Abstract
The self-organization of actin filaments is a topic that links cell biology with condensed matter physics. In vitro assays allow precise manipulation of component mechanical and chemical properties, needed for rigorous tests of theoretical models. We review recent work on in vitro motility assays that documented emergence of ordered actin filament microdomains powered by myosin motor proteins at high filament densities. Motor and filament surface density and mechanochemical cycle kinetics are additional parameters under current investigation. Individual filament collisions have been studied in order to elucidate the emergent population behavior. Apolar, weak interactions evidenced by local filament deformations during crossover events are attenuated at high motor densities. Theoretical analysis requires refinement of rigid rod filament models. In intact cells, accessory proteins modulate actin filament length, bundling or sliding and this gives rise to complex emergent structures and behaviors such as cell motility and chemotaxis. The development of generic, mechanical and biochemical frameworks with predictive power that link molecular properties with micro- and macroscopic phenomena seen in living cells requires dialogue between theoreticians and experimentalists.
Active actin gels utilize the energy of ATP hydrolysis to form locally ordered structures or microdomains. ATP hydrolysis is required both for actin filament polymerization dynamics and for force production by myosins, a superfamily of actin-binding, motor proteins.Citation1 In this mini-review we focus on myosin driven alignment and ordering of phalloidin-stabilized actin filaments in an in vitro motility assay.
Actin is a 42kDa molecular weight, globular, protein that is highly conserved across plant and animal kingdoms. In living cells, actin polymerises to form filamentous actin (known as F-actin) which is comprised of two helical protofilaments of globular actin (G-actin) subunits wound around each other, having a pseudo-repeat distance of 36nm comprising 14 monomers. Each G-actin subunit is oriented in the same axial direction, so the filament is polarized having a rapidly polymerising, “plus” end and a more slowly polymerising, “minus” end. The filament has mean diameter of ~8 nm, and the structure becomes visibly bent by thermal motions. Bending motions are coupled to twisting modesCitation2 and the thermal persistence length is around ~18 µm3, but there is limited extensibility.Citation3 A large set of actin binding proteins (ABPs) exists in living cells to regulate actin cytoskeletal organization.Citation4 Actin polymerisation requires ATP hydrolysis and G-actin monomer addition at the plus end of the filament drives extension of dynamic cellular structures such as filopodia and lamellipodia and thereby powers cell movements in chemotaxis, phagocytosis and neuronal pathfinding.Citation5 More stable, actin networks comprise the cytoskeleton which forms static cellular structures such as hair cell stereocilia,Citation6 stress fibersCitation7 and contractile ringsCitation8 which function in hearing, cell adhesion and cell division respectively.
The development of in vitro motility assays based on purified componentsCitation9 opened the way for quantitative analysis of cytoskeletal filament–motor interactions.Citation10 In these assays the actin filaments are stabilized by addition of phalloidin, a toxin derived from the mushroom, Amanita phalloides, which when covalently coupled to rhodamine allows visualization of individual filaments by fluorescence video-microscopy. Early work on microtubules, a different cytoskeletal filament structure comprised of the protein, tubulin, demonstrated that asters and other structures may form, in vitro, by the action of genetically engineered motor proteins with multiple heads.Citation11 Parallel development of other experimental systems clarified the physical principles. Rigid rods or polar disksCitation12 aligned on a horizontal surface when subjected to vertical motions. Such systems exhibit giant number fluctuations. The fluctuations are a consequence of long-range order based on short-range contacts.Citation13 More recently, we demonstratedCitation14 that upon crowding, actin filaments self-organize into mobile domains that translocate over a myosin coated surface. As filament density was systematically increased, over a physiological range, the surface pattern changed from isotropic to a nematic phase that displayed linear and angular order with a domain size on the order of 100 microns. More recently, another group reported stripes, spirals and more complex patterns under similar conditions.Citation15
The emergence of collective motion in large groups of self-propelled objects was initially analyzed for bird flocks.Citation16 The physical rules are scale independent as developed in more recent work,Citation12 and thus are relevant to systems as diverse as fish shoals, cell aggregates, swimming microorganisms and cytoskeletal networks. Impressively, coarse-grained theories have been shown to have broad applicability to in vitro assays, computer models and cellular processes.Citation17 The “generic” principles must be modulated by system particular properties that reflect functional specialization and must also be understood. For cytoskeletal gels, there must be hydrodynamic terms that describe filament-filament coupling via fluid flow. In addition, there must be elastic and viscous terms that describe the properties of the filaments and their interactions with motor proteins. Microscopic theories, that explain the creation of long-range order in terms of the short-range interactions, model filament linkages as rigid rods actuated by processive motors with a capture range dependent on motor size and elasticity.Citation18,Citation19 Inertial forces are negligible in the low Reynolds number regime and so filament velocity is determined solely by the balance of net force produced by stretch of attached motor linkages and viscous drag. The classical cross-bridge modelCitation20 explains how elastic deformation of the myosin motor and the rate of its attachment to and detachment from actin controls the force-velocity relationship of actin and myosin filament sliding during muscle contraction.
