Abstract
Viruses have developed different survival strategies in host cells by crossing cell-membrane compartments, during different steps of their viral life cycle. In fact, the non-regenerative viral membrane of enveloped viruses needs to encounter the dynamic cell-host membrane, during early steps of the infection process, in which both membranes fuse, either at cell-surface or in an endocytic compartment, to promote viral entry and infection. Once inside the cell, many viruses accomplish their replication process through exploiting or modulating membrane traffic, and generating specialized compartments to assure viral replication, viral budding and spreading, which also serve to evade the immune responses against the pathogen. In this review, we have attempted to present some data that highlight the importance of membrane dynamics during viral entry and replicative processes, in order to understand how viruses use and move through different complex and dynamic cell-membrane structures and how they use them to persist.
Viruses are small structures lacking intravital metabolic pathways and mechanisms to assure their own survival and therefore, depend a fortiori on host-cell machinery to replicate their DNA or RNA genome, and to spread their infectious progeny. Hence, many viruses have developed different survival strategies to enter and infect cells, or to accomplish their replication process through exploiting or modulating membrane traffic and generating specialized compartmentsCitation1–Citation3 (–), which also serve to evade the immune responses. In turn, increasing evidence points to that membrane dynamics, like the traffic of vesicles and their spatial reorganization, is key to cell defense against pathogen infections, as in the case of neutrophil-mediated phagocytosis, where orchestrated secretion of granules and vesicles allows cells to locate and destroy invading microorganisms in a controlled manner.Citation4–Citation7 Viruses use structural or non-structural proteins to exploit the major cellular trafficking pathways to navigate across their target cells by recruiting clathrin, coatomer protein complex (COPI) I and II or endosomal sorting complex required for transport (ESCRT) and their accessory proteins, in a non-structural or structural viral proteins-dependent manner (excellently reviewed in ref. Citation2, Citation3 and Citation8–Citation10). In addition, many of the cellular proteins recruited by the virus to accomplish its viral cycle are small GTPases that are able to generate, move and/or fuse different endosomes or vesicular compartments.Citation1–Citation3,Citation9
This work is not a comprehensive review of all membrane dynamics events reported to occur during the cycle of infection of different viruses. Instead, we have attempted to present some data that highlight the importance of the constant flux of membrane structures during early viral entry and replication processes, in order to understand how viruses move through the different complex and dynamic cell-membrane compartments to survive.
Viral Entry and Membrane Dynamics
As the initial barrier to viral entry, the plasma membrane together with membrane-trafficking machinery is also of fundamental importance in the first stages of the viral cycle.Citation2,Citation11–Citation14 Although the mechanisms of viral entry for non-enveloped virus are poorly characterized, the molecular mechanism involved in viral fusion and entry for enveloped virus begins to be more clearly understood.Citation2 It is thought that certain enveloped viruses such as human immunodeficiency virus type 1 (HIV-1), herpes simplex virus 1 (HSV-1), Sendai virus and many other retroviruses have pH-independent viral fusion proteins which allow the virus to penetrate into cells by fusing directly with the plasma membraneCitation2,Citation13,Citation15–Citation20 (; Viral fusion and entry and Viral endocytosis schemes).
Plasma membrane morphology and polarization appears to be regulated by cell cytoskeleton reorganization and direct association with the different components of cellular cortex,Citation21–Citation26 and by the insertion and uptake of membrane structures at cell-surface that regulates plasma membrane dynamics.Citation27–Citation31 Although cytoskeleton reorganization and dynamics have well-documented roles in HIV-1 fusion and entry events, the contribution of plasma membrane dynamics is less clear during these early viral infection steps. HIV-1 interacts with target cells through cell-surface CD4 and CXCR4 or CCR5 viral co-receptor, a process that is cooperative and requires cell signaling, actin polymerization and reorganizationCitation32–Citation41 and stabilization of microtubules,Citation22,Citation42,Citation43 in order to achieve pore fusion formation throughout which viruses reach the inner cell. In this concern, it has been reported that HIV-1 fusion and entry could occur in micropinosomes and endosomes,Citation44,Citation45 a process that has been proposed to be clathrindependent,Citation46 pH-independent and dynamin-dependent.Citation47,Citation48 In fact, dynamin- and endosome-dependent HIV-1 entry and infection have been recently controverted.Citation49–Citation51
In this regard, it has been reported that HIV-1 internalization and infection in polarized trophoblasts is a pH-dependent process,Citation52,Citation53 which is driven by a clathrin-, caveolae- and dynamin-independent endocytic pathway, and requires free membrane cholesterol.Citation54 A recent work from our group suggested that the fluidity of plasma membrane, regulated by the phosphatidyl-inositol-4-phosphate-5-kinase Iα (PI4P5-K Iα) and subsequent phosphatidylinositol-4,5-biphosphate (PIP2) production, is crucial for HIV-1 entry and the early steps of infection in permissive lymphocytes.Citation39 PIP2 and the PI4P5-K Iα are functionally linked to the small GTPase, ADP-ribosylation factor 6 (Arf6), which regulates membrane trafficking and regeneration of plasma membrane.Citation55–Citation58 Furthermore, efficient early HIV-1 fusion, entry and infection require both an Arf6-dependent dynamic and regenerative plasma membrane at the virus/cell-surface interacting regions,Citation51 and a correct cellsurface localization of viral receptors ( and Viral fusion and entry scheme, and associated ). Thus, movement of PIP2-associated membrane structures, driven by the Arf6-GTP/GDP cycle activity on plasma membrane, assures the regeneration of cell-surface membrane by coordinating the turnover of these PIP2-associated vesicles, which has synergy with the key first HIV-1/receptors interactions.Citation37,Citation40 Altogether, this process promotes pore fusion formation, between the non-regenerative HIV-1 viral membrane and the dynamic cell-surface, thereby favoring efficient cell-to-cell viral transmission, entry and infectionCitation51 (). The alteration of the Arf6-GTP/GDP cycle either by using GDP-bound or GTP-bound inactive mutants, which provokes the accumulation of Arf6/PIP2-associated membrane structures on cell-surface, or by specific Arf6 silencing inhibits HIV-1-envelope-induced membrane fusion, entry and infection of T lymphocytes and permissive cells, regardless of viral tropism. Conversely, Arf6 silencing or its dominant mutants did not affect fusion, entry and infection of viruses pseudotyped with the envelope-G protein of the vesicular stomatitis virus (VSV-G), or ligand-induced CXCR4 or CCR5 endocytosis, both clathrin-dependent processes. These results confirmed that early HIV-1 infection of CD4+ T lymphocytes requires Arf6-coordinated plasma membrane dynamics in order to promotes viral fusion and entry. The non-endocytic route followed by HIV-1 during early infection seems to be decisive to establish viral latent infection,Citation49 thus Arf6-regulated HIV-1 pathway of infection could be key for HIV-1 infection and pathogenesis.
In this concern, a new broad-spectrum antiviral agent, acting only against enveloped viruses (e.g., HIV-1, VSV, Ebola, Marburg, influenza, hepatitis C (HCV) and West Nile viruses), inhibited free virus-cell fusion and infection by perturbing non-regenerative viral membranes, without affecting non-enveloped viruses in vitro (e.g., adenovirus, coxsackievirus and reovirus). However, this molecule failed to block cell-to-cell fusion and infection due to its inactivity on dynamic, regenerative plasma membranes.Citation59 Considering that Arf6 is key to coordinate plasma membrane dynamics, and its functional implication on cellular invasion by HIV-1 and several microorganisms,Citation51,Citation60–Citation66 it is therefore plausible that different enveloped viruses may also benefit from Arf6-coordinated plasma membrane traffic to promote entry and infection processes. Arf6 is the only member of the Ras-related Arf-family of small GTPases that affects cell-surface dynamics, thereby regulating plasma membrane/endosome trafficking and cortical actin reorganization.Citation58,Citation67–Citation73 Remarkably, plasma membrane morphology and dynamics is also regulated by the traffic of PIP2-associated membranes from plasma membrane to a non-clathrin intracellular compartment,Citation67,Citation68,Citation74–Citation78 which in turn relies on the membrane transport activity of Arf6.Citation21,Citation56–Citation58,Citation67–Citation69,Citation79 Therefore, it is also conceivable that Arf6-coordinated membrane movements might control entry and infection processes of some non-enveloped viruses, such as coxsackievirus.Citation80
In the case of the VSV virus, which has had an important role in our increasing understanding of both innate and acquired immunity, as well as virology in general,Citation81 the viral-envelope glycoprotein G binds to phosphatidylserine, a near-universal component of cell-surface membranes, enabling VSV to infect virtually all animal cells. Although phosphatidylserine had been proposed to serve as VSV receptor,Citation82 this conviction has been recently challenged.Citation83 Following attachment to the cell surface, the virus enters by endocytosis and, after a subsequent drop in endosomal pH, the glycoprotein catalyzes the fusion of viral and cellular membranes, releasing the viral ribonucleoprotein (RNP) into the cytoplasm. In fact, VSV fusion occurs in multivesicular transport intermediates, formed between early and late endosome, and not in late endosomes,Citation84,Citation85 where finally viral nucleocapsid is delivered to the cytoplasm ( and Viral endocytosis scheme). Of note, the extensive tissue tropism of VSV enables this virus to be therefore used as an anti-cancer agent in all types of tumors.Citation81
Other viruses could enter cells by more than one mechanism that implies the passage from cell-surface through different membrane structures ( and Viral endocytosis scheme). The enveloped influenza retrovirus mainly enters cells by clathrin-mediated endocytosis, but it can also follow a clathrin-independent pathway,Citation86 which appears to be regulated by Epsin 1.Citation87 Moreover, simian virus 40 (SV40) is alternatively internalized either via caveolaeCitation88 or a CLICs/GEECs (clathrin-independent carriers/glycosylphosphatidylinositol-anchored proteins and enriched early endosomal compartments)-driven pathway.Citation3,Citation89,Citation90 The capsid of the non-enveloped DNA SV40 virus is composed of VP1 homopentamers, which resemble cholera toxin B pentamers, and like the cholera toxin B subunit, SV40 binds gangliosides on the cell surface and enters via a raft/caveolar pathway.Citation2,Citation81,Citation90,Citation91 Echovirus 1, a picornavirus that binds to integrins, follows a caveolar/raft uptake process that involves protein kinase C, and penetration seems to occur in caveosomes without the involvement of the endoplasmic reticulum (ER).Citation2,Citation92,Citation93 For some viruses, such as for Ebola virus, SARS coronavirus (that causes severe acute respiratory syndrome [SARS]), and the non-enveloped mammalian reoviruses, an acidic pH alone is not sufficient to induce virus-endosome fusion and entry, thus participation of cathepsins L and B acid-dependent endosomal proteases are required to acquire the penetration-competent state of viral proteins.Citation94–Citation96
It has been described for certain viruses, such as HIV-1, VSV, murine leukemia virus, human papillomavirus type 16 and the DNA-enveloped vaccinia virus, that before entry into their target cells these viruses interact with and surf upon cell-surface filopodia toward the base of the filopodia,Citation97–Citation99 where certain virus enter cells by macropinocytosis, as echovirus 1 and adenovirus serotype 3,Citation100–Citation102 or by triggering the formation of transient membrane blebsCitation99 ( and Surfing on filopodia in Viral fusion and entry scheme, and surfing in viral synapse).
