Abstract
Mammalian spermatozoa are highly polarized cells composed of two morphological and functional compartments, each optimized for a special task. Although the compartmentalization into head and tail may as such represent the anatomical basis to avoid random diffusion of their special sets of signaling proteins and lipids, recent findings demonstrate the presence of lipid raft-derived membrane platforms and specific scaffolding proteins, thus indicating that additional smaller sub-domains exist in the two compartments of male germ cells. The aim of this review is to summarize new insights into the principles of sub-compartmentalization in mammalian spermatozoa. Special emphasis is placed on recent observations indicating that an “acrosomal synapse” is formed by lipid raft-derived membrane micro-environments and multidomain scaffolding proteins. Both mechanisms appear to be responsible for ensuring the attachment of the huge acrosomal vesicle to the overlaying plasma membrane, as well as for preventing an accidental spontaneous loss of the single acrosome.
Introduction
A mammalian spermatozoon is characterized by two functional parts, both perfectly adapted to perform a number of necessary functions before the sperm can carry out its main challenge, the fertilization of an egg (). The flagellum is the motor module which helps to drive the ejaculated spermatozoon to the site of fertilization in the oviduct. After encountering the egg, a series of functionally coupled events is initiated, which comprises reactions mainly mediated by the sperm head, like recognition and binding of the extracellular glycoprotein coat surrounding the egg, sperm-oocyte fusion and the fusion of their nuclei.Citation1 However, freshly ejaculated sperm are not immediately competent to carry out their main task but have to gain this potency during a series of spatial reorganization and biochemical modification processes, collectively termed capacitation.Citation2,Citation3 One of these “maturating” changes is reflected by an increase in the amplitude of the flagellar beating and slow asymmetrical tail bending, a whiplash moving pattern called hyperactivation.Citation4 The resulting forceful beating of the tail drives sperm through the oviductal mucus and generates the required thrust to successfully penetrate the two protecting barriers of the oocyte, the cumulus cells and the Zona pellucida glycoprotein matrix (reviewed in ref. Citation5). However, an enzymatic degradation of the extracellular matrix of glycoproteins achieved by hydrolyzing enzymes stored in the acrosome is also indispensable (reviewed in ref. Citation6).
The secretion of these hydrolyzing enzymes is elicited by a special form of Ca2+-regulated exocytosis, called acrosome reaction, which also requires capacitation-dependent maturation steps. For instance, an efflux of cholesterol from the sperm plasma membrane (reviewed in ref. Citation7) and the tethering of the huge acrosomal vesicle to the overlaying plasma membrane are required.Citation8 Acrosomal secretion in human sperm has been demonstrated to be initiated when the apex of the sperm head makes the initial contact with the egg's Zona pellucida.Citation9 This event leads to a “zipper-like” formation of multiple fusion pores which propagate to the posterior part of the acrosomeCitation10 and hence accomplish the required entire dispersal of acrosomal contents. The complete fusion of the apical part of the outer acrosomal membrane and the opposed plasma membrane is also necessary to assure exposure of the inner acrosomal membrane in order to fuse with the oocyte's plasma membrane (reviewed in ref. Citation11).
Although the acrosome reaction differs from other known exocytotic processes, mainly because acrosomal secretion is an irreversible “all-or-nothing” event, the single and large acrosome resembles secretory vesicles of neurons and somatic cells.Citation11 Accordingly, acrosomal secretion shows remarkable parallels to vesicle fusion in neurons and neuroendocrine/exocrine cells (reviewed in ref. Citation12). These conspicuous similarities include the core fusion apparatus, consisting of the membrane-bridging proteins called SNAREs (soluble N-ethylmaleimide-sensitive factor attachment protein receptors).Citation13–Citation15 In addition, a Ca2+-controlled pre-fusion clamp has recently been described in spermatozoa.Citation16 Comparable mechanisms in neuroendocrine cells lock the fully assembled SNARE-fusion apparatus at an intermediate pre-fusion stage (reviewed in ref. Citation17), thus preventing vesicle fusion in the absence of a physiological signal.Citation18
Morphological segmentation is known from various cell types, such as the division in soma, dendrite and axon in neurons,Citation19 the inner and outer segment in vertebrate rod and cone photoreceptor cells20 and the apical and basolateral membrane regions in epithelial cells,Citation21 but it also represents a prominent feature of spermatozoa (). A polarized morphology appears to be a successful cellular principle to reliably divide distinct cell signaling operations.Citation22 However, even smaller subcellular domains within polarized cells have been described: This notion is exemplified by the clustering of signaling constituents in liquid-ordered membrane microdomains, termed “lipid rafts”.Citation23,Citation24 In addition, scaffolding proteins can assemble members of a signaling pathway into macromolecular “signalosomes”Citation25 and are also able to interact with other organizing cellular structures, such as lipid rafts and the actin cytoskeleton.Citation26,Citation27 Since recent observations indicate that lipid rafts and a variety of scaffolding proteins are also present in sperm cells, we summarize new findings about subcellular sorting principles in sperm and their functional significance for acrosomal secretion.