In simulations of in vitro motility experiments performed at high actin filament density the excluded volume effects due to filament crowding were accounted for by a repulsive force term that is prohibitive for crossover events in 2D models. At high bulk actin concentration, surface motor density was predicted to favor isotropic to nematic transitions by increasing the attachment of filaments to the surface and, second, by increasing the repulsive force during individual actin filament collision eventsCitation19 We have begun to investigate these theoretical predictions (). Simulations based on these models have been successful in predicting qualitatively the transitions from isotropic to ordered nematics to wave or spiral patterns as a function of filament density.Citation15 The assumptions of rigid filaments and processive motors are accurate for microtubule-kinesin, but not actin-myosin II interactions. In contrast to F-actin, microtubules are hollow tubes with a diameter of 20 five nanometers and, consequently, are more rigid with a persistence length of a few millimeters.Citation21 More detailed scenarios that bear closer resemblance to the actomyosin system have been analyzed.Citation18 Microdomain formation in 3D by elastic rods powered by molecular motors with non-processive duty cycles expected for skeletal muscle myosin (myosin II) was demonstrated by simulations.
Figure 1. A. Isotropic to nematic phase transition is predicted to be favored by either increased filament or motor surface density. (B). Collision between two filaments at incident collision angle, I, moving in directions indicated by arrowheads can result in either crossover with emergent angle E > 0 or alignment (E = 0). C. Crossover vs. alignment data as function of angle I at two different motor densities. Parallel (closed symbols) and anti-parallel (open symbols) filament collisions are compared. The emergent angle E deviates from I with a bias toward alignment of 7.3 ± 2.9° at low motor density over the range I = 20–70°. The bias is reduced (3.1 ± 3.8) at high motor density. The probability of alignment increases from 10% to 34%.
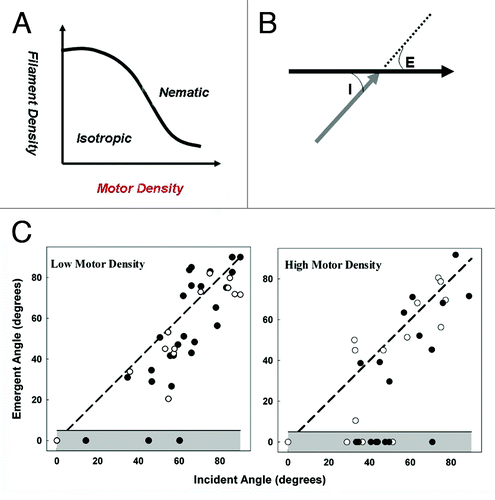
In published work,Citation14 we derived an angle-independent measure, the Kuiper statistic, of population orientation. Other quantitative measures have been used for other systems. Utilizing the Kuiper statistic, we demonstrated that the appearance of aligned domains depends strongly on both filament density and length. The mobile domains had diverse patterns and upon addition of ATP they took a few minutes to form. We also documented that orientation degrades upon depletion of ATP. The approach to steady-state was accompanied by filament fragmentation; perhaps due to filament collisions or breakage by myosin-generated forces. Once formed, the domains re-organized on micrometer length and second time scales, relevant for biological phenomena such as lamellipodial extension in mammalian cells.
In more recent work, we have studied single, filament-filament, interactions in absence of crowding to identify the determinants of local alignment. When two or more filaments collide, they either align or crossover (). The probability of alignment depends upon the angle of contact and motor surface density (). Our observations reveal weak interactions between the myosin propelled filaments as they align or crossover at low motor density. Alignment usually involves some bending of the filaments from their long axis (). Bending also occurs during crossovers, but tends to be localized to the intersection site, forming a kink in the moving filament (). The kink could be coupled to localized twist imparted to the filament due to inter-subunit interactions. Interestingly, the nature of the interaction is insensitive to filament polarity; since the obtained angular deviation is not significantly different for parallel vs. anti-parallel filament interactions. Failed alignment events were also observed (). High contact angle collisions frequently cause filaments to stall and buckle. The buckling provides an estimate of the filament tolerance to severing.Citation22 In such cases the filament transiently aligns but the leading, aligned segment recoils to partially restore the pre-collision filament shape and orientation, at high motor densities, filaments become more firmly attached to the surface and entrained to the direction of travel, presumably because the leading end comes within range of a motor before significant angular deviation from the filament axis. At low actin filament densities sliding speed is unaffected by motor density (except at extremely low valuesCitation23); whereas, at high actin filament densities, filament sliding velocity increases slightly with motor density. This increase is expected since the resultant vector force of the attached motors will be larger due to the improved alignment of the axis of travel with the filament long axis. At high motor densities, the bias of the angular deviation during crossovers due to filament interactions seems to be suppressed (). We expect that the deformations we observe during single collision events would be modulated in living cells by ABPs. For example, cofilins and gelsolins control the rate of filament polmerisation, severins cause filament fragmentation, ARP2/3 causes filament branching while fascins, fimbrins and alph-actinin bundle and align filaments.Citation24 These proteins may therefore act as switches to order or fluidize the actin cytoskeleton within cells.Citation22,Citation25 In order to fully capture the range and effect of these factors, more sophisticated treatments of actin filaments than the homogenous elastic rod model used thus far may be required.
