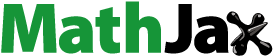
Abstract
Ears boost their sensitivity by means of active, force-generating processes to augment the minute vibrations induced by soft sounds. These processes can alter auditory frequency–tuning in a level-dependent way. In the antennal hearing organ of Drosophila, for example, the active process shifts the best-frequency (BF) of the antennal sound receiver when the sound intensity is varied, tuning the receiver to conspecific songs. Here we show that this level-dependent tuning can be reproduced by an active transduction model as proposed for vertebrate hair cells and the Drosophila ear. We further show that the direction of the frequency shift depends on the system to which the molecular modules for auditory transduction connect: If this system is mass-less such as the sensory hair bundles of bullfrog saccular hair cells, the BF of the displacement response will increase as the sound intensity declines. Conversely, BF will decrease with declining intensity if the transduction modules couple to inertial systems such as the fly’s antennal sound receiver or cupulae in the fish lateral line.
Hearing in Drosophila relies on Johnston's organ, a chordotonal stretch-receptor organ in the second segment of the fly's antenna.Citation1–Citation4 Spanning across an antennal joint, the mechanosensory cells comprised by this organ directly connect to the third, distal antennal segment, which, together with its lateral arista, vibrates in response to acoustic stimuli and serves the reception of sound.Citation5,Citation6 By measuring sound-induced vibrations of this receiver, it has been shown that its frequency-tuning characteristically alters with the intensity of sound: When the sound intensity is high (particle velocity ≥ ca. 1 mm/s), the BF of the receiver is ca. 800 Hz.Citation6,Citation7 When intensity is reduced, however, BF shifts down to lower frequencies with a rate of ca. 11 Hz/dB, assuming values of ca. 150–200 Hz in the absence of sound.Citation6,Citation7 This latter BF well corresponds to the dominant frequency of the fly's courtship songs, suggesting that the fly tunes its antennal sound receiver to conspecific songs.Citation6,Citation7
The mechanical tuning of the fly's antennal receiver reportedly linearizes post mortem, leading to a level-independent BF of ca. 800 Hz immediately after the animal's death.Citation7 CO2 exposure leads to an equivalent, though reversible linearization,Citation7 indicating that the nonlinear tuning of the fly's antennal receiver depends on metabolic processes inside the ear. This physiological vulnerability of the receiver's mechanical nonlinearity, along with self-sustained receiver oscillations,Citation7–Citation9 gave rise to the idea that vibrations of the fly's antennal receiver are nonlinearly enhanced by an active, force-generating process as known from vertebrate ears.Citation8–Citation10 Subsequent studies confirmed that the mechanosensory cells of Johnston's organ actively pump energy into the receiver's vibrations,Citation11,Citation12 documenting the existence of an active process for the fly's auditory system and demonstrating that the auditory mechanosensory cells of Drosophila, like vertebrate hair cells,Citation13,Citation14 expend energy to actively augment the mechanical input of the ear.
A candidate source of active amplification in hearing are the molecular modules for auditory transduction, which, in flies as in vertebrates, seem to consist of force-gated ion channels that occur in series with adaptation motors and gating springs.Citation15–Citation17 When supplemented with feedback between channels and motors, these molecular modules explain the hair bundle motility of vertebrate hair cellsCitation18,Citation19 and, as shown recently,Citation12 also explain the active behavior of the Drosophila ear: coupling hair cell-like active transduction modules to a harmonic oscillator proofed sufficient for reproducing the mechanical properties of the fly's antennal receiver, including its response to force steps, its free fluctuations, its linear response function, its activity and nonlinearity, and properties of mechanically evoked electrical responses in the afferent nerve.Citation12 Whether this simple transducer-based model also reproduces those level-dependent shifts of the receiver's BF that initially betrayed the active process in the fly's auditory systemCitation7 and whether the respective model for vertebrate hair cellsCitation17 also shows such behavior, however, has not been determined yet.
To test whether transducer-based models of active amplification predict level-dependent alterations in tuning for the sensory hair bundles of vertebrate hair cells and the fly's antennal receiver, we explored how the response functions defined by these models alter when the stimulus amplitude is changed. Model and parameter values describing the active mechanics of the fly's antennal receiver and active hair bundle mechanics were taken from references Citation18 and Citation12, respectively. To determine response functions , we subjected the differential equations describing the models to sinusoidal stimuli of varying amplitudes and frequencies. Responses were calculated as the ratio between the Fourier transforms of the resulting displacement X and the stimulus force,
, where f is the stimulus frequency and the tilde denotes the Fourier transform:
Responses obtained for equal forcing amplitudes and distinct frequencies were used to determine level-specific response functions.