Altogether, the viral endocytic route could represent an easy way to overcome the barrier that cortical cytoskeleton imposes to the virus during early entry and infection processes, being also of importance to evade the immune responses against viruses.Citation2,Citation3,Citation81
Viral Replication and Membrane Dynamics
Intracellular membrane dynamics is not only essential during the first stages of viral fusion and subsequent entry but also to accomplish the viral replicative process. Replication of many viruses is associated with specific intracellular compartments so-called viral factories (VF; see VF schemes in ). These are thought to provide a physical scaffold to concentrate viral components for genome replication and morphogenesis.Citation8,Citation103,Citation104 The formation of VF often results in rearrangement of cellular membranes, reorganization of the cytoskeleton and recruitment of mitochondria.Citation105,Citation106 One of the early events in factory formation is the assembly of replication complexes (RCs) that associates with membranes derived from the ER, as observed in the case of flaviviruses, hepaciviruses, coronaviruses, arteriviruses and picornaviruses ( and VF schemes). On the other hand, RC of Togaviruses associate with membranes of endocytic origin, whereas RC of nodaviruses associate with mitochondrial membranes (reviewed in ref. Citation8). In fact, RNA viruses modify specific membranes of the factory to concentrate viral replicases and necessary cofactors for a more efficient replication of the viral genome.Citation107–Citation113 In this regard, rubella virus (RUBV), an important human teratogenic virus and the only member of the genus Rubivirus in the Togaviridae family,Citation114 and Semliki Forest virus (SFV),Citation115 anchors its RNA synthesis in membranes of a cell organelle known as the cytopathic vacuole (CPV),Citation116–Citation118 which derives from modified endosomes and lysosomes.Citation109,Citation117,Citation119 In fact, ER cisternae, mitochondria and Golgi stacks are recruited around CPVs to build RUBV factoriesCitation106 ( and VF schemes), which help the virus to evade host cell defense responses as well as connecting viral replication with assembly and maturation of new viral particles in recruited Golgi membranes.Citation120 The Golgi apparatus is a highly dynamic organelle with functional and sustained membrane and protein flowCitation121 and serves as a morphogenic mold for rubiviruses and other viruses, such as coronaviruses, arteriviruses and Bunyaviruses.Citation8,Citation105,Citation106,Citation120,Citation122,Citation123 The surface of CPVs consists of small vesicular invaginations or spherules (the sites of viral RNA replication) that line the vacuole membrane at regular intervals.Citation109,Citation117–Citation119,Citation124 These CPV structures also exhibit a variety of complex contacts with the endocytic pathway through its internal membranes that are interconnected with different transport vesicles.Citation106 A remarkably case of VF occurs with the poxvirus vaccinia virus, an example of DNA-virus that does not replicate inside the nucleus of host cells. Thus, discrete cisternae derived from the rough ER enclose the cytoplasmic site of viral-DNA replication and it is thought to eventually resemble a cytoplasmic mini-nucleus for viral replication.Citation125–Citation127
Other RNA+ viruses that belong to the Flaviviridae family and the Nidovirales order induce the formation of spherical, double-membrane vesicles (DMVs) to replicateCitation128–Citation130 ( and double-membrane vesicles scheme), while polioviruses-RNA polymerase molecules can also assemble bidimensional arrays.Citation110,Citation131 In fact, one of the best-studied viruses that induce membrane rearrangements is the human pathogen poliovirus, a member of the Picornaviridae family that causes poliomyelitis. Thus the trafficking and alteration of intracellular membrane structures regulate poliovirus infection, where all cellular organelles except mitochondria are virtually converted into virus replication vesicles.Citation132–Citation136 Indeed, endosomes and lysosomes (Togaviruses), peroxisomes and chloroplasts (members of the genus Tombusvirus) and mitochondria (nodaviruses) represents protective environments used as sites for RNA replication, while all plus-strand RNA viruses replicate in association with cytoplasmic membranes of infected cells.Citation107 Some data about the antiviral effect of brefeldin A (BFA) suggest the functional involvement of membrane trafficking proteins on virus replication of Enteroviruses, such as for the picornaviruses poliovirus and coxsackievirus.Citation137 BFA inhibits Arf-GTP exchange proteins (Arf-GEFS), reduces the Arf1-GTP needed to generate COPI coats in the Golgi and blocks the recruitment of membranes into replication compartments.Citation133 Thus sequestration of Arf-1 into the replication complex would also explain the block in secretion seen in infected cells.Citation138 However, BFA does not prevent formation of densely packed vesicles by poliovirus, so vesicle formation does not require activated Arf-GEF proteins.Citation136 Several picornaviruses are resistant to BFA, and their 3A proteins do not slow ER to Golgi transport as occurred with foot-and-mouth disease virus.Citation139–Citation141 For these viruses COPII coated vesicles may provide membranes for replication,Citation142–Citation145 while other studies implicate a role for autophagosomes.Citation146
The induction of coated-pit formation has also been observed for reovirus and SFV.Citation2,Citation147 Internalized SFV early recruits the intermediate-endosome Rab7-small GTPaseCitation148 to later induce the formation of CPVs, important for viral RNA synthesis,Citation118 which are derived from late endocytic compartments. In the case of HCV virus, it seems that recruitment of membrane trafficking proteins to ER-derived membrane scaffolds is key for viral replication. Hence, the NS5A protein is anchored to the cytoplasmic face of this membrane web to recruit the RNA-dependent RNA polymerase, NS5B. Both NS5A and NS5B bind VAMP associated proteins (VAPs) Citation149,Citation150 and recruit Rab1, Rab5 and Rab7 small GTPases that could direct the transport of vesicles to fuse with or enlarge this viral replicative membrane-web compartment.Citation9,Citation151–Citation153 It is conceivable that other RNA+ viruses such as Norwalk virus take advantage of the formation of ER-derived membrane scaffolds to replicate, as observed with the membrane-bound nsp48 protein that also binds VAP-A.Citation9,Citation154
The cell-host plasma membrane undergoes an immense rearrangement and a deformability process during viral budding, a key event of the life cycle of enveloped viruses, thereby determining viral morphology and infectiveness (reviewed in ref. Citation10) ( and , Budding schemes). The majority of studied enveloped viruses bud from cells by co-opting the host ESCRT machinery,Citation155–Citation159 critical for budding of vesicles in multivesicular bodies (MVBs) that are important intermediates in endolysosomal transport.Citation10,Citation160,Citation161 The HIV-1 viral polyprotein Gag binds, through its C-terminal region, both to cellular ESCRT-I complex and to ALIX protein, which both recruit ESCRT-III complex to the budding site to catalyze the scission of nascent virions, an event that it is thought to be carried out by the same process as for cleavage of intralumenal vesicles in MVBs.Citation10,Citation162
Furthermore, the tumor susceptibility gene 101 (Tsg101) subunit of the ESCRT-I complex, which mediates receptor sorting into MVBs,Citation163,Citation164 appears to be dispensable for viral envelope fusion with endosomal membranes and viral RNA transport to late endosomes, but is necessary for infection and RNA release.Citation85 These data indicate that Tsg101, in addition to its role in receptor sorting into MVBs, may well play a direct role in the release of nucleocapsids from within MVBs to the cytoplasm by controlling the back-fusion process. However, several recently reported data indicate that the interaction of Gag viral protein with the ESCRT machinery is dispensable for HIV-1 viral budding and infectiveness.Citation165–Citation167 In this regard, the matrix protein of the enveloped influenza virus lacks an ESCRT binding domain, thus it buds in an ESCRT-independent manner.Citation168,Citation169
In turn, the HIV-1 Gag localizes on PIP2-enriched plasma membrane regions, where PIP2 plays a critical role in HIV-1 particle assembly.Citation170,Citation171 Overexpression of polyphosphoinositide-5-phosphatase IV (5ptaseIV), which hydrolyzes the phosphate at the D5 position and reduces plasma membrane PIP2 levels,Citation172 drastically impairs HIV-1 release by relocalizing the viral polypeptide Gag from the plasma membrane to CD63-positive intracellular compartments.Citation171 Indeed, disrupting PIP2 levels and localization by expression of Arf6/Q67L, a GTP-bound mutant of Arf6, reduces virus release by retargeting Gag to newly formed PIP2-enriched endosomal vesicles.Citation171
