extracellular interactions between GluR2 and N-cadherin in spine regulation. Neuron 2007; 54:461 - 477
- Zhou Z, Hu J, Passafaro M, Xie W, Jia Z. GluA2 (GluR2) regulates metabotropic glutamate receptor-dependent long-term depression through N-cadherindependent and cofilin-mediated actin reorganization. J Neurosci 2011; 31:819 - 833
- Dalva MB, Takasu MA, Lin MZ, Shamah SM, Hu L, Gale NW, et al. EphB receptors interact with NMDA receptors and regulate excitatory synapse formation. Cell 2000; 103:945 - 956
- Calo L, Cinque C, Patane M, Schillaci D, Battaglia G, Melchiorri D, et al. Interaction between ephrins/Eph receptors and excitatory amino acid receptors: possible relevance in the regulation of synaptic plasticity and in the pathophysiology of neuronal degeneration. J Neurochem 2006; 98:1 - 10
- Zhang C, Atasoy D, Arac D, Yang X, Fucillo MV, Robison AJ, et al. Neurexins physically and functionally interact with GABAA receptors. Neuron 2010; 66:403 - 416
- Siddiqui TJ, Pancaroglu R, Kang Y, Rooyakkers A, Craig AM. LRRTMs and neuroligins bind neurexins with a differential code to cooperate in glutamate synapse development. J Neurosci 2010; 30:7495 - 7506
- de Wit J, Sylwestrak E, O'Sullivan ML, Otto S, Tiglio K, Savas JN, et al. LRRTM2 interacts with neurexin1 and regulates excitatory synapse formation. Neuron 2009; 64:799 - 806
- Ko J, Fuccillo MV, Malenka RC, Sudhof TC. LRRTM2 Functions as a neurexin ligand in promoting excitatory synapse formation. Neuron 2009; 64:791 - 798
- Sugita S, Saito F, Tang J, Satz J, Campbell K, Sudhof TC. A stoichiometric complex of neurexins and dystroglycan in brain. J Cell Biol 2001; 154:435 - 446
- Ichtchenko K, Hata Y, Nguyen T, Ullrich B, Missler M, Moomaw C, et al. Neuroligin 1: a splice site-specific ligand for beta-neurexins. Cell 1995; 81:435 - 443
- Ichtchenko K, Nguyen T, Sudhof TC. Structures, alternative splicing and neurexin binding of multiple neuroligins. J Biol Chem 1996; 271:2676 - 2682
- Eroglu C, Allen NJ, Susman MW, O'Rourke NA, Park CY, Ozkan E, et al. Gabapentin receptor α2δ-1 is a neuronal thrombospondin receptor responsible for excitatory CNS synaptogenesis. Cell 2009; 139:380 - 392
- Christopherson KS, Ullian EM, Stokes CC, Mullowney CE, Hell JW, Agah A, et al. Thrombospondins are astrocyte-secreted proteins that promote CNS synaptogenesis. Cell 2005; 120:421 - 433
- Xu J, Xiao N, Xia J. Thrombospondin 1 accelerates synaptogenesis in hippocampal neurons through neuroligin 1. Nat Neurosci 2010; 13:22 - 24
- Brodbeck J, Davies A, Courtney JM, Meir A, Balaguero N, Canti C, et al. The ducky mutation in Cacna2d2 results in altered Purkinje cell morphology and is associated with the expression of a truncated alpha2 delta-2 protein with abnormal function. J Biol Chem 2002; 277:7684 - 7693
- Wycisk KA, Budde B, Feil S, Skosyrski S, Buzzi F, Neidhardt J, et al. Structural and functional abnormalities of retinal ribbon synapses due to Cacna2d4 mutation. Invest Ophthalmol Vis Sci 2006; 47:3523 - 3530
- McPhee JC, Dang YL, Davidson N, Lester HA. Evidence for a functional interaction between integrins and G protein-activated inward rectifier K+ channels. J Biol Chem 1998; 273:34696 - 34702