Lipid Rafts as Transduction Platforms for the Acrosome Reaction
The membrane organizing principle of spatial sequestration by liquid-ordered domains, termed lipid rafts, is an evolutionarily conserved mechanism which ensures correct subcellular localization of molecular constituents.Citation28 Lipid rafts are dynamic nanoscale assemblies of sphingolipids and cholesterolCitation29 within the phospholipid bilayer of the plasma membrane. These platforms are less fluid than the surrounding membrane and maintain a certain degree of rigidity within the neighboring phospholipid bilayer.Citation28,Citation30,Citation31 Due to their particular composition, lipid rafts are characterized by a resistance to extraction with non-ionic detergents, such as Triton X-100 or CHAPS, at low temperature (4°C)Citation32,Citation33 and hence are often referred to as detergent-resistant membranes (DRMs) or detergent-insoluble glycolipid-enriched domains (DIGs).Citation34,Citation35
The growing interest in lipid rafts has been sparked by the observation that DIG-derived lipid clusters appear to act as structural platforms which recruit specific proteins involved in signaling pathways.Citation36 In addition, lipid raft/caveolar membrane microdomains are not static but rather dynamic membrane regions: Raft platforms are able to cluster signaling molecules leading to the formation of multi-unit transduction complexes.Citation37–Citation40 Furthermore, evidence is accumulating that DIGs not only recruit certain proteins and lipids, but that they are able to transiently interact with certain cellular constituents and can even exclude binding of distinct components.Citation40 Hence, they can ensure that components which participate in a defined signal transduction process work in an undisturbed, spatially and temporally coordinated manner.Citation40–Citation42
In the past decade it has been shown for invertebratesCitation43,Citation44 as well as for different mammalian species that DIGs also contribute to spatial organization of the plasma membrane of spermatozoa. This was shown by conventional biochemical extraction methods using their partial insolubility in ice-cold non-ionic detergents. In addition, membrane raft protein markers, like caveolinCitation45,Citation46 and flotillin,Citation47 have been shown to be expressed in mammalian spermatozoa.Citation48–Citation54 Remarkably, isolated sperm-derived rafts have the ability to selectively bind to the Zona pellucida.Citation53,Citation55–Citation59 This observation led to the concept that lipid rafts in spermatozoa may represent platforms for Zona pellucida binding proteinsCitation58,Citation60 and downstream signaling components involved in Ca2+-regulated acrosomal secretionCitation57 (see also ). This model is supported by the observation that molecules involved in acrosome reaction are highly enriched in sperm-derived lipid rafts, like for instance members of the SNARE fusion machinery, including R-SNAREs like synaptobrevin (VAMP) and Q-SNAREs like syntaxin, the Ca2+ sensor protein synaptotagmin and the ATPase NSF (reviewed in ref. Citation58 and Citation61). Moreover, a capacitation-dependent polarized re-distribution to the designated site of sperm/egg interaction has been described: While DRMs in the acrosome of uncapacitated sperm are more or less uniformly distributed, the raft membrane microdomains of capacitated sperm are concentrated at the egg recognition site, where they seem to cluster and form higher ordered “macro” raft platformsCitation53,Citation56,Citation57,Citation62 (see also ). The intracellular pathway/s regulating this directed migration of rafts during sperm capacitation is/are not yet known.Citation55,Citation58,Citation61 However, a comparable scenario of a polarized reorganization of DRMs to sites of inter-cellular recognition, as well as the subsequent controlled and orchestrated transmission of extracellular ligand-binding into intracellular activity, is well known from signaling of immune cells; at the contact site of the antigen presenting cell and the T-lymphocyte, lipid rafts were found to move and cluster, thereby creating a large-scale and stable region of ordered rafts, termed “immunological synapse” (reviewed in ref. Citation63). Taking into consideration that the integrity of the immunological synapse is crucial to initiate and terminate a cognate immune response,Citation64,Citation65 and that acrosomal secretion is a prerequisite for the sperm to successfully fertilize an egg, one may speculate that capacitation-dependent re-organization of the lipid raft architecture in spermatozoa results in the creation of an “acrosomal synapse” (), representing the structural basis responsible for fine-tuned signal transduction during acrosomal exocytosis.
Scaffolding Proteins Spatially Organize Signaling Molecules in Spermatozoa
Another important principle to assure proper subcellular localization of signaling molecules is realized by scaffolding proteins, which simultaneously bind two or sometimes even several different constituents of a signaling pathway. As they are also capable of binding to other scaffolding proteins or even to scaffolding proteins of their own kind, they are able to form highly interwoven protein networks of transduction components, often involved in the same signaling process (reviewed in ref. Citation25). The resulting close-joining of functionally related transmembrane and/ or cytosolic transduction molecules ensures precise interaction with respect to each other, but also within special regions of a cell.Citation66 There is accumulating evidence that besides their role in determining spatial localization, scaffolding proteins are not only passive molecular “tapes;” scaffolding proteins are able to dynamically modify the activity of signaling reactions by coordinating positive and negative feedback signals.Citation36,Citation67 Furthermore, it has been shown that such adapter proteins can protect activated signaling molecules from inactivation, e.g., by regulating the accessibility of phosphatases.Citation68
During the last decade, the list of specific binding modules has been enormously expanded. Currently, it is estimated that a multitude of families of independent protein binding motifs exist in mammals that have evolved to recognize unique target structures, thereby governing the specificity and fidelity of signal transduction processes (reviewed in ref. Citation69 and Citation70). Among the most commonly used structural binding motifs are PDZ domains.Citation71–Citation73 PDZ is an acronym combining the first letters of the three proteins first described to contain such a structural binding module: The postsynaptic density protein (PSD95),Citation74 the Drosophila homologue discs large tumor suppressor (DlgA) gene productCitation75 and the tight junction protein zonula occludens-1 (ZO1).Citation76 The PDZ domain interacting module comprises a globular structure of about 80–90 amino acid residues,Citation77,Citation78 capable of binding the extreme C-terminal region of a target protein,Citation79 internal peptide sequences,Citation80 lipidsCitation81 or even other PDZ binding modules.Citation82 Remarkably, PDZ domains are found as single binding motifs or as multiple repeats within a wide variety of proteins.Citation73 Based on the modular structure and on the ability to recruit signaling components, PDZ domain scaffolding proteins are ideally suited to act as “nucleators” for large signal transduction complexes.Citation26,Citation83
Until now, a variety of PDZ domain proteins have been identified in mammalian spermatozoa. Some of them have been found to play a crucial role during spermatogenesis, like the ubiquitin ligase membrane-associated RING-CH (March-XI),Citation84 the junctional adhesion molecule RA175,Citation85 the Golgi-associated PDZ and coiled-coil motif-containing protein (GOPC),Citation86,Citation87 its binding partner protein interacting with C kinase (PICK1)Citation88 and the glutamate receptor-interacting protein 1 (GRIP1).Citation89 In addition, certain PDZ domain proteins have been described that fulfill their task after ejaculation; the GTPase target protein rhophilin, together with its testis specific binding partner ropporin, both localized in the fibrous sheath of sperm flagella, have been suggested to control flagellar motility.Citation90
In the sperm head, two PDZ scaffolding proteins have been identified. Remarkably, both adapter proteins are also characterized by a prominent expression profile in the mammalian nervous system, where they create macromolecular signaling complexes at the site of synaptic transmission; the multi domain scaffolding protein CASK,Citation91 also known as Lin-2, a member of the membrane-associated guanylate kinase (MAGUK) super-family, which is composed of a C-terminal guanylate kinase domain (GUK), a N-terminal Ca2+/calmodulin kinase domain, two L27 domains, a central PDZ and a Src homology (SH3) binding domain.Citation92 CASK is not involved in the formation of the fusion core,Citation93 but serves as a scaffold at the presynaptic terminal to cluster synaptic proteins, including the synaptic scaffold Mint1,Citation94 which in turn interacts with the vesicle docking protein Munc18/Sec1,Citation95 and Velis, a protein operative in synapse formation.Citation96 In addition, CASK recruits synaptic cell adhesion molecules, like neurexinsCitation94 and synaptic cell adhesion molecules (SynCAMs),Citation97 and interacts as well with Ca2+ and K+ channels (reviewed in ref. Citation92).