Finally, other viruses bud from plasma membrane of host cells through their matrix viral protein. This is the case of Newcastle disease virus (a Paramyxovirus) and VSV virus, where bud formation and scission from membranes are both matrix-dependent processes.Citation173–Citation175
Viral Spread: Membrane Dynamics at the Virological Synapse
There is growing evidence that a number of different viruses can exploit pre-existing mechanisms of physiological communication between cells to facilitate direct cell-cell viral spread.Citation38 Studies revealing the co-clustering of viral egress machinery and viral receptors at the interface between conjugates of infected and uninfected cells respectively, have led to the definition of this structure as virological synapse (VS) Citation176,Citation177 ( and Viral synapse scheme). The first detailed description of VS was reported for the human T cell leukemia virus type 1 (HTLV-1),Citation178 and was then translated to the HIV field.Citation179 The cell-free form of HTLV-1 is very inefficient at infecting T cells and is spread between, and within individuals by strictly dependent cell-to-cell transmission mechanisms. Noteworthy, cell-to-cell viral spread presents numerous advantages for the virus as compared to cell-free virus infections, which could be summarized as follows:
A more rapid replication kinetics is obtained when viruses are transmitted across VS due to the higher concentration of viral particles released at the point of contact between the cell partners thus obviating the rate-limiting step of fluid-phase diffusion of free viral particles.Citation180
Infected cells entering a new host could adhere to, and cross by transmigration, a mucosal epithelial barrier that would otherwise be impermeable to cell free virions.Citation38
The formation of stable junctions whereby viruses traffic, shields the virus from immune response or drugs both sterically and kinetically in terms of exposure time although it is still unclear whether this is also the case for HIV.Citation181,Citation182
At the molecular level, VS synchronize both viral egress and entry processes in the synaptic cleft. Most recent literature suggests that HIV-1 cell-to-cell transmission results from microtubules-mediated polarized and massive HIV budding and subsequent entry of viral particles into target cellsCitation182,Citation183 ( and Viral synapse scheme). Therefore, most of the mechanisms that regulate cell-free virus entry by perturbing membrane dynamics also apply for cell-to-cell virus transmission. Indeed, it is known that membrane-cholesterol sequestering agentsCitation184 and inhibitors of cytoskeleton motilityCitation185 interfere with retroviral transmission between infected and uninfected cells. Similarly, the blockade of Arf6-coordinated plasma membrane dynamics by siRNA-Arf6 silencing or expression of dominant mutants has detrimental effects on cell-to-cell HIV-1 transmissionCitation51 ( and Arf6-dependent viral fusion and entry scheme).
Despite these similarities, some specific events of synaptic contacts that involve membrane dynamics should be also considered. It is well known that massive viral endocytosis accompanies viral entry during cell-to-cell HIV transmissionCitation186 (). In contrast, HTLV-1 appears to be transmitted from biofilm-like structures that accumulate viral particles on the surface of infected cells.Citation187 Other viruses have developed alternative strategies, pseudo-rabies virus transferred from cellular projections, HSV are transported within long membrane protrusions towards adjacent cells, and murine leukaemia virus moves along the surface of filopodia before viral entry at the cell body of fibroblastsCitation188 ( and surfing scheme at Viral synapse). Such filopodia that also participate in HIV transmission extend through receptor-mediated mechanisms from uninfected towards the infected cell.Citation188 A similar retroviral surfing has been described over narrower membranous structures called nanotubes that can connect cells separated by up to 100 mm,Citation189 which also transfer viral proteins at the inner inside. In this case, receptor specificity seems to play a minor role, as these actin-driven structures seems to extend from HIV infected cells in the absence of receptor-envelope interactions ( and Nanotubes scheme).
The formation of different bridging tubular structures within the synapses, usually involve huge membrane invaginations engulfing cellular fragments,Citation183 which may lead to the exchange of membranes between counteracting cells, a synapse-specific event that is known as trogocytosis.Citation190 Trogocytic exchange of membrane patches, initially described during cellular contacts among cells of the immune system, has been proposed as a possible mechanism to control the length and the stability of the synapse and therefore regulate its outcome.Citation190 Recently, trogocytosis has been reported to occur at the HIV induced VS, a process dependent on gp120 binding to CD4. Interestingly, HIV particles as well as membrane components (such as CD4 molecules) were transferred in an unidirectional way from the uninfected towards the infected cellsCitation182 ( and Trogocytosis scheme). This mechanism could have a huge impact rendering cells permissive to HIV infection as suggested in a recent study.Citation191
Besides the direct transfer from infected to uninfected cells, several pathogens take advantage of other mechanisms of immune communication between antigen presenting cells, mainly dendritic cells (DCs) and T cells. As DCs are professional pathogen hunters, several viruses, such as HIV or cytomegalovirus (CMV), have developed strategies to stably associate in an infectious state to these cells and infect lymphocytes during antigen presentation.Citation192,Citation193 HIV has been shown to reside in CD81 enriched salk-like structures inside DC that are released at the synaptic space during T cell scanning or antigen presentation.Citation193 Interestingly, the journey of HIV in DCs appears to be reminiscent of the exosomal pathway of antigen presentation ( and , Exosome schemes), suggesting that HIV hijacks this cellular mechanism in its own benefit and highlighting the similarities between retroviruses and exosomes.Citation194 Importantly, multiple pathogens, including some bacteria and other parasites, hijack the complex mechanisms of recognition and capture by DC to increase their spread efficiency in the infected hosts.Citation195
Conclusions and Perspectives
This article attempt a non-comprehensive review about the journey that different viruses accomplish to successfully infect their target cells, describing viral entry pathways and intracellular trafficking, from cell-surface and through complex and dynamic cell-membrane structures, which often are newly organized by the viruses in order to replicate. The different host intracellular compartments, such as ER, endosomes, lysosomes or mitochondria, serve as an adaptable membrane source to form viral replication factories and allow viruses to adopt their own functional morphology with the appropriated lipid composition. Indeed, it is conceivable that these membrane structures help to confine the process of RNA replication to a specific cytoplasmic location, just preventing the activation of certain host defense mechanisms that can be triggered by the viral genome during unwinding and replication.
Although several data have been reported about the functional role of membrane dynamics during viral infection, many related questions remain to be addressed. For example, how could different viral proteins, from divergent viruses and host cells, gain the ability to modify the same intracellular membrane compartments to assure viral replication, without critically affecting important cellular processes? In turn, why viruses choose different subcellular-membrane compartments for their replication? And, how viral proteins are targeted to and moving across those membranes and what host factors are recruited or involved in these events? The answer of these questions will surely contribute to our understanding of some of the basic mechanisms that control membrane dynamics, both in cellular functions and viral infection cycle. This may open the door to the design of new antiviral strategies aimed at effectively target the dynamic of viral-cell interactions, which hopefully would lead to new therapies for combat viral infections, such as HIV-1 infection and Acquired Immunodeficiency Disease Syndrome (AIDS). Moreover, it may also provide new rational designs by which non-viral gene delivery systems can be improved and therapeutically used in different gene-originated tumor and immune diseases.
Finally, the two- and three-dimensional study of viral fusion, entry and trafficking at the level of cells and virus particles will bring the technical development of new and more powerful microscopy devices, from fluorescent and high-end light microscopy and total internal reflection fluorescence microscopy (TIRFM) to transmission electron microscopy (TEM) with cellular electron tomography (ET),Citation51,Citation106,Citation196–Citation199 which allow to analyze these events with increased spatial and temporal resolution, in order to understand how viruses move through the different complex and dynamic cell-membrane structures to infect and survive.
