Abstract
In all the major secretory organs regulated exocytosis is a fundamental process that is used for releasing molecules in the extracellular space. Molecules destined for secretion are packaged into secretory vesicles that fuse with the plasma membrane upon the appropriate stimulus. In exocrine glands, large secretory vesicles fuse with specialized domains of the plasma membrane, which form ductal structures that are in direct continuity with the external environment and exhibit various architectures and diameters. In a recent study, we used intravital microscopy to analyze in detail the dynamics of exocytic events in the salivary glands of live rodents under conditions that cannot be reproduced in in vitro or ex vivo model systems. We found that after the opening of the fusion pore large secretory vesicles gradually collapse with their limiting membranes being completely absorbed into the apical plasma membrane canaliculi within 40–60 sec. Moreover, we observed that this controlled collapse requires the contractile activity of actin and its motor myosin II, which are recruited onto the large secretory vesicles immediately after their fusion with the plasma membrane. Here we suggest that the actomyosin complex may be required to facilitate exocytosis in those systems, such as the salivary glands, in which the full collapse of the vesicles is not energetically favorable due to a difference in membrane tension between the large secretory vesicles and the canaliculi.
Regulated exocytosis occurs in several organs with different modalities.Citation1-Citation3 Secretory vesicles can undergo: (1) full fusion, in which the limiting membrane of the secretory vesicle is completely absorbed into the plasma membrane (PM),Citation3,Citation4 (2) kiss and run, in which the fusion pore opens transiently, a quanta of the cargo molecule is released, and the vesicle detaches from the PM,Citation5,Citation6 and (3) compound exocytosis, where a secretory vesicle fuses with the PM and serves as a fusion site for other vesicles, generating strings of interconnected vesicles ().Citation3,Citation7-Citation9 Furthermore, the time a secretory vesicle spends at the PM after the fusion step varies considerably, ranging from few milliseconds, as in the case of small synaptic vesicles, up to minutes, as in the case of alveolar type II cells.Citation3 These differences suggest that the molecular machineries regulating the exocytic steps differ significantly among the various secretory systems, possibly to accommodate their different architectures.
Figure 1. Exocytosis of large secretory vesicles. A. Modality of exocytosis. Secretory vesicles may undergo exocytosis following three different modalities: (1) single fusion, (2) kiss and run, and (3) compound exocytosis. B. Diagram of a SGs acinus and organization of the APM. Acini are formed by pyramidal polarized epithelial cells that are in close contact and the APM (red) is shared by two cells and forms narrow canaliculi. The dashed boxes show two orthogonal enlargements of the apical area. The canaliculi have a diameter of 0.2‑0.3 µm and are separated from the basolateral membrane by tight junctions (green). The SCGs (blue) have a much larger diameter (1‑1.5 µm). When the fusion pore opens two compartments with different composition and membrane tension are interconnected (diagram on the right).
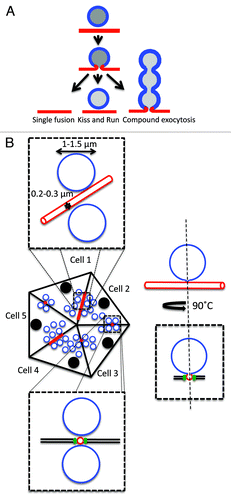
So far exocytosis has been extensively studied using both in vitro (i.e., cultured cells) and ex-vivo (i.e., organ cultures) experimental models. Although both systems continue to provide a plethora of important information, they suffer from two major limitations: (1) in in vitro systems, the geometry and the polarity of the secretory apparatus are not maintained, especially for exocrine models,Citation10-Citation13 and (2) in ex vivo systems, the lack of contribution from a series of signaling molecules provided by the vasculature and the central nervous system may have profound effects on the signaling pathways controlling exocytosis.Citation4,Citation14 For these reasons, it is crucial to study exocytosis in native tissue under the appropriate physiological conditions. Recently, we have described the dynamics of exocytosis in the acini of rodent salivary glands (SGs), focusing on the step that follows the fusion of large secretory vesicles with the apical PM and the opening of the fusion pore.Citation4 Specifically, we used intravital microscopy, an imaging approach based on light microscopy, which provides the necessary spatial and temporal resolution to study the dynamics of subcellular organelles in live animals.Citation15-Citation17 The architecture of the acini and the site where exocytosis occurs have been characterized extensively. Acini are formed by 9‑10 polarized acinar cells, which are tightly packed (). The apical domains of the PM of two adjacent cells form narrow canaliculi with a diameter of 0.2‑0.3 µm and a length of 10‑15 µm. Each cell shares 2‑3 canaliculi with adjacent cells, and they are all interconnected, ultimately merging into a larger duct at the base of the acinus, called the intercalated duct (, andCitation4). A single acinar cell contains 250‑300 secretory vesicles (termed secretory granules (SCGs)), which have a diameter of 1‑1.5 µm.
