Abstract
Amyloid Precursor Protein (APP) and its proteolytic product amyloid beta (Αβ) are critical in the pathogenesis of Alzheimer’s Disease (AD). APP gene duplication and transcriptional upregulation are linked to AD. In addition, normal levels of APP appear to be required for some physiological functions in the developing brain. Several studies in mammalian cell lines and primary neuron cultures indicate that RNA binding proteins and microRNAs interacting with regulatory regions of the APP mRNA modulate expression of APP post-transcriptionally. However, when the various mechanisms of APP post-transcriptional regulation are recruited, and which of them are acting in a synergistic fashion to balance APP protein levels, is unclear. Recent studies suggest that further investigation of the molecules and pathways involved in APP post-transcriptional regulation are warranted.
Introduction
APP is ubiquitously expressed and its biological functions are not completely understood, although the study of APP deficient mice and, more recently, the use of APP siRNAs has begun to clarify APP functions. Neuronal APP modulates neurites outgrowthCitation1 and neuronal migrationCitation2 and APP gene dosage seems to be relevant for proper migration of neuronal cells in the developing cortex.Citation2 APP expression is developmentally regulated and reaches its highest levels during postnatal brain maturation and synaptogenesis.Citation3,Citation4 APP is targeted to the presynaptic terminal as well as dendritesCitation5 where it has been found to potentiate the formation and maintenance of synapses.Citation6 Genetic ablation of APP impairs memory in mice.Citation7
APP is a type I integral membrane protein with a single trans-membrane domain, a large extracellular N-terminal region and a shorter C-terminal tail. APP is processed through two main proteolytic processing pathways: amyloidogenic processing, which leads to generation of Aβ and non-amyloidogenic processing, which is prominent in most cell types. The extracellular domain is released by either α- or β-secretase and the remaining trans-membrane domain is further cleaved by γ-secretase. The cleavage of APP by α-secretase within the Aβ sequence precludes the formation of Aβ peptides. Among the Aβ peptides produced by amyloidogenic processing, Aβ42 is more prone to form fibrillar aggregates.Citation8
In the brain, APP and Aβ are critical in the pathogenesis of AD,Citation9 which is the most common form of dementia in the elderly. The pathology, which affects primarily the hippocampus and the cortical areas, is characterized by early synaptic defects, neuronal dysfunction, Aβ plaques which contain Aβ aggregates and neurofibrillary tangles which consist primarily of aggregated forms of the microtubule-associated protein tau.
While familial cases of AD shed some light on the causes of the disease, more than 90% of AD cases arise sporadically and the aetiology of AD is still unknown, although neuropathological and cognitive abnormalities are similar in familial and sporadic AD.
Genetic studies have identified autosomal-dominant mutations in APP or presenilin (the key component of γ-secretase) in familial AD cases. The majority of APP mutations appear to enhance Aβ aggregation and APP cleavage by presenilin variants results in higher levels of the more amyloidogenic Aβ42 peptide, therefore Aβ load in the brain has been suggested to trigger neuronal dysfunction.Citation8 In the last decade, abnormal processing of APP has been widely investigated with the aim of developing therapeutic strategies to reduce Aβ levels in AD.
Among mutations causing familial AD, duplication of the APP gene has also been detected.Citation10 In addition, individuals with trisomy 21 (the APP gene is located on chromosome 21) are at increased risk of developing AD late in life.Citation11 APP promoter mutations that significantly increase APP expression have also been associated with AD.Citation12 Cytokines, such as IL-1 (Interleukin-1) and TGFβ, which are increased in the brain of AD patients,Citation13,Citation14 are involved in the transcriptional and post-transcriptional regulation of APP.Citation15–Citation18 Moreover, elevated levels of iron have been detected in the AD brainCitation19 and intracellular levels of APP are modulated by a mechanism similar to the post-transcriptional regulation of ferritin mRNA, by iron responsive element stem loops in the 5′ untranslated region.Citation20 Perturbations which induce ischemic conditions in the brain are also proposed to be a causal factor for ADCitation21 and increased APP expression has been observed in ischemic brain tissue.Citation22
This review summarizes the current knowledge of APP post-transcriptional regulation and examines the role post-transcriptional regulation plays in AD.