Consistent with experimental observations,Citation7 numerically simulated response functions predict a tuning for the Drosophila sound receiver that depends on the amplitude of forcing, i.e., the stimulus level (): according to the model, the tuning of this receiver is only independent of the forcing amplitude if forcing is either very weak (Fext < ca. 1 pN) or very strong (Fext > ca. 100 pN, linear regimes). For intermediate forcing amplitudes, however, the BF nonlinearly shifts from ca. 180 to 910 Hz as the stimulus increases with a maximum slope that slightly exceeds the average slope that has been experimentally observed [20 Hz/dB or 0.06 octaves/dB () vs. ca. 11 Hz/dB (ref. Citation7)]. The low-intensity linear regime predicted by the model has been experimentally documented for response functions of the fly's receiver,Citation12 and also the high-intensity linear regime has been observed in the receiver's response to single tones. The very transducer-based model that explains active amplification in the fly's auditory systemCitation12 thus reproduces the level-dependent tuning of the Drosophila sound receiver.
Numerically simulated response functions also predict a level-dependent tuning for the sensory hair bundles of bullfrog saccular hair cells, yet in their case the direction of the frequency shift is inversed (): at intermediate forcing amplitudes, the BF of the hair bundle shifts down with increasing forcing amplitude, moving from ca. 10 Hz at weak forcing (Fext < ca. 2 pN) to 0 Hz (Fext > ca. 200 pN) at high forcing with a maximum slope of −0.37 Hz/dB (−0.08 octaves/dB).
As judged from the models,Citation12,Citation18 the gating of transduction channels and the activity of adaptation motors nonlinearly shifts the BF of both the sensory hair bundles of bullfrog hair cells and the antennal sound receiver of the fly. The models further predict that the direction of this shift depends on the mechanical properties of the stimulus receiver to which the transduction modules connect:
In the fly, the transduction modules couple to the antennal sound receiver that, by itself, can be described as a simple harmonic oscillator with stiffness, friction and mass. The spring constant of this harmonic oscillator and the stiffness of the gating springs together determine the overall stiffness of the system (see Annex). The effective stiffness contributed by the gating springs depends on the open probability of the transduction channels (see Annex), which can assume values between 0 and 1. For intermediate open probabilities, the effective stiffness contributed by the gating springs is reduced by the gating of transduction channels, an effect that is known as nonlinear gating compliance.Citation15,Citation16 For large or small open probabilities, the effective stiffness contributed by the gating springs is independent of transducer gating because no gating events occur. If stimulus amplitudes are large, the channels are all mostly open or closed so that the nonlinear gating compliance can be neglected. If stimulus amplitudes are small, in turn, the channel open probability is mostly around 0.5 and the nonlinear gating compliance softens the system, explaining why the BF of the fly's antennal sound receiver shifts down to lower frequencies as the stimulus intensity drops.
In vertebrate hair cells, the transduction modules couple to inter-linked stereocilia, which, by themselves, can be described as a spring-dashpot system with stiffness, friction, but no mass: at low frequencies, elastic and viscous forces dominate hair bundle mechanics, whereas inertial effects can be neglected. The corresponding BF of such a spring-dashpot is zero. If the BF of the corresponding response function for low stimulus amplitudes is non-zero, the BF of the system must decline towards zero when the stimulus amplitude is increased (see Annex). Note that this is only true for the displacement response functions, , which if the transduction modules connect to a spring-dashpot system, velocity response functions:
display a BF that increases with intensity. Using the parameter values reported for bullfrog sensory hair bundles, we find that the BF of the velocity response function shifts up from about 10 Hz to infinity with a maximum slope of 2.8 Hz/dB. In the case of the fly's antennal receiver, however, simulated displacement and velocity response functions display very similar BF-shifts: the BF of the velocity response functions shifting from ca. 190 to 930 Hz with increasing intensity with a maximum slope of 21 Hz/dB.
We have shown that a simple transduction model as proposed to explain the active properties of the fly's antennal sound receiver also explains the level-dependent tuning of this receiver. According to our analysis, these shifts result from changes of the effective stiffness of the system, which is minimal for low stimulus amplitudes due to the gating of transduction channels. Because this gating takes only place over a finite range of stimulus amplitudes, BF nonlinearly shifts only within a certain range of intensities (nonlinear regime, ) and assumes constant values if intensity is either very high or very low (linear regimes, ). Our analysis also predicts that equivalent transducer modules nonlinearly shift the BF of sensory hair bundles (), and, generally that the sign of the shift depends on the mechanical properties of the stimulus receiver to which the transduction modules connect: In both systems, hair cells and fly ears, the passive mechanical properties of the stimulus receiver dictate the BF of the system in the high intensity linear regime, whereas in the low intensity regime the BF is determined by the interplay between passive receiver mechanics and the active dynamical properties of the transduction modules. If the BFs of the high and low intensity linear regimes are different, level-dependent BF-shifts occur.