After fusion with the PM, the SCGs gradually collapse within 40‑60 s. The kinetics is very slow, especially when compared with other exocytic vesicles, such as synaptic or neuroendocrine vesicles, which exocytose in few milliseconds.Citation18,Citation19 Some studies suggest that the slow kinetics of exocytosis may reflect the nature of large cargo molecules, which are tightly packed and require longer time to disassemble once exposed to the extracellular space.Citation20,Citation21 However, since in SGs cargo molecules do not form dense aggregates,Citation11,Citation22 we favor another possibility based on the difference between the biophysical properties of the fusing membranes. Specifically, when two membranous compartments fuse there is a flow of membranes toward the area with the lowest membrane tension.Citation23,Citation24 Based on geometrical consideration, large SCGs should have a lower membrane tension than narrow canaliculi, and indeed we observed that after the opening of the fusion pore, membranes flow from the canaliculi into the SCGs (see Figure 2 in ref. Citation4). This suggests that the collapse of the SCGs into the PM may not be an energetically favorable process. We propose that the actomyosin complex is recruited onto the surface of the SCGs to form a contractile scaffold, which provides the energy to complete this step (). Although we do not know whether the force generated by the scaffold acts by pushing the membranes or by enlarging the fusion pore, we suggest that the actomyosin complex is required to reduce the size of the SCGs, thus increasing their membrane tension to a point in which the collapse becomes more energetically favorable. Notably, the disruption of the actin cytoskeleton results in a failure of the collapse of the SCGs, which grow in size forming large vacuoles, whereas the inhibition of the myosin motor activity results in an initial expansion of the SCGs followed by a gradual collapse, which is significantly delayed in a small subpopulation of vesicles.
Figure 2. Role of the actomyosin complex in the gradual collapse of the secretory granules. A. SCGs (blue) fuse with the APM (red), the fusion pore opens, and membranes flow from the APM into the SCGs (red arrows). The difference in membrane tension may not favor the flow of the membranes toward the APM that would lead to the gradual collapse of the SCGs. The contractile activity of the actomyosin complex (actin, green rods; myosin II, blue) that assembles around the SCGs may push the membranes (black arrows) and/or dilate further the fusion pore (gray arrows) to facilitate the gradual collapse. B. In the absence of a functional actin cytoskeleton the SCGs expand forming large vacuoles (2‑5 µm). Membranes flow into the large vacuoles, which acquire the properties of the APM. Due to the lower membrane tension of the large vacuoles, the remaining SCGs gradually collapse without the need of a functional actomyosin complex. C. In the absence of a functional myosin II, the acinar canaliculi significantly expand in size (1.8‑2.0 µm). The SCGs fuse with the APM and slightly expand in size (2‑2.5 µm). Under these conditions the difference in membrane tension may be more favorable to a actomyosin-independent gradual collapse.