Figures and Tables
Figure 1 Membrane dynamics processes in host cells that are involved in the life cycle of viral infection. The schematic representation of a cell and the different intracellular membrane-compartments, constitutive or putative sites formed by viruses to accomplish their infection processes, are shown. The non-regenerative viral membrane of enveloped viruses and the dynamics cell-host membranes play an important role during early infection process, since these two opposed membranes need to fuse, either at cell-surface or in an endocytic route (clathrin-, caveolae- or pinocytosis-dependent), to promote viral entry and infection. Endocytosis is initiated at the plasma membrane and progress through early and late endosomes, where some viruses replicate and are recycled back to the plasma membrane or transported to lysosomes to complete the life cycle. In the case of HIV-1, this enveloped virus requires Arf6-membrane dynamics to efficiently fuse with plasma membrane and promote entry and infection of CD4+ T lymphocytes (Asterisk scheme and ). The non-endocytic route followed by HIV-1 during early infection is decisive to establish viral latent infection. Once inside the cell, many viruses accomplish their replication process through exploiting or modulating membrane traffic, and generating specialized compartments to assure viral survival, such as Viral Factories (VF), multivesicular bodies (MVB), double-membrane compartments, budding on plasma membrane and exosomes (it is conceivable that some viruses may actually be released as exosomes ). These membrane structures, cell-constitutive or arranged by the different viral proteins, are required for viral-gene replication, morphogenesis, export, viral maturation and release from cell-surface, and also serve to evade the immune responses against viral genomes. Viral proteins could enter the secretory pathway by co-translational translocation into the endoplasmic reticulum (ER; only a part of the perinuclear ER is shown), to be further transported from the ER to the Golgi complex in vesicles and in a coatomer protein complex (COP) II-dependent manner. Viral complexes formed inside MVBs, in communication with vesicles, mitochondria, Golgi cisternae and ER-membranes, could be transported through the Golgi network to the plasma membrane to be released as viral particles. Viral budding of enveloped viruses is mainly under the control of the activity of ESCRT-III complexes that are recruited to the site of viral release by ESCRT-I or Alix proteins that interacts with matrix viral proteins located on cell-surface.
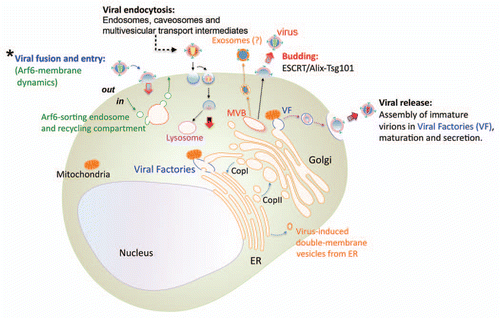
Figure 2 Arf6-membrane dynamics regulates efficient HIV-1 infection. HIV-1 requires Arf6-coordinated membrane dynamics to efficiently fuse with plasma membrane and promote entry and infection of CD4+ T lymphocytes. In fact, movement of PIP2-associated membrane structures, driven by the Arf6-GTP/GDP cycle activity on plasma membrane from a sorting and recycling endosomal compartment, assures the regeneration of cell-surface membrane by coordinating the turnover of these PIP2-associated vesicles. This membrane traffic has synergy with the key first HIV-1/receptors interactions to promote pore fusion formation, between the non-regenerative HIV-1 viral membrane and the dynamic cell-surface, thereby favouring efficient virus-cell fusion, entry and infection (scheme corresponding to early fusion and entry steps of the HIV-1 infection process, also indicated in by an asterisk). The alteration of the Arf6-GTP/GDP cycle, by GDP-bound or GTP-bound mutants provokes an accumulation of Arf6/PIP2-membrane structures on the plasma membrane. Specific Arf6 silencing also inhibits HIV-1-envelope-induced membrane fusion, entry and infection of T lymphocytes and permissive cells, regardless of viral tropism.
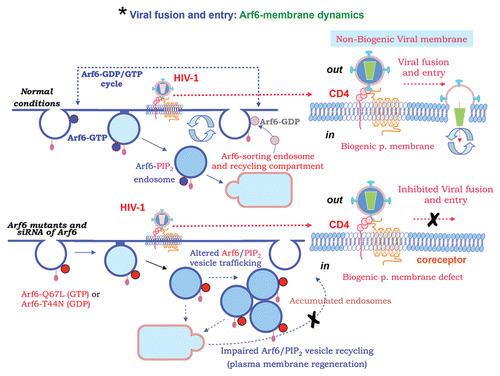
Figure 3 Membrane dynamics at the virological synapse. At the virological synapse (VS), some viruses either attach to the plasma membrane or surf along the filopodia and finally bind to specific receptors on the target cell. Viruses can also directly fuse with the plasma membrane, as in the case of HIV-1. Cell-to-cell transfer of HIV-1 takes place in an Arf6-membrane dynamics-dependent manner, which hijack endocytic pathways, including clathrin-dependent, caveolin-dependent or both independent pathways for viral internalization. The VS represents an efficient environment for viral budding, where the membrane of the infected cell is polarized towards the synaptic junction by the movement for of vesicles or MVBs coordinated by the translocation of the microtubule organizing centre (MTOC). This scaffolding allows for a subsequent viral infection and spread, that favours viral fusion and entry, viral endocytosis and viral protein/gene transfer from the infected to the close non-infected cell. Besides, long membrane nanotubes may also be formed between neighboring cells, which promote viral protein traffic and also HIV surfing and infection, from infected cell to non-infected cell. Other membrane dynamics events involved or occurred during viral infection and spreading are Trogocytosis and exosomal transport. Trogocytosis of cell-surface patches, containing CD4/HIV-1-bound molecules, occurs from non-infected to infected cells in a gp120/CD4-dependent manner. Exosomes are membrane vesicles, formed from MVB that could account for viral infection and spreading within membrane structures that are protected from immune responses.
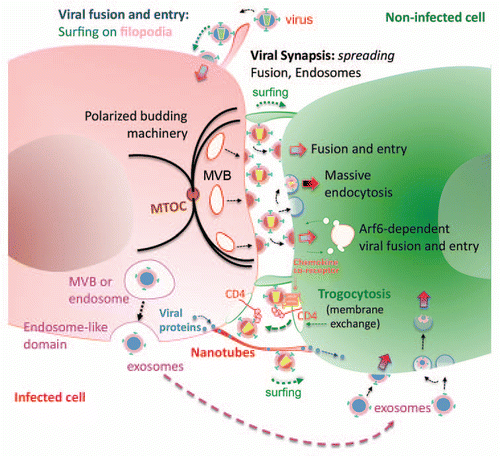
Acknowledgments
This work and A.V.F. are supported by SAF2008-01729 (MICINN, Spain), European Regional Development Fund (ERDF), 24661/07 and 24-0740-09 (Fundacion Investigacion y Prevencion del SIDA en España), and ProID20100020 (Agencia Canaria de Investigación, Innovación y Soc. Información; Gobierno de Canarias) grants. The HIVACAT Program, the FIS project PI08/1306 and the Spanish AIDS network (RD06/0006). J.B.G., L.G.E. and L. de A.R. are supported by FIPSE-24-0740-09, SAF2008-01729 and ProID20100020 associated fellowships, respectively. J.D.M. is supported by the Ramón y Cajal program (R&C-2010-06256, MICINN). J.B. is supported by the ISCIII and Health Department (Generalitat de Catalunya). The authors declare that they have no conflicting financial interests. We specially thank to Prof. Manuel Feria for critical reading of the manuscript and for his continuous and generous support. We apologize for all research works and reviews that we have not reported or considered in this minireview, where we have tried to avoid omissions by our unpremeditated unknowledge.