The second neuronal PDZ scaffolding protein identified in mammalian spermatozoa is the Multi-PDZ domain protein 1 (MUPP1)Citation98 which is characterized by 13 consecutive PDZ domainsCitation99 and a single L27 domain in its amino terminus.Citation82 Initially, MUPP1 was identified as a protein that interacts with the C-terminus of the serotonin type 2C receptor.Citation99–Citation101 Further studies showed that MUPP1 is also a scaffold for not only other G protein coupled receptors, like olfactory receptors,Citation102 the somatostatin receptor 3,103 and the melatonin receptor,Citation104 but for K+ channels,Citation105 neuronal Rho-GEF,Citation106,Citation107 the cytoplasmic adapter protein TAPP1,Citation108 a synaptic GTPase-activating protein (SynGAP),Citation109 the Ca2+/calmodulin-dependent protein kinase II (CaMKII),Citation109,Citation110 the membrane-spanning proteoglycan NG2,Citation111 viral transforming oncoproteins,Citation112–Citation114 the receptor tyrosine kinase c-kit,Citation115 as well as components of the epithelial cell tight junction.Citation116–Citation20
In epididymal and ejaculated spermatozoa of different mammalian species CASKCitation91 and MUPP1Citation98 have been found to be localized to the apical acrosomal region and the equatorial segment in human and bovine spermatozoa (). The physiological role of CASK in the primary processes of fertilizationCitation91 and a possible function of MUPP1 during capacitation and/or sperm-egg fusion are still elusive. However, functional assays performed with streptolysin-permeabilized murine sperm indicate that MUPP1 is functionally active in acrosomal secretion.Citation121 Furthermore, combining exocytotic secretion assays with a photosensitive Ca2+ chelator strategy,Citation122 we found that MUPP1 is involved in a process prior to the efflux of Ca2+ from the acrosomal vesicle.Citation121 Therefore MUPP1 might either be involved in recruiting proteins controlling pre-tethering and/or pre-docking steps of the acrosomal vesicleCitation121 following the hypothesis that acrosomal Ca2+ is necessary to stabilize dockingCitation122,Citation123 or it may control pre-fusion steps according to a model with tethering and docking processes already taking place during capacitationCitation124 (). Since the group of Gadella recently demonstrated for boar sperm cells that SNARE-complex formation indeed already occurs during capacitation,Citation125 it is most likely that MUPP1's function during acrosomal secretion in murine sperm can be considered as a post-docking, pre-fusion role. However, in our set of experiments a functional role during capacitation-dependent vesicle docking has not been addressed and thus cannot be excluded. In this context, it is important to keep in mind that Ca2+-regulated exocytosis mediated by the conserved components of the SNARE fusion machinery is a sequential process; it includes vesicle recruitment, tight plasma membrane contact established by a sequential tethering and docking process, a subsequent priming event required to achieve complete fusion competence, Ca2+ influx elicited by an appropriate stimulus and the final vesicle fusion reaction itself.Citation126 At the presynaptic terminal, the assembly of components of the fusion machinery is essential for efficient synaptic transmission and is known to be mediated by the interaction of multiple, closely interconnected specialized scaffolding proteins,Citation127–Citation129 including PDZ domain proteins.Citation130–Citation132 Since acrosomal exocytosis is based on the conserved molecular program of conventional Ca2+-triggered exocytosis,Citation133 MUPP1 in spermatozoa may function as a scaffold in preparatory fusion steps of acrosomal exocytosis, which might be of particular importance to ensure the reliable fusion of the eminently large acrosomal vesicle. However, it is remarkable in this regard that acrosomal secretion is characterized by the formation of multiple fusion pores which spread out from the contact site with the Zona pellucida.Citation10,Citation125,Citation134,Citation135 Thus, it is imaginable that the propagating fenestration and vesiculation during acrosomal exocytosis occurs by a mechanical coupling of pre-assembled SNARE complexes, with MUPP1 positioned as a functional scaffold by PDZ/PDZ head-to-tail formation, thus arranging the pre-assembled fusion machineries below the plasma membrane.
However, MUPP1 was also found to be functionally operative in preventing accidental acrosomal secretion. This spontaneous acrosomal secretion would lead to a definite loss of the one and only secretory vesicle of a spermatozoon, and thus impairment of male fertilizing ability. As described for hippocampal neurons,Citation110 MUPP1 was found to recruit the Ca2+/calmodulin dependent protein kinase IIα isozyme (CaMKIIα) in rodent spermatozoa, a kinase also known to regulate neurotransmitter release at the active zone.Citation136,Citation137 Competitive displacement of CaMKIIα from MUPP1 or inhibition of the autonomously active state of CaMKII were observed to increase spontaneous agonist-independent acrosomal secretion,Citation16 indicating that a MUPP1/CaMKIIα complex prevents ZP-independent spontaneous acrosomal exocytosis. Equally important, MUPP1 and CaMKIIα were found to be co-localized in sperm-derived detergent-resistant membrane fractions, hence indicating that spermatozoa use two cross-linking mechanisms to position activated CaMKIIα and possibly also its target substrate/s at a defined space between the outer acrosomal membrane and the plasma membrane (see , top part). Such a dual strategy, which has been suggested to be most effective to orchestrate enzymes involved in sequential molecular reactions,Citation138 is also realized for the SNARE fusion machinery at the presynaptic junctionCitation139,Citation140 and might be of pivotal importance to prevent a spontaneous acrosome reaction in resting sperm. However, since Ca2+/calmodulin was found to release CaMKIIα from the PDZ scaffolding proteinCitation16,Citation110 (, bottom part), such a duplicate subcellular organizing principle may also be responsible for reliably linking an increase in cytosolic Ca2+ to synchronized fusion pore formation, thereby ensuring the complete release of the acrosomal contents.
Conclusion
Lipid rafts and scaffolding proteins in mammalian spermatozoa play important roles as molecular organizers in the crucial event of fertilization. So far, the available data indicate that the interaction of signaling molecules with the PDZ domain protein MUPP1 may participate in pre-fusion steps of the huge acrosomal vesicle as well as in freezing the pre-assembled fusion machinery; lipid rafts are likely to facilitate these protein-protein interactions. However, it will be necessary to elucidate which factors are responsible for the observed capacitation-dependent lateral migration of raft-derived membrane micro-domains. In addition, it will be important to obtain a complete list of proteins interacting with MUPP1 as well as to clarify the functional significance of its binding partners in order to get the whole picture of the molecular architecture and dynamics of the “acrosomal synapse”.
Conflict-of-Interest and Financial Disclosure Statement
I, Ingrid Boekhoff, have no proprietary, financial, professional or other personal interest of any nature or kind in any product, service and/or company that could be construed as influencing the position presented in this manuscript.