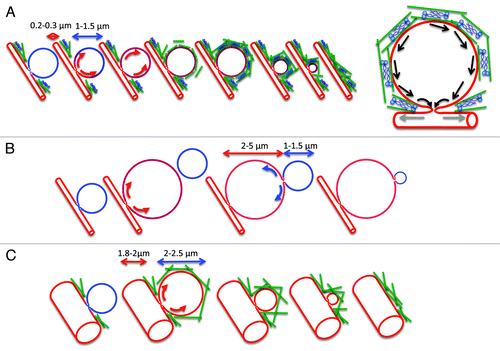
Two interesting observations further support this model. First, in the absence of a functional actin cytoskeleton the SCGs collapse with the large vacuoles. Under this condition, SCGs (diameter 1‑1.5 µm) have a higher membrane tension than the large vacuoles (4‑5 µm) and their collapse is more favorable (). Second, we noted that when the activity of the myosin II motor is impaired, the acinar canaliculi are significantly expanded (diameter 1.8‑2.0 µm). Under this condition, SCGs should have a higher membrane tension than the expanded canaliculi and the process could possibly be brought to completion without the need for a contractile activity ().
This model may apply to other systems as well. For example, the recruitment of the actomyosin scaffold around the SCGs have been observed in other exocrine glands such as the pancreas and the lacrimal glands, which share a similar geometry with the SGs, and in which exocytosis proceeds with a slow kinetics as well.Citation25,Citation26 Actin and myosins have been observed around cortical granules in Xenopus eggs, in Weibel-Palade bodies in endothelial cells, and in lamellar bodies in alveolar type II cells.Citation21,Citation25,Citation28 However, in these systems the role of the actomyosin complex may be related to the dense packing of cargo molecules rather than the differences in membrane tension.
In other exocytic systems, such as endocrine glands or pre-synaptic terminals, secretory vesicles are much smaller in size (diameter 50‑300 nm) and they fuse very rapidly with membrane domains that have much lower tensions. Interestingly, in these systems actin was not reported to coat the vesicles but rather to have a function at the PM in preventing premature fusion of the secretory vesicles or to direct them to specific exocytic sites.Citation3,Citation29,Citation30 Although these findings correlate well with the model presented, a more detailed characterization of the modality and kinetics of exocytosis in vivo has to be performed for these systems.
Acknowledgments
This research was supported by the Intramural Research Program of the NIH, National Institute of Dental and Craniofacial Research.
- Masedunskas A, Sramkova M, Parente L, Sales KU, Amornphimoltham P, Bugge TH, et al. Role for the acto-myosin complex in regulated exocytosis revealed by intravital microscopy. Proc Natl Acad Sci USA 2011; 108:13552 - 7; http://dx.doi.org/10.1073/pnas.1016778108; PMID: 21808006
References
- Burgoyne RD, Morgan A. Secretory granule exocytosis. Physiol Rev 2003; 83:581 - 632; PMID: 12663867
- De Matteis MA, Luini A. Exiting the Golgi complex. Nat Rev Mol Cell Biol 2008; 9:273 - 84; http://dx.doi.org/10.1038/nrm2378; PMID: 18354421
- Sokac AM, Bement WM. Kiss-and-coat and compartment mixing: coupling exocytosis to signal generation and local actin assembly. Mol Biol Cell 2006; 17:1495 - 502; http://dx.doi.org/10.1091/mbc.E05-10-0908; PMID: 16436510
- Masedunskas A, Sramkova M, Parente L, Sales KU, Amornphimoltham P, Bugge TH, et al. Role for the actomyosin complex in regulated exocytosis revealed by intravital microscopy. Proc Natl Acad Sci USA 2011; 108:13552 - 7; http://dx.doi.org/10.1073/pnas.1016778108; PMID: 21808006