Regulation of APP mRNA Translation and Stability by RNA-Binding Proteins
APP expression is extensively regulated at the post-transcriptional level by a number of different RNA binding proteins that control both APP mRNA stability and translation (). Westermar and MalterCitation23 demonstrated that the Fragile-X Mental Retardation Protein, FMRP, binds APP mRNA and regulates its translation. FMRP is highly expressed in the brain and its reduction causes Fragile X Syndrome, which is characterized by cognitive impairment, suggesting that FMRP plays an important role in memory formation and normal cognition. FMRP harbors multiple RNA-binding motifs, including two tandem KH motifs and an RGG box, although the exact roles played by the different RNAbinding domains in target recognition are unclear. Two RNA structures have been suggested to mediate the interaction between FMRP and its target mRNAs, the G-quartet RNA motif and the RNA kissing complex. APP mRNA has been purified from subcellular preparations enriched in presynaptic and postsynaptic structures (synaptoneurosomes, SN), suggesting that APP protein, which has been previously localized to dendrites and post-synaptic densities, may be produced through local translation. Indeed, FMRP associates with APP mRNA in SN preparations, as demonstrated by immunoprecipitation and RT-PCR analysis. Moreover, FMRP is required for type 1 metabotropic glutamate receptor (mGluR)-dependent translation of APP mRNA. Stimulation with the mGluR agonist 3,5-dihydroxyphenylglycine (DHPG) induces rapid dissociation of FMRP from APP mRNA, correlated with an increase in APP translation. These data indicate that the binding of FMPR to the APP mRNA represses its translation. In vitro RNase protection assays revealed that FMRP binds to the highly conserved G-rich region shortly upstream of the predicted G-quartet motif. The relevance of this G-rich region in FMRP translational repression of APP mRNA was confirmed by a subsequent study.Citation24 Interestingly, Gorospe and co-workers found that FMRP association with APP mRNA is modulated by competitive binding of another RNA-binding protein, heterogeneous nuclear ribonucleoprotein (hnRNP) C, at the same G-rich motif. FMRP and hnRNP C appear to have opposite effects on APP mRNA translation: the binding of hnRNP C enhances translation, whereas FMRP represses it. FMRP may repress translation via different mechanisms,25 the fact that APP mRNA co-localizes with P bodies when bound to FMRP, suggests that FMRP may block translation initiation of APP mRNA by modulating the assembly of the cap dependent initiation complex.Citation26
hnRNP C promotes translation of Unr mRNA by stimulating IRES (internal ribosome entry site) dependent translation.Citation27 In the case of APP mRNA, however, it is not clear whether hnRNP C stimulation of translation is dependent on the IRES present in the APP 5′UTR or is simply the result of alleviating FMRP mediated repression. Whether hnRNP C binding of APP mRNA is linked to mGluR stimulation is also unknown. It appears that hnRNP C not only regulates the translation of APP mRNA, but also increases its stability by binding to a highly conserved 29 nt element within the 3′UTR, approximately 200 nt downstream of the stop codon.Citation28 The same 29 nt sequence is also the target of the RNA-binding protein nucleolin. However, the role of nucleolin in regulating APP mRNA translation and/or stability is presently unknown, although nucleolin has been detected in a ribonucleoprotein complex with FMRP.Citation29 While the 29 nt sequence appears to de-stabilize the APP mRNA, another sequence located in the first 52 bases downstream of the stop codon seems to have the opposite effect and increases mRNA stability.Citation30 Six cytoplasmic proteins have been shown to interact with the 52 nt element: nucleolin, Rck/p54, plasminogen activator inhibitor-RNA binding protein 1 (PAI/RBP1), Y-box binding protein 1 (YB1), autoantigen La/Sjogren syndrome antigen B (La/SS-B) and elongation factor 1α (EF1a). Whether these proteins are present in a single complex or bind APP mRNA independently, is unknown. The identified proteins may interact with each other within a complex through both RNA dependent and independent interactions. However, the role of the individual proteins in APP regulation remains to be defined. Only Rck/p54 has been studied further: overexpression of Rck/p54 increases both APP mRNA and protein levels.Citation30 Rck/p54 is a member of the DEAD-box family of RNA helicases that modulate mRNA secondary structure, which has been implicated in translation and mRNA degradation. Although the precise role of Rck/p54 in the regulation of APP mRNA stability remains to be elucidated, Rck/p54 helicase activity could maintain the APP mRNA in a favorable conformation for interacting with other elements of the multi-protein complex to stabilize the APP mRNA.Citation30