References
- Greber UF, Way M. A superhighway to virus infection. Cell 2006; 124:741 - 754
- Marsh M, Helenius A. Virus entry: open sesame. Cell 2006; 124:729 - 740
- Gruenberg J. Viruses and endosome membrane dynamics. Curr Opin Cell Biol 2009; 21:582 - 588
- Bajno L, Peng XR, Schreiber AD, Moore HP, Trimble WS, Grinstein S. Focal exocytosis of VAMP3-containing vesicles at sites of phagosome formation. J Cell Biol 2000; 149:697 - 706
- Nordenfelt P, Winberg ME, Lonnbro P, Rasmusson B, Tapper H. Different requirements for early and late phases of azurophilic granule-phagosome fusion. Traffic 2009; 10:1881 - 1893
- Faurschou M, Borregaard N. Neutrophil granules and secretory vesicles in inflammation. Microbes Infect 2003; 5:1317 - 1327
- Maganto-Garcia E, Punzon C, Terhorst C, Fresno M. Rab5 activation by Toll-like receptor 2 is required for Trypanosoma cruzi internalization and replication in macrophages. Traffic 2008; 9:1299 - 1315
- Miller S, Krijnse-Locker J. Modification of intracellular membrane structures for virus replication. Nat Rev Microbiol 2008; 6:363 - 374
- Pierini R, Cottam E, Roberts R, Wileman T. Modulation of membrane traffic between endoplasmic reticulum, ERGIC and Golgi to generate compartments for the replication of bacteria and viruses. Semin Cell Dev Biol 2009; 20:828 - 833
- Hurley JH, Boura E, Carlson LA, Rozycki B. Membrane budding. Cell 2010; 143:875 - 887
- Mudhakir D, Harashima H. Learning from the viral journey: how to enter cells and how to overcome intracellular barriers to reach the nucleus. Aaps J 2009; 11:65 - 77
- Sodeik B. Mechanisms of viral transport in the cytoplasm. Trends Microbiol 2000; 8:465 - 472
- Doms RW, Trono D. The plasma membrane as a combat zone in the HIV battlefield. Genes Dev 2000; 14:2677 - 2688
- Waheed AA, Freed EO. Lipids and membrane microdomains in HIV-1 replication. Virus Res 2009; 143:162 - 176
- Stein BS, Gowda SD, Lifson JD, Penhallow RC, Bensch KG, Engleman EG. pH-independent HIV entry into CD4-positive T cells via virus envelope fusion to the plasma membrane. Cell 1987; 49:659 - 668
- Stein BS, Engleman EG. Mechanism of HIV-1 entry into CD4+ T cells. Adv Exp Med Biol 1991; 300:71 - 86
- Pelchen-Matthews A, Clapham P, Marsh M. Role of CD4 endocytosis in human immunodeficiency virus infection. J Virol 1995; 69:8164 - 8168
- Wyatt R, Sodroski J. The HIV-1 envelope glycoproteins: fusogens, antigens and immunogens. Science 1998; 280:1884 - 1888
- Earp LJ, Delos SE, Park HE, White JM. The many mechanisms of viral membrane fusion proteins. Curr Top Microbiol Immunol 2005; 285:25 - 66
- Harrison SC. Mechanism of membrane fusion by viral envelope proteins. Adv Virus Res 2005; 64:231 - 261
- Behnia R, Munro S. Organelle identity and the sign-posts for membrane traffic. Nature 2005; 438:597 - 604
- Valenzuela-Fernandez A, Cabrero JR, Serrador JM, Sanchez-Madrid F. HDAC6: a key regulator of cytoskeleton, cell migration and cell-cell interactions. Trends Cell Biol 2008; 18:291 - 297
- Albiges-Rizo C, Destaing O, Fourcade B, Planus E, Block MR. Actin machinery and mechanosensitivity in invadopodia, podosomes and focal adhesions. J Cell Sci 2009; 122:3037 - 3049
- Mogilner A, Keren K. The shape of motile cells. Curr Biol 2009; 19:762 - 771
- Pollard TD, Cooper JA. Actin, a central player in cell shape and movement. Science 2009; 326:1208 - 1212
- Saarikangas J, Zhao H, Lappalainen P. Regulation of the actin cytoskeleton-plasma membrane interplay by phosphoinositides. Physiol Rev 2010; 90:259 - 289
- Mellman I. Quo vadis: polarized membrane recycling in motility and phagocytosis. J Cell Biol 2000; 149:529 - 530
- Mellman I, Warren G. The road taken: past and future foundations of membrane traffic. Cell 2000; 100:99 - 112
- Heck JN, Mellman DL, Ling K, Sun Y, Wagoner MP, Schill NJ, et al. A conspicuous connection: structure defines function for the phosphatidylinositol-phosphate kinase family. Crit Rev Biochem Mol Biol 2007; 42:15 - 39
- Folsch H, Mattila PE, Weisz OA. Taking the scenic route: biosynthetic traffic to the plasma membrane in polarized epithelial cells. Traffic 2009; 10:972 - 981
- Shibata Y, Hu J, Kozlov MM, Rapoport TA. Mechanisms shaping the membranes of cellular organelles. Annu Rev Cell Dev Biol 2009; 25:329 - 354
- Iyengar S, Hildreth JE, Schwartz DH. Actin-dependent receptor colocalization required for human immunodeficiency virus entry into host cells. J Virol 1998; 72:5251 - 5255
- Doms RW. Beyond receptor expression: the influence of receptor conformation, density and affinity in HIV-1 infection. Virology 2000; 276:229 - 237
- Kuhmann SE, Platt EJ, Kozak SL, Kabat D. Cooperation of multiple CCR5 coreceptors is required for infections by human immunodeficiency virus type 1. J Virol 2000; 74:7005 - 7015
- Jimenez-Baranda S, Gomez-Mouton C, Rojas A, et al. Filamin-A regulates actin-dependent clustering of HIV receptors. Nat Cell Biol 2007; 9:838 - 846
- Yoder A, Yu D, Dong L, Iyer SR, Xu X, Kelly J, et al. HIV envelope-CXCR4 signaling activates cofilin to overcome cortical actin restriction in resting CD4 T cells. Cell 2008; 134:782 - 792
- Barrero-Villar M, Cabrero JR, Gordón-Alonso M, Barroso-González J, Alvarez-Losada S, Muñoz-Fernández MA, et al. Moesin is required for HIV-1-induced CD4-CXCR4 interaction, F-actin redistribution, membrane fusion and viral infection in lymphocytes. J Cell Sci 2009; 122:103 - 113
- Sattentau Q. Avoiding the void: cell-to-cell spread of human viruses. Nat Rev Microbiol 2008; 6:815 - 826
- Barrero-Villar M, Barroso-González J, Cabrero JR, Gordón-Alonso M, Alvarez-Losada S, Muñoz-Fernández MA, et al. PI4P5-kinase Ialpha is required for efficient HIV-1 entry and infection of T cells. J Immunol 2008; 181:6882 - 6888
- Liu Y, Belkina NV, Shaw S. HIV infection of T cells: actin-in and actin-out. Sci Signal 2009; 2:23
- Vorster PJ, Guo J, Yoder A, Wang W, Zheng Y, Xu X, et al. LIM kinase 1 modulates cortical actin and CXCR4 cycling and is activated by HIV-1 to initiate viral infection. J Biol Chem 2011; 286:12554 - 12564
- Valenzuela-Fernández A, Alvarez S, Gordon-Alonso M, Barrero M, Ursa A, Cabrero JR, et al. Histone deacetylase 6 regulates human immunodeficiency virus type 1 infection. Mol Biol Cell 2005; 16:5445 - 5454
- Malinowsky K, Luksza J, Dittmar MT. Susceptibility to virus-cell fusion at the plasma membrane is reduced through expression of HIV gp41 cytoplasmic domains. Virology 2008; 376:69 - 78
- Pauza CD, Price TM. Human immunodeficiency virus infection of T cells and monocytes proceeds via receptor-mediated endocytosis. J Cell Biol 1988; 107:959 - 968
- Marechal V, Prevost MC, Petit C, Perret E, Heard JM, Schwartz O. Human immunodeficiency virus type 1 entry into macrophages mediated by macropinocytosis. J Virol 2001; 75:11166 - 11177
- Daecke J, Fackler OT, Dittmar MT, Krausslich HG. Involvement of clathrin-mediated endocytosis in human immunodeficiency virus type 1 entry. J Virol 2005; 79:1581 - 1594
- Miyauchi K, Kim Y, Latinovic O, Morozov V, Melikyan GB. HIV enters cells via endocytosis and dynamin-dependent fusion with endosomes. Cell 2009; 137:433 - 444
- Carter GC, Bernstone L, Baskaran D, James W. HIV-1 infects macrophages by exploiting an endocytic route dependent on dynamin, Rac1 and Pak1. Virology 2011; 409:234 - 250
- Yu D, Wang W, Yoder A, Spear M, Wu Y. The HIV envelope but not VSV glycoprotein is capable of mediating HIV latent infection of resting CD4 T cells. PLoS Pathog 2009; 5:1000633
- Harmon B, Campbell N, Ratner L. Role of Abl kinase and the Wave2 signaling complex in HIV-1 entry at a post-hemifusion step. PLoS Pathog 2010; 6:1000956
- Garcia-Exposito L, Barroso-Gonzalez J, Puigdomenech I, Machado JD, Blanco J, Valenzuela-Fernandez A. HIV-1 requires Arf6-mediated membrane dynamics to efficiently enter and infect T lymphocytes. Mol Biol Cell 2011; 22:1148 - 1166
- Vidricaire G, Imbeault M, Tremblay MJ. Endocytic host cell machinery plays a dominant role in intracellular trafficking of incoming human immunodeficiency virus type 1 in human placental trophoblasts. J Virol 2004; 78:11904 - 11915
- Vidricaire G, Tremblay MJ. Rab5 and Rab7, but not ARF6, govern the early events of HIV-1 infection in polarized human placental cells. J Immunol 2005; 175:6517 - 6530