Abbreviations
CaM | = | calmodulin |
CaMKII | = | Ca2+/calmodulin-dependent protein kinase II |
DIGs | = | detergent-insoluble glycolipid-enriched domains |
DRMs | = | detergent-resistant membranes |
IP3-R | = | inositol-1,4,5-trisphosphate receptor |
MUPP1 | = | multi PDZ domain protein 1 |
SNARE | = | soluble N-ethylmaleimide-sensitive factor attachment protein receptor |
ZP-R | = | Zona pellucida receptor |
Figures and Tables
Figure 1 Subcellular compartmentalization of a mouse sperm cell. (A) Schematic diagram of a murine sperm as an example of spermatozoon compartmentalization: The sperm head, with the acrosomal vesicle and the condensed nucleus and the flagellum with the mitochondrial-enriched mid-piece region, the principal piece and end piece segment are shown. The red dotted line demonstrates the separation between head and flagellum. (B) Hypothetical working model illustrating capacitation-dependent reorganization of lipid rafts in the plasma membrane of the acrosomal region. The two schematic drawings of the murine sperm head (upper and lower left part) show the distribution and size of raft plasma membrane platforms in the whole acrosomal region. In freshly ejaculated spermatozoa (upper parts) lipid rafts, enriched in sphingolipids (green) and cholesterol (blue), are uniformly distributed in the plasma membrane (pm) overlaying the whole acrosomal cap. In contrast, following capacitation (lower parts), rafts are more concentrated in a crescent shaped region of the sperm head. Following the size and location of rafts at higher magnification (right parts), it becomes obvious that rafts in the anterior region of the acrosomal cap in capacitated sperm aggregate upon maturation, thus leading to large-scale and stable raft clusters in the plasma membrane. Since lipid rafts in sperm recruit ZP-binding receptors as well as components of the SNARE-fusion machinery, this area may present the preferential site of hybrid vesicle formation during acrosomal secretion. This structural organization resembles the one at the active zone of the presynaptic terminal in neurons where neurotransmitter vesicle fusion occurs and thus may be termed “acrosomal synapse”. The cholesterol efflux from the plasma membrane during capacitation has been suggested to occur from the non-raft fraction and is illustrated by far less cholesterol molecules in the lower right part compared to the upper one. Possible lipid rafts in the outer acrosomal membrane (oam), and the tighter association between the two membranes, which has been suggested to occur upon capacitation, are not shown.
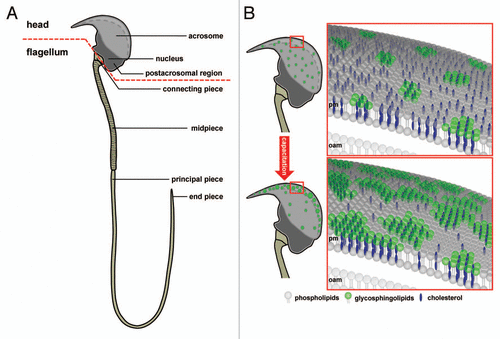
Figure 2 Subcellular localization and possible function of MUPP1 in spermatozoa. (A) Confocal laser scanning micrographs showing the subcellular localization of MUPP1 in spermatozoa of different mammalian species. Isolated spermatozoa from different mammalian species (mouse, rat, bull and man) were fixed and incubated with a specific antibody recognizing MUPP1. Antibody binding was visualized using a fluorescein-conjugated anti-rabbit IgG (green). Sperm nuclei were counter-stained with propidium iodide (PI, red). Note that MUPP1 expression in all analyzed species does not overlap with the propidium iodide labeling of the nucleus but is restricted to the acrosomal region of the sperm head and the equatorial segment (bull, man). The inserts indicate a region shown at higher magnification in the lower parts. Photomicrographs show overlays of the green and red channel and the transmitted light channel. Scale bars for the upper parts: mouse, rat: 20 µm; bull, man: 10 µm. Scale bars for the lower parts: mouse, rat and bull: 5 µm; man: 2 µm. (B) working model illustrating a possible functional role of a MUPP1/CaMKIIα complex in preventing spontaneous acrosome reaction during the sequential fusion steps of SNARE-promoted acrosomal exocytosis. The model summarizes the molecular rearrangements and modifications of proteins and lipids involved in the acrosomal fusion process, first depicting a pre-fusion state (upper part) and second at the time after fusion of the outer acrosomal membrane and the plasma membrane (lower part). After capacitation, the acrosomal vesicle has been suggested to be in a pre-fusion stage which is reflected by pre-assembled trans-SNARE complexes which force the outer acrosomal membrane (oam, grey) and the adjacent plasma membrane (pm, dark grey) to close proximity (see also insert on the left). At this stage, a catalytically active CaMKIIα, recruited by the PDZ domains 10 and/or 11 of MUPP1, is “freezing” the acrosomal exocytotic process in this intermediate fusion state, thereby preventing “accidental” spontaneous acrosomal secretion. whether this CaMKIIα/MUPP1 mediated fusion clamp is the result of a CaMKII-catalyzed phosphorylation of proteins participating in the sequential Ca2+-controlled secretion pathway, like SNARE-components and/or ion channels, is currently not known. Furthermore, it is not clear whether the observed co-localization of CaMKIIα and MUPP1 in detergent resistant membrane platforms (green) is necessary to position the kinase and possibly also its “interlocking” target/s to a defined space between the outer acrosomal membrane and the plasma membrane. Upon sperm/egg interaction, Zona pellucida ligand/s bind to complementary receptors on the capacitated sperm plasma membrane (ZP-R), thus inducing an initial transient influx of Ca2+ mediated by voltage-gated channels (Cav) and a subsequent sustained increase in Ca2+ concentration mediated by the interplay of inositol-1,4,5-trisphosphate receptors (IP3-R) on the outer acrosomal membrane and transient receptor potential channels (TRP) in the plasma membrane (bottom part). A local increase in Ca2+ (blue spots) and the formation of Ca2+ occupied calmodulin (CaM) might then be able to release the kinase from the PDZ scaffolding protein, thereby unfreezing the CaMKIIα/MUPP1 clamp. At this point, Ca2+ also triggers the final steps of membrane fusion, coinciding with the formation of hybrid vesicles at multiple sites of the membrane (see insert on the left), controlled by the calcium sensor protein synaptotagmin (synt). For the sake of simplicity, not all known molecules, which are functionally operative in the acrosome reaction (e.g., cAMP, Enkurin, EPAC, NSF, PLCδ, PI3K, Rab3A) are depicted. Localization of Q- and R-SNAREs is plotted according to reference Citation125. Cav, voltage-activated Ca2+ channel; CaMKIIα, Ca2+/calmodulin-dependent kinase IIα; IP3-R, inositol 1,4,5-trisphosphate receptor; MUPP1, Multi PDZ domain Protein 1; TRP, transient receptor potential; VAMP, vesicle-associated membrane protein/synaptobrevin; ZP-R, ZP-receptor.
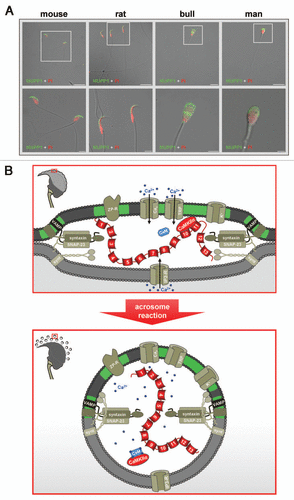
Acknowledgements
The authors wish to thank Marga Losekam and Heinz-Gerhard Janser for excellent technical assistance and Hennig Stieve for his comments on preliminary versions of this manuscript. Work in our laboratory is funded by the Hertie-Exzellenzprogramm Neurowissenschaften and the Universitätsklinikum Giessen/Marburg (Förderung gem. § 2 Abs. 3 des Kooperationsvertrages).
The authors apologize to their colleagues whose works could not be cited due to limited space requirements.