Another 81 nt sequence within the APP 3′UTR, at approximately 600 nt from the stop codon, may be involved in post-transcriptional regulation of APP. Insertion of this 81 nt cis-regulatory element into the chloramphenicol acetyltransferase (CAT) mRNA conferred TGFβ mediated mRNA stability in transfected human astrocytes. Although a TGFβ responsive protein forming a 68 kDa RNA-protein complex was proposed to mediate stabilization of the APP mRNA,Citation17 further characterization of the protein has not yet been conducted.
Interestingly, two polyadenylation sites are present in the APP 3′UTR and they give rise to a short and a long transcript. Selection of the last polyadenylation site, generating the long transcript, increased the efficiency of translation in Xenopous oocytes.Citation31 Mbella et al.Citation32 observed that the last 258 nucleotides of the 3′UTR enhanced APP mRNA translation in mammalian cells and mapped two guanine residues that are crucial for this action. Using an RNA electrophoretic mobility shift assay (REMSA), Mbella et al. determined that the shorter transcript interacts with a translational repressor and the presence of the last 258 nucleotides blocks the binding of this repressor. The molecular characterization of this RNA binding protein remains to be conducted.
More recently, using in vivo UV crosslinking with Argonaute immunoprecipitation in HEK293 cells, a fragment containing nt 1,106–1,146, near the end of the APP 3′UTR, was isolated.Citation33 Identification of the microRNAs involved in this interaction will be of considerable interest.
In addition to the regulatory elements in the 3′UTR and the coding region, the 5′UTR also contains several elements that control the translation of APP mRNA, including an IRES,Citation34 an IL-1 translation enhancer elementCitation18 and an iron responsive element (IRE).Citation35 Rogers and colleaguesCitation35 found that the levels of APP are regulated by an RNA stem loop element within the 5′UTR similar to the originally identified IRE that regulates the translation of the L- and H-ferritin mRNAs in response to intracellular iron levels.Citation36,Citation37 IRP1, one of two iron regulatory proteins (IRP), binds the APP IRE in human neuroblastoma cells in response to intracellular iron chelation with desferrioxamine.Citation20 Moreover, the increased binding of IRP1 to the APP IRE as a function of iron chelation was correlated with a rapid decrease in APP protein levels. These observations support a model previously proposed for the regulation of ferritin translation, in which IRP1, in the absence of iron, binds tightly to the ferritin mRNA IREs and prevents recruitment of the 40S ribosome at the 5′ cap sites. Increased intracellular iron also appears to affect APP mRNA translation via an IRES located within the first 50 nt of the 5′UTR. In glioma cells, endogenous APP is translated in a cap-independent manner, as demonstrated both by treatment with rapamycin (an inhibitor of cap-dependent translation) and by knocking down the cap-binding protein elongation initiation factor 4E (eIF-4E).Citation34 The actual mechanism by which the levels of iron affect APP IRES activity is unknown, since it is still unclear whether cap-dependent and cap-independent translation of APP mRNA represent two alternative mechanisms by which intracellular iron concentration controls APP levels, perhaps in different cell types or in combination with other signals. Interestingly, several studies suggest that elevated intracellular iron may be linked to neuronal loss in the AD brain, where there is an enrichment of iron without a corresponding increase of ferritin.Citation19 Iron may have multiple detrimental effects in the aetiology of AD. First, by enhancing APP synthesis and increasing Aβ peptide accumulation; second, by physically associating with amyloid plaques and potentially favouring the polymerization of Aβ. Inflammatory mechanisms have also been implicated in AD. Increased levels of IL-1 have been associated with increased APP immunoreactivity in the AD brain. In this regard, a 90 nt element in the 5′UTR upstream of the cap site conferred translational regulation by IL-1a and IL-1β to a CAT reporter gene. The IL-1 responsive 90 nt element did not affect steady-state mRNA levels of the reporter gene, but did increase its translation.Citation18
Regulation of APP Translation by microRNAs
Recently, several studies have reported that APP expression is post-transcriptionally regulated by microRNAs (), which are small, 21-nucleotides, double stranded non-coding RNAs that modulate gene expression at the post-transcriptional level in many species, including mammals. One strand of an miRNA is incorporated into the Argonaute-containing RNA-induced silencing complex (RISC) and drives the RISC to bind target mRNAs, leading to translational repression and/or mRNA destabilization.Citation38 The miRNAs can modulate the expression of multiple mRNA targets and are candidates for temporally and spatially regulating several processes in neurons.