- Rizzoli SO, Jahn R. Kiss-and-run, collapse and 'readily retrievable' vesicles. Traffic 2007; 8:1137 - 44; http://dx.doi.org/10.1111/j.1600-0854.2007.00614.x; PMID: 17645434
- Südhof TC. The synaptic vesicle cycle. Annu Rev Neurosci 2004; 27:509 - 47; http://dx.doi.org/10.1146/annurev.neuro.26.041002.131412; PMID: 15217342
- Behrendorff N, Dolai S, Hong W, Gaisano HY, Thorn P. Vesicle-Associated Membrane Protein 8 (VAMP8) is a SNARE (Soluble N-Ethylmaleimide-sensitive Factor Attachment Protein Receptor) selectively required for sequential granule-to-granule fusion. J Biol Chem 2011; 286:29627 - 34; http://dx.doi.org/10.1074/jbc.M111.265199; PMID: 21733851
- Kasai H, Kishimoto T, Nemoto T, Hatakeyama H, Liu TT, Takahashi N. Two-photon excitation imaging of exocytosis and endocytosis and determination of their spatial organization. Adv Drug Deliv Rev 2006; 58:850 - 77; http://dx.doi.org/10.1016/j.addr.2006.07.008; PMID: 16996640
- Zhang J, Castle D. Regulation of fusion pore closure and compound exocytosis in neuroendocrine PC12 cells by SCAMP1. Traffic 2011; 12:600 - 14; http://dx.doi.org/10.1111/j.1600-0854.2011.01170.x; PMID: 21272170
- Castle JC, Zhang C, Shah JK, Kulkarni AV, Kalsotra A, Cooper TA, et al. Expression of 24,426 human alternative splicing events and predicted cis regulation in 48 tissues and cell lines. Nat Genet 2008; 40:1416 - 25; http://dx.doi.org/10.1038/ng.264; PMID: 18978788
- Gorr SU, Venkatesh SG, Darling DS. Parotid secretory granules: crossroads of secretory pathways and protein storage. J Dent Res 2005; 84:500 - 9; http://dx.doi.org/10.1177/154405910508400604; PMID: 15914585
- Royce LS, Kibbey MC, Mertz P, Kleinman HK, Baum BJ. Human neoplastic submandibular intercalated duct cells express an acinar phenotype when cultured on a basement membrane matrix. Differentiation 1993; 52:247 - 55; http://dx.doi.org/10.1111/j.1432-0436.1993.tb00637.x; PMID: 7683292
- Yeh C, Mertz PM, Oliver C, Baum BJ, Kousvelari EE. Cellular characteristics of long-term cultured rat parotid acinar cells. In Vitro Cell Dev Biol 1991; 27A:707 - 12; http://dx.doi.org/10.1007/BF02633215; PMID: 1717429
- Proctor GB, Carpenter GH. Regulation of salivary gland function by autonomic nerves. Auton Neurosci 2007; 133:3 - 18; http://dx.doi.org/10.1016/j.autneu.2006.10.006; PMID: 17157080
- Masedunskas A, Weigert R. Intravital two-photon microscopy for studying the uptake and trafficking of fluorescently conjugated molecules in live rodents. Traffic 2008; 9:1801 - 10; http://dx.doi.org/10.1111/j.1600-0854.2008.00798.x; PMID: 18647170
- Sramkova M, Masedunskas A, Parente L, Molinolo A, Weigert R. Expression of plasmid DNA in the salivary gland epithelium: novel approaches to study dynamic cellular processes in live animals. Am J Physiol Cell Physiol 2009; 297:C1347 - 57; http://dx.doi.org/10.1152/ajpcell.00262.2009; PMID: 19794147
- Weigert R, Sramkova M, Parente L, Amornphimoltham P, Masedunskas A. Intravital microscopy: a novel tool to study cell biology in living animals. Histochem Cell Biol 2010; 133:481 - 91; http://dx.doi.org/10.1007/s00418-010-0692-z; PMID: 20372919