References
- Ikawa M, Inoue N, Benham AM, Okabe M. Fertilization: a sperm's journey to and interaction with the oocyte. J Clin Invest 2010; 120:984 - 994
- Austin CR. The capacitation of the mammalian sperm. Nature 1952; 170:326
- Chang MC. Fertilizing capacity of spermatozoa deposited into the fallopian tubes. Nature 1951; 168:697 - 698
- Suarez SS. Control of hyperactivation in sperm. Hum Reprod Update 2008;
- Luconi M, Baldi E. How do sperm swim? Molecular mechanisms underlying sperm motility. Cell Mol Biol (Noisy-le-Grand, France) 2003; 49:357 - 369
- Kim E, Yamashita M, Kimura M, Honda A, Kashiwabara S, Baba T. Sperm penetration through cumulus mass and Zona pellucida. Int J Dev Biol 2008; 52:677 - 682
- Travis AJ, Kopf GS. The role of cholesterol efflux in regulating the fertilization potential of mammalian spermatozoa. J Clin Invest 2002; 110:731 - 736
- Abou-Haila A, Tulsiani DR. Evidence for the capacitation-associated membrane priming of mouse spermatozoa. Histochem Cell Biol 2003; 119:179 - 187
- Wassarman PM, Litscher ES. Mammalian fertilization: the eggs multifunctional Zona pellucida. Int J Dev Biol 2008; 52:665 - 676
- Harper CV, Cummerson JA, White MR, Publicover SJ, Johnson PM. Dynamic resolution of acrosomal exocytosis in human sperm. J Cell Sci 2008; 121:2130 - 2135
- Florman HM, Jungnickel MK, Sutton KA. Regulating the acrosome reaction. Int J Dev Biol 2008; 52:503 - 510
- Mayorga LS, Tomes CN, Belmonte SA. Acrosomal exocytosis, a special type of regulated secretion. IUBMB Life 2007; 59:286 - 292
- Rothman JE. Mechanisms of intracellular protein transport. Nature 1994; 372:55 - 63
- Jahn R. Principles of exocytosis and membrane fusion. Ann NY Acad Sci 2004; 1014:170 - 178
- Sudhof TC. The synaptic vesicle cycle: a cascade of protein-protein interactions. Nature 1995; 375:645 - 653
- Ackermann F, Zitranski N, Borth H, Buech T, Gudermann T, Boekhoff I. CaMKIIalpha interacts with multi-PDZ domain protein MUPP1 in spermatozoa and prevents spontaneous acrosomal exocytosis. J Cell Sci 2009; 122:4547 - 4557
- Sudhof TC, Rothman JE. Membrane fusion: grappling with SNARE and SM proteins. Science 2009; 323:474 - 477
- Brose N. For better or for worse: complexins regulate SNARE function and vesicle fusion. Traffic 2008; 9:1403 - 1413
- Rolls MM, Satoh D, Clyne PJ, Henner AL, Uemura T, Doe CQ. Polarity and intracellular compartmentalization of Drosophila neurons. Neural Dev 2007; 2:7
- Kennedy B, Malicki J. What drives cell morphogenesis: a look inside the vertebrate photoreceptor. Dev Dyn 2009; 238:2115 - 2138
- Nejsum LN, Nelson WJ. Epithelial cell surface polarity: the early steps. Front Biosci 2009; 14:1088 - 1098
- Roodbeen R, van Hest JC. Synthetic cells and organelles: compartmentalization strategies. Bioessays 2009; 31:1299 - 1308
- Tsui-Pierchala BA, Encinas M, Milbrandt J, Johnson EM Jr. Lipid rafts in neuronal signaling and function. Trends Neurosci 2002; 25:412 - 417
- Benarroch EE. Lipid rafts, protein scaffolds and neurologic disease. Neurology 2007; 69:1635 - 639
- Burack WR, Shaw AS. Signal transduction: hanging on a scaffold. Curr Opin Cell Biol 2000; 12:211 - 216
- Kreienkamp HJ. Scaffolding proteins at the postsynaptic density: shank as the architectural framework. Handb Exp Pharmacol 2008; 365 - 380
- Glynne PA, Evans TJ. Role of the PDZ scaffolding protein in tubule cells in maintenance of polarised function. Exp Nephrol 2002; 10:307 - 312
- Ikonen E, Simons K. Protein and lipid sorting from the trans-Golgi network to the plasma membrane in polarized cells. Semin Cell Dev Biol 1998; 9:503 - 509
- Brown DA, Rose JK. Sorting of GPI-anchored proteins to glycolipid-enriched membrane subdomains during transport to the apical cell surface. Cell 1992; 68:533 - 544
- Calder PC, Yaqoob P. Lipid rafts—composition, characterization and controversies. J Nutr 2007; 137:545 - 547
- Lingwood D, Kaiser HJ, Levental I, Simons K. Lipid rafts as functional heterogeneity in cell membranes. Biochem Soc Trans 2009; 37:955 - 960
- Shogomori H, Brown DA. Use of detergents to study membrane rafts: the good, the bad and the ugly. Biol Chem 2003; 384:1259 - 1263
- Ostrom RS, Liu X. Detergent and detergent-free methods to define lipid rafts and caveolae. Methods Cell Sci 2007; 400:459 - 468
- Brown DA. Lipid rafts, detergent-resistant membranes and raft targeting signals. Physiology 2006; 21:430 - 439
- Lingwood D, Simons K. Detergent resistance as a tool in membrane research. Nat Protoc 2007; 2:2159 - 2165
- Lajoie P, Goetz JG, Dennis JW, Nabi IR. Lattices, rafts and scaffolds: domain regulation of receptor signaling at the plasma membrane. J Cell Biol 2009; 185:381 - 385
- Simons K, Ikonen E. Functional rafts in cell membranes. Nature 1997; 387:569 - 572
- Kusumi A, Ike H, Nakada C, Murase K, Fujiwara T. Single-molecule tracking of membrane molecules: plasma membrane compartmentalization and dynamic assembly of raft-philic signaling molecules. Semin Immunol 2005; 17:3 - 21
- Hoessli DC, Ilangumaran S, Soltermann A, Robinson PJ, Borisch B, Nasir UdD. Signaling through sphingolipid microdomains of the plasma membrane: the concept of signaling platform. Glycoconj J 2000; 17:191 - 197
- Rajendran L, Simons K. Lipid rafts and membrane dynamics. J Cell Sci 2005; 118:1099 - 1102
- Chini B, Parenti M. G-protein coupled receptors in lipid rafts and caveolae: how, when and why do they go there?. J Mol Endocrinol 2004; 32:325 - 338
- Pani B, Singh BB. Lipid rafts/caveolae as microdomains of calcium signaling. Cell Calcium 2009; 45:625 - 633
- Ohta K, Sato C, Matsuda T, Toriyama M, Vacquier VD, Lennarz WJ, et al. Co-localization of receptor and transducer proteins in the glycosphingolipid-enriched, low density, detergent-insoluble membrane fraction of sea urchin sperm. Glycoconj J 2000; 17:205 - 214