The 3′UTR of APP mRNA is a direct target for several microRNAs: miR-106a and miR-520c,Citation39 miR-20a family members (including miR-20a, miR-106b and miR-17-5p),Citation40 and miR-101.Citation41 In transfected human HEK-293 cells, miR-520c and miR106a negatively regulate expression of reporter genes containing the predicted APP target sequence in their 3′UTR.Citation39 When overexpressed in human cell lines, both miR-106a and miR-520c reduced endogenous APP levels by 50% compared to cells transfected with a non-targeting miRNA. However, only miR-106a is expressed in a neuronal context. Overexpression of miR-20a (and miR-17-5p/106b) post-transcriptionally reduced endogenous APP expression in both non-neuronal and neuronal cells.Citation40 Moreover, reduction of miR-20a, miR-17-5p and miR-106b levels was correlated with an increase in APP protein levels in developing mouse brain and in primary cultured neurons. Although two putative miR-20 family responsive elements (REs) are predicted within the APP 3′UTR (nt 461–467 and nt 709–715) only the nt 461–467 sequence functionally interacted with miR-20 family miRNAs. Interestingly, the nt 709–715 sequence, which did not interact with miRNAs, is part of the 81 nt cis-regulatory element which associated with the 68 kDa complex that stabilizes the APP mRNA (see above). When miRNA expression levels in human AD brains were analyzed, miR-106b was significantly reduced with respect to control samples. However, since APP levels varied among the different samples, a tight correlation between miR-106b and APP levels in AD tissues was not obtained. More recently, the regulation of APP expression by the RISC/miRNA pathway was examined in primary rat hippocampal neurons.Citation41 First, silencing of Ago2 in hippocampal neurons increased APP protein levels, suggesting that APP translation may be regulated by an miRNA pathway. Among miRNAs potentially targeting the APP 3′UTR, miR101 was selected for further study because two putative miR-101 target sites (nt 242–248 and nt 531–537), which are conserved among the human, rat and murine APP genes, are present in the 3′UTR. miR-101 is a brain-enriched miRNA and its expression is inversely correlated with APP in developing rat hippocampal neurons and tissues. Using site directed mutagenesis, a functional interaction between miR-101 and the RE at nt 242–248 was demonstrated. The inhibition of endogenous miR-101 increased APP levels, whereas lentiviral-mediated miR-101 overexpression significantly reduced APP and Aβ load in hippocampal neurons. These data support the hypothesis that miR-101 is a repressor of APP expression. Moreover, as described for other miRNAs, miR-101 is downregulated in the human AD cerebral cortex,Citation42,Citation43 suggesting that miR-101 downregulation may play a role in the development of AD.