- Vidricaire G, Tremblay MJ. A clathrin, caveolae and dynamin-independent endocytic pathway requiring free membrane cholesterol drives HIV-1 internalization and infection in polarized trophoblastic cells. J Mol Biol 2007; 368:1267 - 1283
- Honda A, Nogami M, Yokozeki T, Yamazaki M, Nakamura H, Watanabe H, et al. Phosphatidylinositol-4-phosphate-5-kinase alpha is a downstream effector of the small G protein ARF6 in membrane ruffle formation. Cell 1999; 99:521 - 532
- Aikawa Y, Martin TF. ADP-ribosylation factor 6 regulation of phosphatidylinositol-4,5-bisphosphate synthesis, endocytosis and exocytosis. Methods Enzymol 2005; 404:422 - 431
- Brown FD, Rozelle AL, Yin HL, Balla T, Donaldson JG. Phosphatidylinositol-4,5-bisphosphate and Arf6-regulated membrane traffic. J Cell Biol 2001; 154:1007 - 1017
- Donaldson JG. Multiple roles for Arf6: sorting, structuring and signaling at the plasma membrane. J Biol Chem 2003; 278:41573 - 41576
- Wolf MC, Freiberg AN, Zhang T, Akyol-Ataman Z, Grock A, Hong PW, et al. A broad-spectrum antiviral targeting entry of enveloped viruses. Proc Natl Acad Sci USA 2010; 107:3157 - 3162
- Criss AK, Silva M, Casanova JE, McCormick BA. Regulation of Salmonella-induced neutrophil transmigration by epithelial ADP-ribosylation factor 6. J Biol Chem 2001; 276:48431 - 48439
- Smith AC, Cirulis JT, Casanova JE, Scidmore MA, Brumell JH. Interaction of the Salmonella-containing vacuole with the endocytic recycling system. J Biol Chem 2005; 280:24634 - 24641
- Lodge R, Descoteaux A. Phagocytosis of Leishmania donovani amastigotes is Rac1 dependent and occurs in the absence of NADPH oxidase activation. Eur J Immunol 2006; 36:2735 - 2744
- Nishi K, Saigo K. Cellular internalization of green fluorescent protein fused with herpes simplex virus protein VP22 via a lipid raft-mediated endocytic pathway independent of caveolae and Rho family GTPases but dependent on dynamin and Arf6. J Biol Chem 2007; 282:27503 - 27517
- Muschiol S, Normark S, Henriques-Normark B, Subtil A. Small molecule inhibitors of the Yersinia type III secretion system impair the development of Chlamydia after entry into host cells. BMC Microbiol 2009; 9:75
- Marchant D, Sall A, Si X, Abraham T, Wu W, Luo Z, et al. ERK MAP kinase-activated Arf6 trafficking directs coxsackievirus type B3 into an unproductive compartment during virus host-cell entry. J Gen Virol 2009; 90:854 - 862
- Laakkonen JP, Mäkelä AR, Kakkonen E, Turkki P, Kukkonen S, Peränen J, et al. Clathrin-independent entry of baculovirus triggers uptake of E. coli in non-phagocytic human cells. PLoS One 2009; 4:5093
- Radhakrishna H, Donaldson JG. ADP-ribosylation factor 6 regulates a novel plasma membrane recycling pathway. J Cell Biol 1997; 139:49 - 61
- Donaldson JG, Honda A. Localization and function of Arf family GTPases. Biochem Soc Trans 2005; 33:639 - 642
- Naslavsky N, Weigert R, Donaldson JG. Convergence of non-clathrin- and clathrin-derived endosomes involves Arf6 inactivation and changes in phosphoinositides. Mol Biol Cell 2003; 14:417 - 431
- D'Souza-Schorey C, Li G, Colombo MI, Stahl PD. A regulatory role for ARF6 in receptor-mediated endocytosis. Science 1995; 267:1175 - 1178
- Chavrier P, Goud B. The role of ARF and Rab GTPases in membrane transport. Curr Opin Cell Biol 1999; 11:466 - 475
- Al-Awar O, Radhakrishna H, Powell NN, Donaldson JG. Separation of membrane trafficking and actin remodeling functions of ARF6 with an effector domain mutant. Mol Cell Biol 2000; 20:5998 - 6007
- D'Souza-Schorey C, Chavrier P. ARF proteins: roles in membrane traffic and beyond. Nat Rev Mol Cell Biol 2006; 7:347 - 358
- Song J, Khachikian Z, Radhakrishna H, Donaldson JG. Localization of endogenous ARF6 to sites of cortical actin rearrangement and involvement of ARF6 in cell spreading. J Cell Sci 1998; 111:2257
- Martin TF. PI(4,5)P(2) regulation of surface membrane traffic. Curr Opin Cell Biol 2001; 13:493 - 499
- Luton F, Klein S, Chauvin JP, Le Bivic A, Bourgoin S, Franco M, et al. EFA6, exchange factor for ARF6, regulates the actin cytoskeleton and associated tight junction in response to E-cadherin engagement. Mol Biol Cell 2004; 15:1134 - 1145
- Donaldson JG. Arf6 and its role in cytoskeletal modulation. Methods Mol Biol 2002; 189:191 - 198
- Hernandez-Deviez DJ, Roth MG, Casanova JE, Wilson JM. ARNO and ARF6 regulate axonal elongation and branching through downstream activation of phosphatidylinositol-4-phosphate-5-kinase alpha. Mol Biol Cell 2004; 15:111 - 120
- Franco M, Peters PJ, Boretto J, van Donselaar E, Neri A, D'Souza-Schorey C, et al. EFA6, a sec7 domain-containing exchange factor for ARF6, coordinates membrane recycling and actin cytoskeleton organization. EMBO J 1999; 18:1480 - 1491
- Heikkilä O, Susi P, Tevaluoto T, Härmä H, Marjomäki V, Hyypiä T, et al. Internalization of coxsackievirus A9 is mediated by {beta}2-microglobulin, dynamin and Arf6 but not by caveolin-1 or clathrin. J Virol 2010; 84:3666 - 3681
- Lichty BD, Power AT, Stojdl DF, Bell JC. Vesicular stomatitis virus: re-inventing the bullet. Trends Mol Med 2004; 10:210 - 216
- Schlegel R, Tralka TS, Willingham MC, Pastan I. Inhibition of VSV binding and infectivity by phosphatidylserine: is phosphatidylserine a VSV-binding site?. Cell 1983; 32:639 - 646
- Coil DA, Miller AD. Phosphatidylserine is not the cell surface receptor for vesicular stomatitis virus. J Virol 2004; 78:10920 - 10926
- Le Blanc I, Luyet PP, Pons V, Ferguson C, Emans N, Petiot A, et al. Endosome-to-cytosol transport of viral nucleocapsids. Nat Cell Biol 2005; 7:653 - 664
- Luyet PP, Falguieres T, Pons V, Pattnaik AK, Gruenberg J. The ESCRT-I subunit TSG101 controls endosome-to-cytosol release of viral RNA. Traffic 2008; 9:2279 - 2290
- Rust MJ, Lakadamyali M, Zhang F, Zhuang X. Assembly of endocytic machinery around individual influenza viruses during viral entry. Nat Struct Mol Biol 2004; 11:567 - 573
- Chen C, Zhuang X. Epsin 1 is a cargo-specific adaptor for the clathrin-mediated endocytosis of the influenza virus. Proc Natl Acad Sci USA 2008; 105:11790 - 11795
- Pelkmans L, Kartenbeck J, Helenius A. Caveolar endocytosis of simian virus 40 reveals a new two-step vesicular-transport pathway to the ER. Nat Cell Biol 2001; 3:473 - 483
- Damm EM, Pelkmans L, Kartenbeck J, Mezzacasa A, Kurzchalia T, Helenius A. Clathrin- and caveolin-1-independent endocytosis: entry of simian virus 40 into cells devoid of caveolae. J Cell Biol 2005; 168:477 - 488
- Mayor S, Pagano RE. Pathways of clathrin-independent endocytosis. Nat Rev Mol Cell Biol 2007; 8:603 - 612
- Neu U, Woellner K, Gauglitz G, Stehle T. Structural basis of GM1 ganglioside recognition by simian virus 40. Proc Natl Acad Sci USA 2008; 105:5219 - 5224
- Pietiainen V, Marjomaki V, Upla P, Pelkmans L, Helenius A, Hyypia T. Echovirus 1 endocytosis into caveosomes requires lipid rafts, dynamin II and signaling events. Mol Biol Cell 2004; 15:4911 - 4925
- Upla P, Marjomäki V, Kankaanpää P, Ivaska J, Hyypiä T, Van Der Goot FG, et al. Clustering induces a lateral redistribution of alpha2beta1 integrin from membrane rafts to caveolae and subsequent protein kinase C-dependent internalization. Mol Biol Cell 2004; 15:625 - 636
- Chandran K, Sullivan NJ, Felbor U, Whelan SP, Cunningham JM. Endosomal proteolysis of the Ebola virus glycoprotein is necessary for infection. Science 2005; 308:1643 - 1645
- Ebert DH, Deussing J, Peters C, Dermody TS. Cathepsin L and cathepsin B mediate reovirus disassembly in murine fibroblast cells. J Biol Chem 2002; 277:24609 - 24617
- Simmons G, Gosalia DN, Rennekamp AJ, Reeves JD, Diamond SL, Bates P. Inhibitors of cathepsin L prevent severe acute respiratory syndrome coronavirus entry. Proc Natl Acad Sci USA 2005; 102:11876 - 11881
- Lehmann MJ, Sherer NM, Marks CB, Pypaert M, Mothes W. Actin- and myosin-driven movement of viruses along filopodia precedes their entry into cells. J Cell Biol 2005; 170:317 - 325
- Schelhaas M, Ewers H, Rajamaki ML, Day PM, Schiller JT, Helenius A. Human papillomavirus type 16 entry: retrograde cell surface transport along actin-rich protrusions. PLoS Pathog 2008; 4:1000148
- Mercer J, Helenius A. Vaccinia virus uses macropinocytosis and apoptotic mimicry to enter host cells. Science 2008; 320:531 - 535
- Karjalainen M, Kakkonen E, Upla P, Paloranta H, Kankaanpää P, Liberali P, et al. A Raft-derived, Pak1-regulated entry participates in alpha2beta1 integrin-dependent sorting to caveosomes. Mol Biol Cell 2008; 19:2857 - 2869