- Belton RJ Jr, Adams NL, Foltz KR. Isolation and characterization of sea urchin egg lipid rafts and their possible function during fertilization. Mol Reprod Dev 2001; 59:294 - 305
- Couet J, Belanger MM, Roussel E, Drolet MC. Cell biology of caveolae and caveolin. Adv Drug Deliv Rev 2001; 49:223 - 235
- Williams TM, Lisanti MP. The Caveolin genes: from cell biology to medicine. Ann Med 2004; 36:584 - 595
- Langhorst MF, Reuter A, Stuermer CA. Scaffolding microdomains and beyond: the function of reggie/flotillin proteins. Cell Mol Life Sci 2005; 62:2228 - 2240
- Trevino CL, Serrano CJ, Beltran C, Felix R, Darszon A. Identification of mouse trp homologs and lipid rafts from spermatogenic cells and sperm. FEBS Lett 2001; 509:119 - 125
- Travis AJ, Merdiushev T, Vargas LA, Jones BH, Purdon MA, Nipper RW, et al. Expression and localization of caveolin-1, and the presence of membrane rafts, in mouse and Guinea pig spermatozoa. Dev Biol 2001; 240:599 - 610
- Gamboa S, Ramalho-Santos J. SNARE proteins and caveolin-1 in stallion spermatozoa: possible implications for fertility. Theriogenology 2005; 64:275 - 291
- Cross NL. Reorganization of lipid rafts during capacitation of human sperm. Biol Reprod 2004; 71:1367 - 1373
- Sleight SB, Miranda PV, Plaskett NW, Maier B, Lysiak J, Scrable H, et al. Isolation and proteomic analysis of mouse sperm detergent-resistant membrane fractions: evidence for dissociation of lipid rafts during capacitation. Biol Reprod 2005; 73:721 - 729
- van Gestel RA, Brewis IA, Ashton PR, Helms JB, Brouwers JF, Gadella BM. Capacitation-dependent concentration of lipid rafts in the apical ridge head area of porcine sperm cells. Mol Hum Reprod 2005; 11:583 - 590
- Miranda PV, Allaire A, Sosnik J, Visconti PE. Localization of low-density detergent-resistant membrane proteins in intact and acrosome-reacted mouse sperm. Biol Reprod 2009; 80:897 - 904
- Tanphaichitr N, Carmona E, Bou Khalil M, Xu H, Berger T, Gerton GL. New insights into sperm-Zona pellucida interaction: involvement of sperm lipid rafts. Front Biosci 2007; 12:1748 - 1766
- Bou Khalil M, Chakrabandhu K, Xu H, Weerachatyanukul W, Buhr M, Berger T, et al. Sperm capacitation induces an increase in lipid rafts having Zona pellucida binding ability and containing sulfogalactosylglycerolipid. Dev Biol 2006; 290:220 - 235
- Boerke A, Tsai PS, Garcia-Gil N, Brewis IA, Gadella BM. Capacitation-dependent reorganization of micro-domains in the apical sperm head plasma membrane: functional relationship with zona binding and the zona-induced acrosome reaction. Theriogenology 2008; 70:1188 - 1196
- Gadella BM, Tsai PS, Boerke A, Brewis IA. Sperm head membrane reorganisation during capacitation. Int J Dev Biol 2008; 52:473 - 480
- Jones R, Howes E, Dunne PD, James P, Bruckbauer A, Klenerman D. Tracking diffusion of GM1 gangliosides and Zona pellucida binding molecules in sperm plasma membranes following cholesterol efflux. Dev Biol 2010; 339:398 - 406
- Nixon B, Bielanowicz A, McLaughlin EA, Tanphaichitr N, Ensslin MA, Aitken RJ. Composition and significance of detergent resistant membranes in mouse spermatozoa. J Cell Physiol 2009; 218:122 - 134
- Nixon B, Aitken RJ. The biological significance of detergent-resistant membranes in spermatozoa. J Reprod Immunol 2009; 83:8 - 13
- Shadan S, James PS, Howes EA, Jones R. Cholesterol efflux alters lipid raft stability and distribution during capacitation of boar spermatozoa. Biol Reprod 2004; 71:253 - 265
- Krummel MF, Cahalan MD. The immunological synapse: a dynamic platform for local signaling. J Clin Immunol 2010; 30:364 - 372
- Dykstra M, Cherukuri A, Sohn HW, Tzeng SJ, Pierce SK. Location is everything: lipid rafts and immune cell signaling. Ann Rev Immunol 2003; 21:457 - 481
- Viola A, Contento RL, Molon B. Signaling amplification at the immunological synapse. Curr Top Microbiol Immunol 2010; 340:109 - 122
- Tsunoda S, Zuker CS. The organization of INAD-signaling complexes by a multivalent PDZ domain protein in Drosophila photoreceptor cells ensures sensitivity and speed of signaling. Cell Calcium 1999; 26:165 - 171
- Shaw AS, Filbert EL. Scaffold proteins and immune-cell signalling. Nat Rev Immunol 2009; 9:47 - 56
- Smith FD, Scott JD. Signaling complexes: junctions on the intracellular information super highway. Curr Biol 2002; 12:32 - 40
- Songyang Z. Recognition and regulation of primary-sequence motifs by signaling modular domains. Prog Biophys Mol Biol 1999; 71:359 - 372
- Zarrinpar A, Park SH, Lim WA. Optimization of specificity in a cellular protein interaction network by negative selection. Nature 2003; 426:676 - 680
- Fanning AS, Anderson JM. Protein-protein interactions: PDZ domain networks. Curr Biol 1996; 6:1385 - 1388
- Ranganathan R, Ross EM. PDZ domain proteins: scaffolds for signaling complexes. Curr Biol 1997; 7:770 - 773
- Fan JS, Zhang M. Signaling complex organization by PDZ domain proteins. Neurosignals 2002; 11:315 - 321
- Cho KO, Hunt CA, Kennedy MB. The rat brain postsynaptic density fraction contains a homolog of the Drosophila discs-large tumor suppressor protein. Neuron 1992; 9:929 - 942
- Woods DF, Bryant PJ. The discs-large tumor suppressor gene of Drosophila encodes a guanylate kinase homolog localized at septate junctions. Cell 1991; 66:451 - 464
- Itoh M, Nagafuchi A, Yonemura S, Kitani-Yasuda T, Tsukita S, Tsukita S. The 220 kD protein colocalizing with cadherins in non-epithelial cells is identical to ZO-1, a tight junction-associated protein in epithelial cells: cDNA cloning and immunoelectron microscopy. J Cell Biol 1993; 121:491 - 502