Conclusions and Perspectives
Multiple cis-acting elements present within the 5′UTR, the coding sequence or the 3′UTR of the APP mRNA and several transacting molecules including RNA binding proteins and microR-NAs, have been shown to regulate APP expression (). Many of these studies have been performed in vitro using cell lines rather than in post-mitotic neuronal cells or in vivo in the brain. However, APP regulation by FRMP has been studied both in vitro and in vivo and these studies revealed that APP post-transcriptional regulation may occur in specific neuronal compartments such as synapses.
The studies summarized above highlight the fact that APP translation may be either activated or reduced by RNA binding proteins and may also be repressed by an miRNA/RISC pathway. Are these systems working in synergistic or competitive fashion? In this regard, it will be interesting to explore whether repression of APP mediated by microRNA-3′UTR associations influences the interaction of FMRP with the coding region of the APP transcript. Indeed, in mouse brain, a functional interaction between an miRNA, Ago1, FMRP and the 3′UTR of target mRNAs has recently been described.Citation44 In addition, the possibility that microRNAs regulating APP expression through a functional interaction with the APP 3′UTR also target other molecules which affect FMRP binding to APP mRNA cannot be excluded.
Which post-transcriptional mechanisms regulating APP are present and functional in brain regions susceptible to AD remains to be determined. Indeed, preliminary investigations have indicated that IRP1 binding to the APP 5′UTR occurs in human brain tissue.Citation20 Expression profiles of miRNAs obtained from temporal cortices of sporadic AD brains have shown that miR-101 and miR-106 are downregulated compared to normal human brain tissue. The identification of molecules and pathways actively involved in APP post-transcriptional regulation in the AD brain may contribute to understanding the molecular basis of AD and lead to novel therapeutic targets.
Abbreviations
APP | = | amyloid precursor protein |
Aβ | = | amyloid beta |
AD | = | Alzheimer's disease |
miRNAs | = | microRNAs |
IL-1 | = | interleukin-1 |
TGFβ | = | transforming growth factor β |
5′UTR | = | 5′ untranslated region |
3′UTR | = | 3′ untranslated region |
FMRP | = | fragile-X mental retardation protein |
SN | = | synaptoneurosomes |
mGluR | = | type 1 metabotropic glutamate receptor |
DHPG | = | 3,5-dihydroxyphenylglycine |
hnRNPC | = | heterogeneous nuclear ribonucleoprotein C |
IRES | = | internal ribosome entry site |
CAT | = | chloramphenicol acetyltransferase |
REMSA | = | RNA electrophoretic mobility shift assay |
IRE | = | iron response element |
Res | = | responsive elements |
RISC | = | RNA-induced silencing complex |
Figures and Tables
Figure 1 Synopsis of cis- and trans-regulatory elements associated with APP post-transcriptional regulation. The 5′UTR of the APP mRNA (gray) contains an internal ribosome entry site (IRES), an iron responsive element (IRE, stem loop) which binds IRP1 and an IL-1 translation enhancer element. In the APP coding sequence (blue), the G-rich motif interacts (white circle) either with hnRNPC or FMRP. FMRP interacting with CYFIP1 (magenta) may inhibit APP translation by binding to eIF4E. FMRP association with the APP mRNA mediates its recruitment to processing bodies (Ago1, Ago2, Rck/p54). The APP 3′UTR (red) contains two regulatory elements proximal to the stop codons (white circles): a 52 nt stabilizing element recognized by six proteins YB1, La/SS-B, EF1a, nucleolin, Rck/p54 and PAIRBP1 and a 29 nt destabilizing element which binds hnRNPC and nucleolin. More distal regulatory sequences include: validated microRNA responsive elements (white triangles) for miR-101 and miR-20 family elements, an 81 nt stabilizing element which interacts with a 68 kDa protein and nt 1,106–1,146 immunoprecipitated by the Ago protein (white circles). The alternative polyadenylation site leading to a shorter APP transcript is represented by the double slash.