- Ratcliffe CF, Qu Y, McCormick KA, Tibbs VC, Dixon JE, Scheuer T, et al. A sodium channel signaling complex: modulation by associated receptor protein tyrosine phosphatase beta. Nature Neurosci 2000; 3:437 - 444
- McEwen DP, Isom LL. Heterophilic interactions of sodium channel beta1 subunits with axonal and glial cell adhesion molecules. J Biol Chem 2004; 279:52744 - 52752
- Malhotra JD, Kazen-Gillespie K, Hortsch M, Isom LL. Sodium channel beta subunits mediate homophilic cell adhesion and recruit ankyrin to points of cell-cell contact. J Biol Chem 2000; 275:11383 - 11388
- Ratcliffe CF, Westenbroek RE, Curtis R, Catterall WA. Sodium channel beta1 and beta3 subunits associate with neurofascin through their extracellular immunoglobulin-like domain. J Cell Biol 2001; 154:427 - 434
- Srinivasan J, Schachner M, Catterall WA. Interaction of voltage-gated sodium channels with the extracellular matrix molecules tenascin-C and tenascin-R. Proc Natl Acad Sci USA 1998; 95:15753 - 15757
- Xiao ZC, Ragsdale DS, Malhotra JD, Mattei LN, Braun PE, Schachner M, et al. Tenascin-R is a functional modulator of sodium channel beta subunits. J Biol Chem 1999; 274:26511 - 26517
- Malhotra JD, Thyagarajan V, Chen C, Isom LL. Tyrosine-phosphorylated and nonphosphorylated sodium channel beta1 subunits are differentially localized in cardiac myocytes. J Biol Chem 2004; 279:40748 - 40754
- Isom LL. The role of sodium channels in cell adhesion. Frontiers Biosci 2002; 7:12 - 23
- Catterall WA. Signaling complexes of voltage-gated sodium and calcium channels. Neurosci Lett 2010; 486:107 - 116
- Kaczmarek LK. Non-conducting functions of voltage-gated ion channels. Nat Rev Neurosci 2006; 7:761 - 771
- Gu G, Caldwell GA, Chalfie M. Genetic interactions affecting touch sensitivity in Caenorhabditis elegans. Proc Natl Acad Sci USA 1996; 93:6577 - 6582
- Du H, Gu G, William CM, Chalfie M. Extracellular proteins needed for C. elegans mechanosensation. Neuron 1996; 16:183 - 194
- Emtage L, Gu G, Hartwieg E, Chalfie M. Extracellular proteins organize the mechanosensory channel complex in C. elegans touch receptor neurons. Neuron 2004; 44:795 - 807
- Liu J, Schrank B, Waterston RH. Interaction between a putative mechanosensory membrane channel and a collagen. Science 1996; 273:361 - 364
- Misgeld T, Burgess RW, Lewis RM, Cunningham JM, Lichtman JW, Sanes JR. Roles of neurotransmitter in synapse formation: development of neuromuscular junctions lacking choline acetyltransferase. Neuron 2002; 36:635 - 648
- Verhage M, Maia AS, Plomp JJ, Brussaard AB, Heeroma JH, Vermeer H, et al. Synaptic assembly of the brain in the absence of neurotransmitter secretion. Science 2000; 287:864 - 869
- Varoqueaux F, Sigler A, Rhee JS, Brose N, Enk C, Reim K, et al. Total arrest of spontaneous and evoked synaptic transmission but normal synaptogenesis in the absence of Munc13-mediated vesicle priming. Proc Natl Acad Sci USA 2002; 99:9037 - 9042
- Miyazaki T, Hashimoto K, Shin HS, Kano M, Watanabe M. P/Q-type Ca2+ channel alpha1A regulates synaptic competition on developing cerebellar Purkinje cells. J Neurosci 2004; 24:1734 - 1743