- Doyle DA, Lee A, Lewis J, Kim E, Sheng M. MacKinnon R, Crystal structures of a complexed and peptide-free membrane protein-binding domain: molecular basis of peptide recognition by PDZ. Cell 1996; 85:1067 - 1076
- Jemth P, Gianni S. PDZ domains: folding and binding. Biochemistry 2007; 46:8701 - 8708
- Saras J, Heldin CH. PDZ domains bind carboxyterminal sequences of target proteins. Trends Biochem Sci 1996; 21:455 - 458
- Sheng M, Sala C. PDZ domains and the organization of supramolecular complexes. Annu Rev Neurosci 2001; 24:1 - 29
- Gallardo R, Ivarsson Y, Schymkowitz J, Rousseau F, Zimmermann P. Structural diversity of PDZ-lipid interactions. Chembiochem 2010; 11:456 - 467
- Nourry C, Grant SG, Borg JP. PDZ domain proteins: plug and play!. Sci STKE 2003; 2003:7
- Harris BZ, Lim WA. Mechanism and role of PDZ domains in signaling complex assembly. J Cell Sci 2001; 114:3219 - 3231
- Morokuma Y, Nakamura N, Kato A, Notoya M, Yamamoto Y, Sakai Y, et al. MARCH-XI, a novel trans-membrane ubiquitin ligase implicated in ubiquitindependent protein sorting in developing spermatids. J Biol Chem 2007; 282:24806 - 24815
- Fujita E, Tanabe Y, Hirose T, Aurrand-Lions M, Kasahara T, Imhof BA, et al. Loss of partitioningdefective-3/isotype-specific interacting protein (par-3/ASIP) in the elongating spermatid of RA175 (IGSF4A/SynCAM)-deficient mice. Am J Pathol 2007; 171:1800 - 1810
- Suzuki-Toyota F, Ito C, Toyama Y, Maekawa M, Yao R, Noda T, et al. Factors maintaining normal sperm tail structure during epididymal maturation studied in Gopc−/− mice. Biol Reprod 2007; 77:71 - 82
- Yao R, Ito C, Natsume Y, Sugitani Y, Yamanaka H, Kuretake S, et al. Lack of acrosome formation in mice lacking a Golgi protein, GOPC. Proc Natl Acad Sci USA 2002; 99:11211 - 11216
- Xiao N, Kam C, Shen C, Jin W, Wang J, Lee KM, et al. PICK1 deficiency causes male infertility in mice by disrupting acrosome formation. J Clin Invest 2009; 119:802 - 812
- Irino Y, Ichinohe M, Nakamura Y, Nakahara M, Fukami K. Phospholipase Cdelta4 associates with glutamate receptor interacting protein 1 in testis. J Biochem 2005; 138:451 - 456
- Fujita A, Nakamura K, Kato T, Watanabe N, Ishizaki T, Kimura K, et al. Ropporin, a sperm-specific binding protein of rhophilin, that is localized in the fibrous sheath of sperm flagella. J Cell Sci 2000; 113:103 - 112
- Burkin HR, Zhao L, Miller DJ. CASK is in the mammalian sperm head and is processed during epididymal maturation. Mol Reprod Dev 2004; 68:500 - 506
- Hsueh YP. The role of the MAGUK protein CASK in neural development and synaptic function. Curr Med Chem 2006; 13:1915 - 1927
- Atasoy D, Schoch S, Ho A, Nadasy KA, Liu X, Zhang W, et al. Deletion of CASK in mice is lethal and impairs synaptic function. Proc Natl Acad Sci USA 2007; 104:2525 - 2530
- Biederer T, Sudhof TC. Mints as adaptors. Direct binding to neurexins and recruitment of munc18. J Biol Chem 2000; 275:39803 - 39806
- Okamoto M, Sudhof TC. Mints, Munc18-interacting proteins in synaptic vesicle exocytosis. J Biol Chem 1997; 272:31459 - 1464
- Tabuchi K, Biederer T, Butz S, Sudhof TC. CASK participates in alternative tripartite complexes in which Mint 1 competes for binding with caskin 1, a novel CASK-binding protein. J Neurosci 2002; 22:4264 - 4273
- Biederer T, Sara Y, Mozhayeva M, Atasoy D, Liu X, Kavalali ET, et al. SynCAM, a synaptic adhesion molecule that drives synapse assembly. Science 2002; 297:1525 - 1531
- Heydecke D, Meyer D, Ackermann F, Wilhelm B, Gudermann T, Boekhoff I. The multi PDZ domain protein MUPP1 as a putative scaffolding protein for organizing signaling complexes in the acrosome of mammalian spermatozoa. J Androl 2006; 27:390 - 404
- Ullmer C, Schmuck K, Figge A, Lubbert H. Cloning and characterization of MUPP1, a novel PDZ domain protein. FEBS Lett 1998; 424:63 - 68
- Becamel C, Figge A, Poliak S, Dumuis A, Peles E, Bockaert J, et al. Interaction of serotonin 5-hydroxytryptamine type 2C receptors with PDZ10 of the multi-PDZ domain protein MUPP1. J Biol Chem 2001; 276:12974 - 12982
- Parker LL, Backstrom JR, Sanders-Bush E, Shieh BH. Agonist-induced phosphorylation of the serotonin 5-HT2C receptor regulates its interaction with multiple PDZ protein 1. J Biol Chem 2003; 278:21576 - 21583
- Dooley R, Baumgart S, Rasche S, Hatt H, Neuhaus EM. Olfactory receptor signaling is regulated by the post-synaptic density 95, Drosophila discs large, zonaoccludens 1 (PDZ) scaffold multi-PDZ domain protein 1. FEBS J 2009; 276:7279 - 7290
- Liew CW, Vockel M, Glassmeier G, Brandner JM, Fernandez-Ballester GJ, Schwarz JR, et al. Interaction of the human somatostatin receptor 3 with the multiple PDZ domain protein MUPP1 enables somatostatin to control permeability of epithelial tight junctions. FEBS Lett 2009; 583:49 - 54
- Guillaume JL, Daulat AM, Maurice P, Levoye A, Migaud M, Brydon L, et al. The PDZ protein mupp1 promotes Gi coupling and signaling of the Mt1 melatonin receptor. J Biol Chem 2008; 283:16762 - 6771
- Sindic A, Huang C, Chen AP, Ding Y, Miller-Little WA, Che D, et al. MUPP1 complexes renal K+ channels to alter cell surface expression and whole cell currents. Am J Physiol 2009; 297:36 - 45
- Penzes P, Johnson RC, Sattler R, Zhang X, Huganir RL, Kambampati V, et al. The neuronal Rho-GEF Kalirin-7 interacts with PDZ domain-containing proteins and regulates dendritic morphogenesis. Neuron 2001; 29:229 - 242
- Estevez MA, Henderson JA, Ahn D, Zhu XR, Poschmann G, Lubbert H, et al. The neuronal RhoA GEF, Tech, interacts with the synaptic multi-PDZdomain-containing protein, MUPP1. J Neurochem 2008; 106:1287 - 1297
- Kimber WA, Trinkle-Mulcahy L, Cheung PC, Deak M, Marsden LJ, Kieloch A, et al. Evidence that the tandem-pleckstrin-homology-domain-containing protein TAPP1 interacts with Ptd(3,4)P2 and the multi-PDZdomain-containing protein MUPP1 in vivo. Biochem J 2002; 361:525 - 536