Our analysis shows that level-dependent BF-shifts in hearing can directly result from transducer gating. If the transduction modules couple to inertial systems, as is the case in the Drosophila hearing organ, the BF shifts down as intensity declines. In effect, cupulae, which sit on top of sensory hair bundles in hair cells of the fish lateral line and can be considered as inertial systems, also display such BF-shifts.Citation20 Shifts in the opposite direction have been documented for the antennal sound receivers of male mosquitoes,Citation21 which evidently are inertial systems, indicating that the simple transducer-based model that explains active amplification in the antennal ear of Drosophila may not suffice to explain the macroscopic phenomenology of active amplification in the antennal ears of mosquitoes.
Annex
Model equations describing active amplification in hair cellsCitation18 and fly earsCitation12 allow to analytically calculate linear response functions, , which determine the displacement response X(t) to small external forces Fext (t) in the low-intensity linear regimes:Citation12,Citation18
(1)
(1) The linear response function of the fly's receiver,
, can be written in the form of the response function of a harmonic oscillator with frequency-dependent stiffness kF (f) and friction γF(f):
(2)
(2) with
(3)
(3)
(4)
(4)
Here, KGS = KGS(1 − DP′o,s), is the effective gating-spring stiffness around the stationary point, Po,s the opening probability at the stationary point, and
is the derivative of the open probability at the stationary point XS. For explanations of the parameters, see ref. Citation12.
Likewise, the linear response function of the hair bundle, , can be written as:
(5)
(5) with
(6)
(6)
(7)
(7) Here, we assume that the feedback between channels and motors is fast (time constant τ → 0).Citation18 For explanations of the parameters, see ref. Citation18.
The linear response functions of both systems are similar with two main exceptions: First, the inertial term in the response function for the fly's receiver (Equationequation 2(2)
(2) ) is absent from that for the hair bundle (Equationequation 5
(5)
(5) ). Second, the factor 2 in the expressions for the frequency-dependent stiffness (Equationequation 3
(3)
(3) ) and friction (Equationequation 4
(4)
(4) ) of the fly's receiver is absent in the corresponding expressions for the hair bundle (Equationequations 6
(6)
(6) and Equation7
(7)
(7) ). This factor accounts for the symmetric arrangement of transduction modules, which is an anatomical peculiarity of the Drosophila ear.Citation12
For large forcing amplitudes, the channel open probability in both systems is close to zero or one for most of the time, which means that the response function of both systems to very large forces can be approximated by choosing P′o,s = 0 in the equations describing the linear response functions to very small forces (Equationequations 2(2)
(2) and Equation5
(5)
(5) ). Note that the resulting response functions are equivalent to those of the passive systems (Fmax = 0 or S = 0), KGS → KGS and Fmax P′o,S S → 0.Citation12,Citation18 A simple calculation shows that the derivative of the amplitude of the hair-bundle's response function for high intensities is always negative, explaining why the corresponding BF is zero. The sensitivity of the system at this point is:
where KSP is the stiffness of the stereociliar pivot. Hence, although the system displays frequency-dependent stiffness and friction terms, the spring-dashpot system with stiffness KSP dominates at low frequencies, and the BF as well as the highest sensitivity of the whole hair-bundle are those of the spring-dashpot system. Therefore, if the hair-bundle's BF for small stimuli is non-zero, BF must decrease as the stimulus amplitude increases. Because the derivative of the amplitude of the hair bundle's velocity response function is always positive, the corresponding BF is infinitely high for very large stimulus forces.
In the case of the fly's sound receiver, the BF is non-zero and finite for both very weak and very strong forcing. In principle, it is possible to find parameter values that allow for BF-shifts in the opposite direction than experimentally observed: a decreasing BF with increasing stimulus level. However, BF will always increase with the stimulus level if (i.e., if the motor friction dominates the stiffness terms): in this regime, the dominant friction is given by λ alone and the dominant stiffness increases with intensity from KSP + 2KGS (1 − DP′o,S) to KSP + 2KGS, thus raising the system's BF. Given the parameter values reported for the fly's antennal receiver,Citation12 the friction term becomes larger than the stiffness term
for low amplitudes and at f > 42 Hz for high amplitudes, where KGS + FmaxP′o,S S → KGS.
Figures and Tables
Figure 1 Numerically simulated response functions of the Drosophila sound receiver (A) and the sensory hair bundle of bullfrog saccular hair cells (B) using models and parameter values from references 12 (‘fly 6’) and 18, respectively. Response functions are shown for different forcing amplitudes (for stimulus forces, see lower panel). Blue lines and circles correspond to the strongest stimuli, red lines and circles to the weakest stimuli. Upper, amplitude, which provides a measure of sensitivity. Middle: phase. Lower, BF as a function of the stimulus force. Both systems display nonlinear regimes in which the BF continuously shifts with the amplitude of forcing, yet their BFs shift in different directions.