Figure 2. Collision induced deformations. A. i. Two filaments prior to collision. ii. 0.1 sec post-collision. ii. Align. The alignment zone has brighter intensity that that of the single filaments. B. The vertical filament kinked at the intersection with the orthogonal filament, with straight segments on either side. The kink remained localized at the intersection while the filament traveled through. The vertical filament regained its straight shape, once crossover was complete. C. i. Two filaments 0.1 sec prior to collision. ii.The leading end of the vertically moving filament buckles to align with the horizontal filament, while its rear end deforms due to motor forces that push it against the horizontal filament. iii. The deformation builds a restoring force that breaks the transient alignment and recoils the filament toward its un-deformed shape.
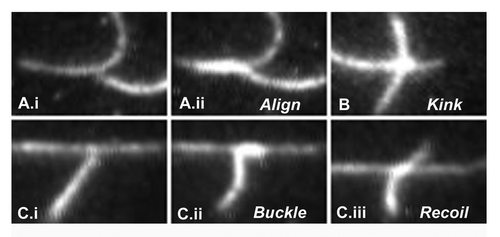
Much remains to be done to fully exploit the power of in vitro assays for understanding these phenomena. For instance, systematic study of the effect of ATP concentration, myosin motor density, actin filament length and crowding are needed to map the phase transitions. Also, increasing use needs to be made of multi-component mixtures to catalog the effect of particular ABPs.Citation26 High throughput, computerized analysis of single collision events will require development of more sophisticated tracking algorithms that utilize both static and dynamic cues to segment and distinguish the paths of interacting filaments.Citation14
Theoretical work has been important in revealing the general applicability of the underlying physics. Increasingly precise measurements of the biochemically defined mixtures utilized in in-vitro assays now offer the prospect for theoretical description and computational modeling of the microscopic detail underlying the collective motion of elastic rods and molecular motor generated forces. In turn, such effort might also help define the scope and value of generic theories for the emergence of collective motion in far from equilibrium systems.
Acknowledgment
We thank Dr S. Ramaswamy for comments on the manuscript.
References
- Goodson HV, Dawson SC. Multiplying myosins. Proc Natl Acad Sci USA 2006; 103:3498 - 9; http://dx.doi.org/10.1073/pnas.0600045103; PMID: 16537440
- Claessens MM, Semmrich C, Ramos L, Bausch AR. Helical twist controls the thickness of F-actin bundles. Proc Natl Acad Sci USA 2008; 105: 8819-22; Claessens MM, Bathe M, Frey E, and Bausch AR. Actin-binding proteins sensitively mediate F-actin bundle stiffness. Nat Mater 2006; 5:748 - 53; http://dx.doi.org/10.1038/nmat1718; PMID: 16921360
- Kojima H, Ishijima A, Yanagida T. Direct measurement of stiffness of single actin filaments with and without tropomyosin in in vitro nanomanipulation. Proc Natl Acad Sci USA 1994; 91:12962 - 6; http://dx.doi.org/10.1073/pnas.91.26.12962; PMID: 7809155
- Pollard TD. The cytoskeleton, cellular motility and the reductionist agenda. Nature 2003; 422:741 - 5; http://dx.doi.org/10.1038/nature01598; PMID: 12700767
- Pollard TD, Borisy GG. Cellular motility driven by assembly and disassembly of actin filaments. Cell 2003; 112:453 - 65; http://dx.doi.org/10.1016/S0092-8674(03)00120-X; PMID: 12600310
- Martin P, Hudspeth AJ, Julicher F. Comparison of a hair bundle's spontaneous oscillations with its response to mechanical stimulation reveals the underlying active process. Proc Natl Acad Sci USA 2001; 98:14380 - 5; http://dx.doi.org/10.1073/pnas.251530598; PMID: 11724945
- Pellegrin S, Mellor H. Actin stress fibres. J Cell Sci 2007; 120:3491 - 9; http://dx.doi.org/10.1242/jcs.018473; PMID: 17928305