- Liberali P, Kakkonen E, Turacchio G, Valente C, Spaar A, Perinetti G, et al. The closure of Pak1-dependent macropinosomes requires the phosphorylation of CtBP1/BARS. EMBO J 2008; 27:970 - 981
- Amstutz B, Gastaldelli M, Kälin S, Imelli N, Boucke K, Wandeler E, et al. Subversion of CtBP1-controlled macropinocytosis by human adenovirus serotype 3. EMBO J 2008; 27:956 - 969
- Netherton C, Moffat K, Brooks E, Wileman T. A guide to viral inclusions, membrane rearrangements, factories and viroplasm produced during virus replication. Adv Virus Res 2007; 70:101 - 182
- Novoa RR, Calderita G, Arranz R, Fontana J, Granzow H, Risco C. Virus factories: associations of cell organelles for viral replication and morphogenesis. Biol Cell 2005; 97:147 - 172
- Fontana J, Lopez-Montero N, Elliott RM, Fernandez JJ, Risco C. The unique architecture of Bunyamwera virus factories around the Golgi complex. Cell Microbiol 2008; 10:2012 - 2028
- Fontana J, Lopez-Iglesias C, Tzeng WP, Frey TK, Fernandez JJ, Risco C. Three-dimensional structure of Rubella virus factories. Virology 2010; 405:579 - 591
- Salonen A, Ahola T, Kaariainen L. Viral RNA replication in association with cellular membranes. Curr Top Microbiol Immunol 2005; 285:139 - 173
- Dye BT, Miller DJ, Ahlquist P. In vivo self-interaction of nodavirus RNA replicase protein a revealed by fluorescence resonance energy transfer. J Virol 2005; 79:8909 - 8919
- Fontana J, Tzeng WP, Calderita G, Fraile-Ramos A, Frey TK, Risco C. Novel replication complex architecture in rubella replicon-transfected cells. Cell Microbiol 2007; 9:875 - 890
- Lyle JM, Bullitt E, Bienz K, Kirkegaard K. Visualization and functional analysis of RNA-dependent RNA polymerase lattices. Science 2002; 296:2218 - 2222
- Mackenzie J. Wrapping things up about virus RNA replication. Traffic 2005; 6:967 - 977
- Spagnolo JF, Rossignol E, Bullitt E, Kirkegaard K. Enzymatic and nonenzymatic functions of viral RNA-dependent RNA polymerases within oligomeric arrays. RNA 2010; 16:382 - 393
- Wang QM, Hockman MA, Staschke K, Johnson RB, Case KA, Lu J, et al. Oligomerization and cooperative RNA synthesis activity of hepatitis C virus RNA-dependent RNA polymerase. J Virol 2002; 76:3865 - 3872
- Frey TK. Molecular biology of rubella virus. Adv Virus Res 1994; 44:69 - 160
- Kujala P, Ikaheimonen A, Ehsani N, Vihinen H, Auvinen P, Kaariainen L. Biogenesis of the Semliki Forest virus RNA replication complex. J Virol 2001; 75:3873 - 3884
- Kujala P, Ahola T, Ehsani N, Auvinen P, Vihinen H, Kaariainen L. Intracellular distribution of rubella virus nonstructural protein P150. J Virol 1999; 73:7805 - 7811
- Lee JY, Marshall JA, Bowden DS. Characterization of rubella virus replication complexes using antibodies to double-stranded RNA. Virology 1994; 200:307 - 312
- Froshauer S, Kartenbeck J, Helenius A. Alphavirus RNA replicase is located on the cytoplasmic surface of endosomes and lysosomes. J Cell Biol 1988; 107:2075 - 2086
- Magliano D, Marshall JA, Bowden DS, Vardaxis N, Meanger J, Lee JY. Rubella virus replication complexes are virus-modified lysosomes. Virology 1998; 240:57 - 63
- Risco C, Carrascosa JL, Frey TK. Structural maturation of rubella virus in the Golgi complex. Virology 2003; 312:261 - 269
- James Morre D, Mollenhauer HH. Microscopic morphology and the origins of the membrane maturation model of Golgi apparatus function. Int Rev Cytol 2007; 262:191 - 218
- Salanueva IJ, Novoa RR, Cabezas P, López-Iglesias C, Carrascosa JL, Elliott RM, et al. Polymorphism and structural maturation of bunyamwera virus in Golgi and post-Golgi compartments. J Virol 2003; 77:1368 - 1381
- Novoa RR, Calderita G, Cabezas P, Elliott RM, Risco C. Key Golgi factors for structural and functional maturation of bunyamwera virus. J Virol 2005; 79:10852 - 10863
- Grimley PM, Levin JG, Berezesky IK, Friedman RM. Specific membranous structures associated with the replication of group A arboviruses. J Virol 1972; 10:492 - 503
- Cairns J. The initiation of vaccinia infection. Virology 1960; 11:603 - 623
- Kit S, Dubbs DR, Hsu TC. Biochemistry of vaccinia-infected mouse fibroblasts (strain L-M). III. Radioautographic and biochemical studies of thymidine-H3 uptake into DNA of L-M cells and rabbit cells in primary culture. Virology 1963; 19:13 - 22
- Tolonen N, Doglio L, Schleich S, Krijnse Locker J. Vaccinia virus DNA replication occurs in endoplasmic reticulum-enclosed cytoplasmic mini-nuclei. Mol Biol Cell 2001; 12:2031 - 2046
- Hall RA, Scherret JH, Mackenzie JS. Kunjin virus: an Australian variant of West Nile?. Ann NY Acad Sci 2001; 951:153 - 160
- Pedersen KW, van der Meer Y, Roos N, Snijder EJ. Open reading frame 1a-encoded subunits of the arterivirus replicase induce endoplasmic reticulum-derived double-membrane vesicles which carry the viral replication complex. J Virol 1999; 73:2016 - 2026
- van der Meer Y, van Tol H, Locker JK, Snijder EJ. ORF1a-encoded replicase subunits are involved in the membrane association of the arterivirus replication complex. J Virol 1998; 72:6689 - 6698
- Hobson SD, Rosenblum ES, Richards JC, Richmond K, Kirkegaard K, Schultz SC. Oligomeric structures of poliovirus polymerase are important for function. EMBO J 2001; 20:1153 - 1163
- Cherry S, Kunte A, Wang H, Coyne C, Rawson RB, Perrimon N. COPI activity coupled with fatty acid biosynthesis is required for viral replication. PLoS Pathog 2006; 2:102
- Belov GA, Ehrenfeld E. Involvement of cellular membrane traffic proteins in poliovirus replication. Cell Cycle 2007; 6:36 - 38
- Hogle JM. Poliovirus cell entry: common structural themes in viral cell entry pathways. Annu Rev Microbiol 2002; 56:677 - 702
- Bubeck D, Filman DJ, Hogle JM. Cryo-electron microscopy reconstruction of a poliovirus-receptor-membrane complex. Nat Struct Mol Biol 2005; 12:615 - 618
- Belov GA, Feng Q, Nikovics K, Jackson CL, Ehrenfeld E. A critical role of a cellular membrane traffic protein in poliovirus RNA replication. PLoS Pathog 2008; 4:1000216
- Maynell LA, Kirkegaard K, Klymkowsky MW. Inhibition of poliovirus RNA synthesis by brefeldin A. J Virol 1992; 66:1985 - 1994
- Doedens JR, Kirkegaard K. Inhibition of cellular protein secretion by poliovirus proteins 2B and 3A. EMBO J 1995; 14:894 - 907
- Wessels E, Duijsings D, Lanke KH, van Dooren SH, Jackson CL, Melchers WJ, et al. Effects of picornavirus 3A Proteins on Protein Transport and GBF1-dependent COP-I recruitment. J Virol 2006; 80:11852 - 11860
- Choe SS, Dodd DA, Kirkegaard K. Inhibition of cellular protein secretion by picornaviral 3A proteins. Virology 2005; 337:18 - 29
- Moffat K, Howell G, Knox C, Belsham GJ, Monaghan P, Ryan MD, et al. Effects of foot-and-mouth disease virus nonstructural proteins on the structure and function of the early secretory pathway: 2BC but not 3A blocks endoplasmic reticulum-to-Golgi transport. J Virol 2005; 79:4382 - 4395
- Gazina EV, Mackenzie JM, Gorrell RJ, Anderson DA. Differential requirements for COPI coats in formation of replication complexes among three genera of Picornaviridae. J Virol 2002; 76:11113 - 11122
- Rust RC, Landmann L, Gosert R, Tang BL, Hong W, Hauri HP, et al. Cellular COPII proteins are involved in production of the vesicles that form the poliovirus replication complex. J Virol 2001; 75:9808 - 9818
- Egger D, Bienz K. Intracellular location and translocation of silent and active poliovirus replication complexes. J Gen Virol 2005; 86:707 - 718
- Wei T, Wang A. Biogenesis of cytoplasmic membranous vesicles for plant potyvirus replication occurs at endoplasmic reticulum exit sites in a COPI- and COPII-dependent manner. J Virol 2008; 82:12252 - 12264
- Jackson WT, Giddings TH Jr, Taylor MP, Mulinyawe S, Rabinovitch M, Kopito RR, et al. Subversion of cellular autophagosomal machinery by RNA viruses. PLoS Biol 2005; 3:156
- Ehrlich M, Boll W, Van Oijen A, Hariharan R, Chandran K, Nibert ML, et al. Endocytosis by random initiation and stabilization of clathrin-coated pits. Cell 2004; 118:591 - 605
- Vonderheit A, Helenius A. Rab7 associates with early endosomes to mediate sorting and transport of Semliki forest virus to late endosomes. PLoS Biol 2005; 3:233
- Tu H, Gao L, Shi ST, Taylor DR, Yang T, Mircheff AK, et al. Hepatitis C virus RNA polymerase and NS5A complex with a SNARE-like protein. Virology 1999; 263:30 - 41