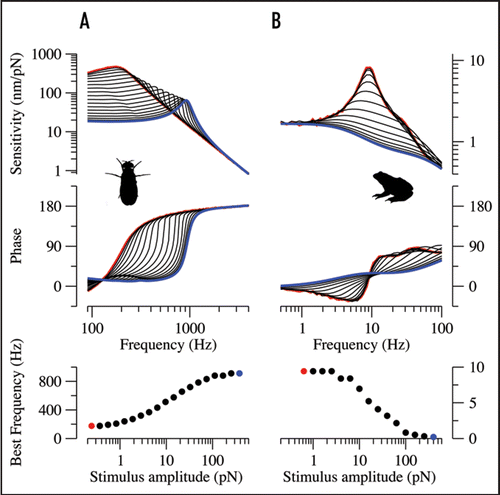
Acknowledgements
This work was supported by a research fellowship from the Volkswagen Foundation (to Björn Nadrowski), grants from the Volkswagen Foundation and the German BMBF Bernstein Network for Computational Neuroscience (to Martin C. Göfert), and a start-up grant from the University of Göttingen.
Addendum to:
References
- Eberl DF. Feeling the vibes: chordotonal mechanisms in insect hearing. Curr Opin Neurobiol 1999; 9:389 - 393
- Kernan MJ. Mechanotransduction and auditory transduction in Drosophila. Pflügers Arch 2007; 454:703 - 720
- Albert JT, Nadrowksi B, Göpfert MC. Drosophila mechanotransduction-linking proteins and functions. Fly 2007; 1:238 - 241
- Kamikouchi A, Shimada T, Ito K. Comprehensive classification of the auditory sensory projections in the brain of the fruit fly Drosophila melanogaster. J Comp Neurol 2006; 499:317 - 356
- Göpfert MC, Robert D. Biomechanics. Turning the key on Drosophila audition. Nature 2001; 411:908
- Göpfert MC, Robert D. The mechanical basis of Drosophila audition. J Exp Biol 2002; 205:1199 - 1208
- Göpfert MC, Robert D. Motion generation by Drosophila mechanosensory neurons. Proc Natl Acad Sci USA 2003; 100:5514 - 5519
- Stoop R, Kern A, Göpfert MC, Smirnov DA, Dikanev TV, Bezrucko BP. A generalization of the van-der-Pol oscillator underlies active signal amplification in Drosophila hearing. Eur Biophys J 2006; 35:511 - 516
- Göpfert MC, Albert JT, Nadrowski B, Kamikouchi A. Specification of auditory sensitivity by Drosophila TRP channels. Nat Neurosci 2006; 9:999 - 1000
- Hudspeth AJ. Making an effort to listen: mechanical amplification in the ear. Neuron 2008; 59:530 - 545
- Göpfert MC, Humphris AD, Albert JT, Robert D, Hendrich O. Power gain exhibited by motile mechanosensory neurons in Drosophila ears. Proc Natl Acad Sci USA 2005; 102:325 - 330
- Nadrowski B, Albert JT, Göpfert MC. Transducer-based force generation explains active process in Drosophila hearing. Curr Biol 2008; 18:1365 - 1372
- Martin P, Hudspeth AJ, Jülicher F. Comparison of a hair bundle's spontaneous oscillations with its response to mechanical stimulation reveals the underlying active process. Proc Natl Acad Sci USA 2001; 98:14380 - 14385
- LeMasurier M, Gillespie PG. Hair-cell mechanotransduction and cochlear amplification. Neuron 2005; 48:403 - 415
- Howard J, Hudspeth AJ. Compliance of the hair bundle associated with gating of mechanoelectrical transduction channels in the bullfrog's saccular hair cell. Neuron 1988; 1:189 - 199
- Hudspeth AJ, Choe Y, Mehta AD, Martin P. Putting ion channels to work: Mechanoelectrical transduction, adaptation and amplification by hair cells. Proc Natl Acad Sci USA 2000; 97:11765 - 11772
- Albert JT, Nadrowski B, Göpfert MC. Mechanical signatures of transducer gating in the Drosophila ear. Curr Biol 2007; 17:1000 - 1006
- Nadrowski B, Martin P, Jülicher F. Active hair-bundle motility harnesses noise to operate near an optimum of mechanosensitivity. Proc Natl Acad Sci USA 2004; 17:101 12195 - 12200
- Tinevez JY, Jülicher F, Martin P. Unifying the various incarnations of active hair-bundle motility by the vertebrate hair cell. Biophys J 2007; 93:4053 - 4067
- van Netten SM. Hair cell mechano-transduction: its influence on the gross mechanical characteristics of a hair cell sense organ. Biophys Chem 1997; 68:43 - 52
- Göpfert MC, Robert D. Active auditory mechanics in mosquitoes. Proc Biol Sci 2001; 268:333 - 339