- Salbreux G, Prost J, Joanny JF. Hydrodynamics of cellular cortical flows and the formation of contractile rings. Phys Rev Lett 2009; 103:058102; http://dx.doi.org/10.1103/PhysRevLett.103.058102; PMID: 19792537
- Kron SJ, Spudich JA. Fluorescent actin filaments move on myosin fixed to a glass surface. Proc Natl Acad Sci USA 1986; 83:6272 - 6; http://dx.doi.org/10.1073/pnas.83.17.6272; PMID: 3462694
- Vikhorev PG, Vikhoreva NN, Sundberg M, Balaz M, Albet-Torres N, Bunk R, et al. Diffusion dynamics of motor-driven transport: gradient production and self-organization of surfaces. Langmuir 2008; 24:13509 - 17; http://dx.doi.org/10.1021/la8016112; PMID: 18989944
- Surrey T, Nedelec F, Leibler S, Karsenti E. 2001 Physical properties determining self-organization of motors and microtubules. Science 2001; 292:1167 - 71; http://dx.doi.org/10.1126/science.1059758; PMID: 11349149
- Deseigne J, Dauchot O, Chaté H. Collective Motion of Vibrated Polar Disks. Phys Rev Lett 2010; 105:098001; http://dx.doi.org/10.1103/PhysRevLett.105.098001; PMID: 20868196
- Narayan V, Ramaswamy S, Menon N. Long-lived giant number fluctuations in a swarming granular nematic. Science 2007; 317:105 - 8; http://dx.doi.org/10.1126/science.1140414; PMID: 17615353
- Butt T, Mufti T, Humayun A, Rosenthal PB, Khan S, Khan S, et al. Myosin motors drive long range alignment of actin filaments. J Biol Chem 2010; 285:4964 - 74; http://dx.doi.org/10.1074/jbc.M109.044792; PMID: 19940124
- Schaller V, Weber C, Semmrich C, Frey E, Bausch AR. Polar patterns of driven filaments. Nature 2010; 467:73 - 7; http://dx.doi.org/10.1038/nature09312; PMID: 20811454
- Vicsek T, Czirók A, Ben-Jacob E, Cohen II, Shochet O. Novel type of phase transition in a system of self-driven particles. Phys Rev Lett 1995; 75:1226 - 9; http://dx.doi.org/10.1103/PhysRevLett.75.1226; PMID: 10060237
- Ramaswamy S. The Mechanics and Statistics of Active Matter. Annu Review Condensed Matter Physics 2010; 1:323 - 5; http://dx.doi.org/10.1146/annurev-conmatphys-070909-104101
- Liverpool TB, Marchetti MC. Instabilities of isotropic solutions of active polar filaments. Phys Rev Lett 2003; 90:138102; http://dx.doi.org/10.1103/PhysRevLett.90.138102; PMID: 12689327
- Kraikivski P, Lipowsky R, Kierfeld J. Enhanced ordering of interacting filaments by molecular motors. Phys Rev Lett 2006; 96:258103; http://dx.doi.org/10.1103/PhysRevLett.96.258103; PMID: 16907349
- Huxley AF. Muscle structure and theories of contraction. Prog Biophys Biophys Chem 1957; 7:255 - 318; PMID: 13485191
- Gittes F, Mickey B, Nettleton J, Howard J. Flexural Rigidity Of Microtubules and Actin-Filaments Measured From Thermal Fluctuations In Shape. J Cell Biol 1993; 120:923 - 34; http://dx.doi.org/10.1083/jcb.120.4.923; PMID: 8432732
- Kikuchi N, Ehrlicher A, Koch D, Käs JA, Ramaswamy S, Rao M. Buckling, stiffening, and negative dissipation in the dynamics of a biopolymer in an active medium. Proc Natl Acad Sci USA 2009; 106:19776 - 9; PMID: 19901332
- Uyeda TQP, Warrick HM, Kron SJ, Spudich JA. Quantized Velocities at Low Myosin Densities in an In Vitro Motility Assay. Nature 1991; 352:307 - 11; http://dx.doi.org/10.1038/352307a0; PMID: 1852205
- Bresnick AR. Molecular mechanisms of non-muscle myosin-II regulation. Curr Opin Cell Biol 1999; 11:26 - 33; http://dx.doi.org/10.1016/S0955-0674(99)80004-0; PMID: 10047526
- Kasza KE, Rowat AC, Liu J, Angelini TE, Brangwynne CP, Koenderink GH, et al. The cell as a material. Curr Opin Cell Biol 2007; 19:101 - 7; http://dx.doi.org/10.1016/j.ceb.2006.12.002; PMID: 17174543
- Köhler S, Schaller V, Bausch AR. Structure formation in active networks. Nat Mater 2011; 10:462 - 8; http://dx.doi.org/10.1038/nmat3009; PMID: 21516093