- Rama S, Krapivinsky G, Clapham DE, Medina I. The MUPP1-SynGAPalpha protein complex does not mediate activity-induced LTP. Mol Cell Neurosci 2008; 38:183 - 188
- Krapivinsky G, Medina I, Krapivinsky L, Gapon S, Clapham DE. SynGAP-MUPP1-CaMKII synaptic complexes regulate p38 MAP kinase activity and NMDA receptor-dependent synaptic AMPA receptor potentiation. Neuron 2004; 43:563 - 574
- Barritt DS, Pearn MT, Zisch AH, Lee SS, Javier RT, Pasquale EB, et al. The multi-PDZ domain protein MUPP1 is a cytoplasmic ligand for the membrane-spanning proteoglycan NG2. J Cell Biochem 2000; 79:213 - 224
- Lee SS, Glaunsinger B, Mantovani F, Banks L, Javier RT. Multi-PDZ domain protein MUPP1 is a cellular target for both adenovirus E4-ORF1 and high-risk papillomavirus type 18 E6 oncoproteins. J Virol 2000; 74:9680 - 9693
- Massimi P, Gammoh N, Thomas M, Banks L. HPV E6 specifically targets different cellular pools of its PDZ domain-containing tumour suppressor substrates for proteasome-mediated degradation. Oncogene 2004; 23:8033 - 8039
- Latorre IJ, Roh MH, Frese KK, Weiss RS, Margolis B, Javier RT. Viral oncoprotein-induced mislocalization of select PDZ proteins disrupts tight junctions and causes polarity defects in epithelial cells. J Cell Sci 2005; 118:4283 - 4293
- Mancini A, Koch A, Stefan M, Niemann H, Tamura T. The direct association of the multiple PDZ domain containing proteins (MUPP-1) with the human c-Kit C-terminus is regulated by tyrosine kinase activity. FEBS Lett 2000; 482:54 - 58
- Hamazaki Y, Itoh M, Sasaki H, Furuse M, Tsukita S. Multi-PDZ domain protein 1 (MUPP1) is concentrated at tight junctions through its possible interaction with claudin-1 and junctional adhesion molecule. J Biol Chem 2002; 277:455 - 461
- Jeansonne B, Lu Q, Goodenough DA, Chen YH. Claudin-8 interacts with multi-PDZ domain protein 1 (MUPP1) and reduces paracellular conductance in epithelial cells. Cell Mol Biol 2003; 49:13 - 21
- Coyne CB, Voelker T, Pichla SL, Bergelson JM. The coxsackievirus and adenovirus receptor interacts with the multi-PDZ domain protein-1 (MUPP-1) within the tight junction. J Biol Chem 2004; 279:48079 - 48084
- Lanaspa MA, Andres-Hernando A, Rivard CJ, Dai Y, Berl T. Hypertonic stress increases claudin-4 expression and tight junction integrity in association with MUPP1 in IMCD3 cells. Proc Natl Acad Sci USA 2008; 105:15797 - 157802
- Sugihara-Mizuno Y, Adachi M, Kobayashi Y, Hamazaki Y, Nishimura M, Imai T, et al. Molecular characterization of angiomotin/JEAP family proteins: interaction with MUPP1/Patj and their endogenous properties. Genes Cells 2007; 12:473 - 486
- Ackermann F, Zitranski N, Heydecke D, Wilhelm B, Gudermann T, Boekhoff I. The Multi-PDZ domain protein MUPP1 as a lipid raft-associated scaffolding protein controlling the acrosome reaction in mammalian spermatozoa. J Cell Physiol 2008; 214:757 - 768
- De Blas GA, Roggero CM, Tomes CN, Mayorga LS. Dynamics of SNARE assembly and disassembly during sperm acrosomal exocytosis. PLoS Biol 2005; 3:323
- Zanetti N, Mayorga LS. Acrosomal swelling and membrane docking are required for hybrid vesicle formation during the human sperm acrosome reaction. Biol Reprod 2009; 81:396 - 405
- Tulsiani DR, Abou-Haila A. Is sperm capacitation analogous to early phases of Ca2+-triggered membrane fusion in somatic cells and viruses?. Bioessays 2004; 26:281 - 290
- Tsai PS, Garcia-Gil N, van Haeften T, Gadella BM. How pig sperm prepares to fertilize: stable acrosome docking to the plasma membrane. PloS One 2010; 5:11204
- Wojcik SM, Brose N. Regulation of membrane fusion in synaptic excitation-secretion coupling: speed and accuracy matter. Neuron 2007; 55:11 - 24
- Specht CG, Triller A. The dynamics of synaptic scaffolds. Bioessays 2008; 30:1062 - 1074
- Schoch S, Gundelfinger ED. Molecular organization of the presynaptic active zone. Cell Tissue Res 2006; 326:379 - 391
- Wang X, Hu B, Zieba A, Neumann NG, Kasper-Sonnenberg M, Honsbein A, et al. A protein interaction node at the neurotransmitter release site: domains of Aczonin/Piccolo, Bassoon, CAST and rim converge on the N-terminal domain of Munc13-1. J Neurosci 2009;; 29:12584 - 12596
- Hata Y, Nakanishi H, Takai Y. Synaptic PDZ domain-containing proteins. Neurosci Res 1998; 32:1 - 7
- Garner CC, Nash J, Huganir RL. PDZ domains in synapse assembly and signalling. Trends Cell Biol 2000; 10:274 - 280
- Kim E, Sheng M. PDZ domain proteins of synapses. Nat Rev 2004; 5:771 - 781
- Tomes CN. Molecular mechanisms of membrane fusion during acrosomal exocytosis. Soc Reprod Fertil 2007; 65:275 - 291
- Barros C, Bedford JM, Franklin LE, Austin CR. Membrane vesiculation as a feature of the mammalian acrosome reaction. J Cell Biol 1967; 34:1 - 5
- Flechon JE. Sperm surface changes during the acrosome reaction as observed by freeze-fracture. Am J Anat 1985; 174:239 - 248
- Wang ZW. Regulation of synaptic transmission by presynaptic CaMKII and BK channels. Mol Neurobiol 2008; 38:153 - 166
- Leal-Ortiz S, Waites CL, Terry-Lorenzo R, Zamorano P, Gundelfinger ED, Garner CC. Piccolo modulation of Synapsin1a dynamics regulates synaptic vesicle exocytosis. J Cell Biol 2008; 181:831 - 846
- Bray D. Signaling complexes: biophysical constraints on intracellular communication. Ann Rev Biophys Biomol Struc 1998; 27:59 - 75
- Gil C, Cubi R, Blasi J, Aguilera J. Synaptic proteins associate with a sub-set of lipid rafts when isolated from nerve endings at physiological temperature. Biochem Biophys Res Commun 2006; 348:1334 - 1342
- Lang T. SNARE proteins and ‘membrane rafts’. J Physiol 2007; 585:693 - 698