- Hamamoto I, Nishimura Y, Okamoto T, Aizaki H, Liu M, Mori Y, et al. Human VAP-B is involved in hepatitis C virus replication through interaction with NS5A and NS5B. J Virol 2005; 79:13473 - 13482
- Sklan EH, Serrano RL, Einav S, Pfeffer SR, Lambright DG, Glenn JS. TBC1D20 is a Rab1 GTPase-activating protein that mediates hepatitis C virus replication. J Biol Chem 2007; 282:36354 - 36361
- Stone M, Jia S, Heo WD, Meyer T, Konan KV. Participation of rab5, an early endosome protein, in hepatitis C virus RNA replication machinery. J Virol 2007; 81:4551 - 4563
- Manna D, Aligo J, Xu C, Park WS, Koc H, Heo WD, et al. Endocytic Rab proteins are required for hepatitis C virus replication complex formation. Virology 398:21 - 37
- Ettayebi K, Hardy ME. Norwalk virus nonstructural protein p48 forms a complex with the SNARE regulator VAP-A and prevents cell surface expression of vesicular stomatitis virus G protein. J Virol 2003; 77:11790 - 11797
- Chen BJ, Lamb RA. Mechanisms for enveloped virus budding: can some viruses do without an ESCRT?. Virology 2008; 372:221 - 232
- Welsch S, Muller B, Krausslich HG. More than one door—Budding of enveloped viruses through cellular membranes. FEBS Lett 2007; 581:2089 - 2097
- Bieniasz PD. Late budding domains and host proteins in enveloped virus release. Virology 2006; 344:55 - 63
- Morita E, Sundquist WI. Retrovirus budding. Annu Rev Cell Dev Biol 2004; 20:395 - 425
- Freed EO. Viral late domains. J Virol 2002; 76:4679 - 4687
- Gruenberg J, Stenmark H. The biogenesis of multivesicular endosomes. Nat Rev Mol Cell Biol 2004; 5:317 - 323
- Piper RC, Katzmann DJ. Biogenesis and function of multivesicular bodies. Annu Rev Cell Dev Biol 2007; 23:519 - 547
- Hurley JH, Hanson PI. Membrane budding and scission by the ESCRT machinery: it's all in the neck. Nat Rev Mol Cell Biol 2010; 11:556 - 566
- Saksena S, Emr SD. ESCRTs and human disease. Biochem Soc Trans 2009; 37:167 - 172
- Rodahl LM, Stuffers S, Lobert VH, Stenmark H. The role of ESCRT proteins in attenuation of cell signaling. Biochem Soc Trans 2009; 37:137 - 142
- Fujii K, Munshi UM, Ablan SD, Demirov DG, Soheilian F, Nagashima K, et al. Functional role of Alix in HIV-1 replication. Virology 2009; 391:284 - 292
- Zhang Y, Qian H, Love Z, Barklis E. Analysis of the assembly function of the human immunodeficiency virus type 1 gag protein nucleocapsid domain. J Virol 1998; 72:1782 - 1789
- Popova E, Popov S, Gottlinger HG. Human immunodeficiency virus type 1 nucleocapsid p1 confers ESCRT pathway dependence. J Virol 2010; 84:6590 - 6597
- Bruce EA, Medcalf L, Crump CM, Noton SL, Stuart AD, Wise HM, et al. Budding of filamentous and non-filamentous influenza A virus occurs via a VPS4 and VPS28-independent pathway. Virology 2009; 390:268 - 278
- Chen BJ, Leser GP, Morita E, Lamb RA. Influenza virus hemagglutinin and neuraminidase, but not the matrix protein, are required for assembly and budding of plasmid-derived virus-like particles. J Virol 2007; 81:7111 - 7123
- Chukkapalli V, Hogue IB, Boyko V, Hu WS, Ono A. Interaction between the human immunodeficiency virus type 1 Gag matrix domain and phosphatidylinositol-(4,5)-bisphosphate is essential for efficient gag membrane binding. J Virol 2008; 82:2405 - 2417
- Ono A, Ablan SD, Lockett SJ, Nagashima K, Freed EO. Phosphatidylinositol-(4,5)-bisphosphate regulates HIV-1 Gag targeting to the plasma membrane. Proc Natl Acad Sci USA 2004; 101:14889 - 14894
- Kisseleva MV, Wilson MP, Majerus PW. The isolation and characterization of a cDNA encoding phospholipid-specific inositol polyphosphate-5-phosphatase. J Biol Chem 2000; 275:20110 - 20116
- Shnyrova AV, Ayllon J, Mikhalyov II, Villar E, Zimmerberg J, Frolov VA. Vesicle formation by self-assembly of membrane-bound matrix proteins into a fluidlike budding domain. J Cell Biol 2007; 179:627 - 633
- Solon J, Gareil O, Bassereau P, Gaudin Y. Membrane deformations induced by the matrix protein of vesicular stomatitis virus in a minimal system. J Gen Virol 2005; 86:3357 - 3363
- Irie T, Licata JM, Jayakar HR, Whitt MA, Bell P, Harty RN. Functional analysis of late-budding domain activity associated with the PSAP motif within the vesicular stomatitis virus M protein. J Virol 2004; 78:7823 - 7827
- Piguet V, Sattentau Q. Dangerous liaisons at the virological synapse. J Clin Invest 2004; 114:605 - 610
- Jolly C, Sattentau QJ. Retroviral spread by induction of virological synapses. Traffic 2004; 5:643 - 650
- Igakura T, Stinchcombe JC, Goon PK, Taylor GP, Weber JN, Griffiths GM, et al. Spread of HTLV-I between lymphocytes by virus-induced polarization of the cytoskeleton. Science 2003; 299:1713 - 1716
- Jolly C, Kashefi K, Hollinshead M, Sattentau QJ. HIV-1 cell to cell transfer across an Env-induced, actin-dependent synapse. J Exp Med 2004; 199:283 - 293
- Dimitrov DS, Willey RL, Sato H, Chang LJ, Blumenthal R, Martin MA. Quantitation of human immunodeficiency virus type 1 infection kinetics. J Virol 1993; 67:2182 - 2190
- Martin N, Sattentau Q. Cell-to-cell HIV-1 spread and its implications for immune evasion. Curr Opin HIV AIDS 2009; 4:143 - 149
- Massanella M, Puigdomènech I, Cabrera C, Fernandez-Figueras MT, Aucher A, Gaibelet G, et al. Antigp41 antibodies fail to block early events of virological synapses but inhibit HIV spread between T cells. Aids 2009; 23:183 - 188
- Martin N, Welsch S, Jolly C, Briggs JA, Vaux D, Sattentau QJ. Virological synapse-mediated spread of human immunodeficiency virus type 1 between T cells is sensitive to entry inhibition. J Virol 2010; 84:3516 - 3527
- Jolly C, Sattentau QJ. Human immunodeficiency virus type 1 virological synapse formation in T cells requires lipid raft integrity. J Virol 2005; 79:12088 - 12094
- Jolly C, Mitar I, Sattentau QJ. Requirement for an intact T-cell actin and tubulin cytoskeleton for efficient assembly and spread of human immunodeficiency virus type 1. J Virol 2007; 81:5547 - 5560
- Puigdomenech I, Massanella M, Cabrera C, Clotet B, Blanco J. On the steps of cell-to-cell HIV transmission between CD4 T cells. Retrovirology 2009; 6:89
- Pais-Correia AM, Sachse M, Guadagnini S, Robbiati V, Lasserre R, Gessain A, et al. Biofilm-like extracellular viral assemblies mediate HTLV-1 cell-to-cell transmission at virological synapses. Nat Med 2010; 16:83 - 89
- Sherer NM, Lehmann MJ, Jimenez-Soto LF, Horensavitz C, Pypaert M, Mothes W. Retroviruses can establish filopodial bridges for efficient cell-to-cell transmission. Nat Cell Biol 2007; 9:310 - 315
- Sowinski S, Jolly C, Berninghausen O, Purbhoo MA, Chauveau A, Köhler K, et al. Membrane nanotubes physically connect T cells over long distances presenting a novel route for HIV-1 transmission. Nat Cell Biol 2008; 10:211 - 219
- Joly E, Hudrisier D. What is trogocytosis and what is its purpose?. Nat Immunol 2003; 4:815
- Aucher A, Puigdomenech I, Joly E, Clotet B, Hudrisier D, Blanco J. Could CD4 capture by CD8+ T cells play a role in HIV spreading?. J Biomed Biotechnol 2010; 2010:907371
- Halary F, Amara A, Lortat-Jacob H, Messerle M, Delaunay T, Houlès C, et al. Human cytomegalovirus binding to DC-SIGN is required for dendritic cell infection and target cell trans-infection. Immunity 2002; 17:653 - 664
- Izquierdo-Useros N, Naranjo-Gómez M, Erkizia I, Puertas MC, Borràs FE, Blanco J, et al. HIV and mature dendritic cells: Trojan exosomes riding the Trojan horse?. PLoS Pathog 2010; 6:1000740
- Izquierdo-Useros N, Puertas MC, Borras FE, Blanco J, Martinez-Picado J. Exosomes and retroviruses: the chicken or the egg?. Cell Microbiol 2011; 13:10 - 17
- van Kooyk Y, Geijtenbeek TB. DC-SIGN: escape mechanism for pathogens. Nat Rev Immunol 2003; 3:697 - 709
- Brandenburg B, Zhuang X. Virus trafficking—learning from single-virus tracking. Nat Rev Microbiol 2007; 5:197 - 208
- McIntosh R, Nicastro D, Mastronarde D. New views of cells in 3D: an introduction to electron tomography. Trends Cell Biol 2005; 15:43 - 51
- Kopek BG, Perkins G, Miller DJ, Ellisman MH, Ahlquist P. Three-dimensional analysis of a viral RNA replication complex reveals a virus-induced mini-organelle. PLoS Biol 2007; 5:220
- Diestra E, Fontana J, Guichard P, Marco S, Risco C. Visualization of proteins in intact cells with a clonable tag for electron microscopy. J Struct Biol 2009; 165:157 - 168