- Malacombe M, Bader MF, Gasman S. Exocytosis in neuroendocrine cells: new tasks for actin. Biochim Biophys Acta 2006; 1763:1175 - 83; http://dx.doi.org/10.1016/j.bbamcr.2006.09.004; PMID: 17034880
- Thorn P, Fogarty KE, Parker I. Zymogen granule exocytosis is characterized by long fusion pore openings and preservation of vesicle lipid identity. Proc Natl Acad Sci USA 2004; 101:6774 - 9; http://dx.doi.org/10.1073/pnas.0400336101; PMID: 15090649
- Dietl P, Liss B, Felder E, Miklavc P, Wirtz H. Lamellar body exocytosis by cell stretch or purinergic stimulation: possible physiological roles, messengers and mechanisms. Cell Physiol Biochem 2010; 25:1 - 12; http://dx.doi.org/10.1159/000272046; PMID: 20054140
- Nightingale TD, White IJ, Doyle EL, Turmaine M, Harrison-Lavoie KJ, Webb KF, et al. Actomyosin II contractility expels von Willebrand factor from Weibel-Palade bodies during exocytosis. J Cell Biol 2011; 194:613 - 29; http://dx.doi.org/10.1083/jcb.201011119; PMID: 21844207
- Venkatesh SG, Cowley DJ, Gorr SU. Differential aggregation properties of secretory proteins that are stored in exocrine secretory granules of the pancreas and parotid glands. Am J Physiol Cell Physiol 2004; 286:C365 - 71; http://dx.doi.org/10.1152/ajpcell.00338.2003; PMID: 14576088
- Chizmadzhev YA, Kumenko DA, Kuzmin PI, Chernomordik LV, Zimmerberg J, Cohen FS. Lipid flow through fusion pores connecting membranes of different tensions. Biophys J 1999; 76:2951 - 65; http://dx.doi.org/10.1016/S0006-3495(99)77450-3; PMID: 10354423
- Chizmadzhev YA, Kuzmin PI, Kumenko DA, Zimmerberg J, Cohen FS. Dynamics of fusion pores connecting membranes of different tensions. Biophys J 2000; 78:2241 - 56; http://dx.doi.org/10.1016/S0006-3495(00)76771-3; PMID: 10777723
- Jerdeva GV, Wu K, Yarber FA, Rhodes CJ, Kalman D, Schechter JE, et al. Actin and non-muscle myosin II facilitate apical exocytosis of tear proteins in rabbit lacrimal acinar epithelial cells. J Cell Sci 2005; 118:4797 - 812; http://dx.doi.org/10.1242/jcs.02573; PMID: 16219687
- Valentijn JA, Valentijn K, Pastore LM, Jamieson JD. Actin coating of secretory granules during regulated exocytosis correlates with the release of rab3D. Proc Natl Acad Sci USA 2000; 97:1091 - 5; http://dx.doi.org/10.1073/pnas.97.3.1091; PMID: 10655489
- Valentijn KM, Sadler JE, Valentijn JA, Voorberg J, Eikenboom J. Functional architecture of Weibel-Palade bodies. Blood 2011; 117:5033 - 43; http://dx.doi.org/10.1182/blood-2010-09-267492; PMID: 21266719
- Sokac AM, Co C, Taunton J, Bement W. Cdc42-dependent actin polymerization during compensatory endocytosis in Xenopus eggs. Nat Cell Biol 2003; 5:727 - 32; http://dx.doi.org/10.1038/ncb1025; PMID: 12872130
- Trifaró JM, Gasman S, Gutierrez LM. Cytoskeletal control of vesicle transport and exocytosis in chromaffin cells. Acta Physiol (Oxf) 2008; 192:165 - 72; http://dx.doi.org/10.1111/j.1748-1716.2007.01808.x; PMID: 18021329
- Eitzen G. Actin remodeling to facilitate membrane fusion. Biochim Biophys Acta 2003; 1641:175 - 81; http://dx.doi.org/10.1016/S0167-4889(03)00087-9; PMID: 12914958