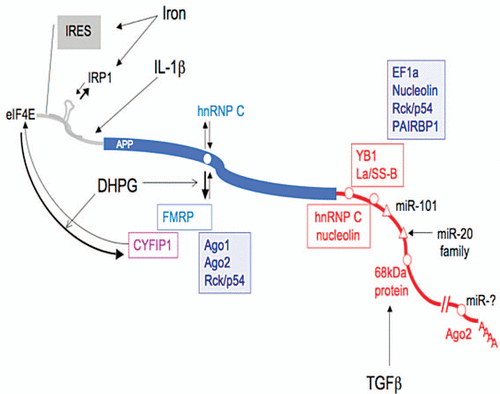
Acknowledgements
This work was supported by National Research Council (CNR) grant DG.RSTL.059.012 (to F.R.) and by Compagnia di San Paolo—Programma Neuroscienze- ‘MicroRNAs in Neurodegenerative Diseases’ (to C.B.) and FIRB RBIN04H5AS-002 and Regione Lazio Grant (to C.C.).
References
- Hoe HS, Lee KJ, Carney RS, Lee J, Markova A, Lee JY, et al. Interaction of reelin with amyloid precursor protein promotes neurite outgrowth. J Neurosci 2009; 29:7459 - 7473
- Young-Pearse TL, Bai J, Chang R, Zheng JB, LoTurco JJ, Selkoe DJ. A critical function for β-amyloid precursor protein in neuronal migration revealed by in utero RNA interference. J Neurosci 2007; 27:14459 - 14469
- Loffler J, Huber G. Beta-amyloid precursor protein isoforms in various rat brain regions and during brain development. J Neurochem 1992; 59:1316 - 1324
- Moya KL, Benowitz LI, Schneider GE, Allinquant B. The amyloid precursor protein is developmentally regulated and correlated with synaptogenesis. Dev Biol 1994; 161:597 - 603
- Sabo SL, Ikin AF, Buxbaum JD, Greengard P. The amyloid precursor protein and its regulatory protein, FE65, in growth cones and synapses in vitro and in vivo. J Neurosci 2003; 23:5407 - 5541
- Priller C, Bauer T, Mitteregger G, Krebs B, Kretzschmar HA, Herms J. Synapse formation and function is modulated by the amyloid precursor protein. J Neurosci 2006; 26:7212 - 7221
- Dawson GR, Seabrook GR, Zheng H, Smith DW, Graham S, O'Dowd G, et al. Age-related cognitive deficits, impaired long term potentiation and reduction in synaptic marker density in mice lacking the beta-amyloid precursor protein. Neuroscience 1999; 90:1 - 13
- Haass C, Selkoe DJ. Soluble protein oligomers in neurodegeneration: lessons from the Alzheimer's amyloid beta-peptide. Nat Rev Mol Cell Biol 2007; 8:101 - 112
- Hardy J. A hundred years of Alzheimer's disease research. Neuron 2006; 52:3 - 13
- Rovelet-Lecrux A, Hannequin D, Raux G, Le Meur N, Laquerrie're A, Vital A, et al. APP locus duplication causes autosomal dominantearly-onset Alzheimer disease with cerebral amyloid angiopathy. Nat Genet 2006; 38:24 - 26
- Podlisny MB, Lee G, Selkoe DJ. Gene dosage of the amyloid beta precursor protein in Alzheimer's disease. Science 1987; 238:669 - 671
- Theuns J, Brouwers N, Engelborghs S, Sleegers K, Bogaerts V, Corsmit E, et al. Promoter mutations that increase amyloid precursor-protein expression are associated with Alzheimer disease. Am J Hum Genet 2006; 78:936 - 946
- Griffin WS, Stanley LC, Ling C, White L, MacLeod V, Perrot LJ, et al. Brain interleukin 1 and S-100 immunoreactivity are elevated in Down syndrome and Alzheimer disease. Proc Natl Acad Sci USA 1989; 86:7611 - 7615
- Flanders C, Lippa CF, Smith T, Pollen DAW, Sporn MB. Altered expression of transforming growth factor-beta in Alzheimer's disease. Neurologo 1995; 45:1561 - 1569
- Docagne F, Gabriel C, Lebeurrier N, Lesné S, Hommet Y, Plawinski L, et al. Sp1 and Smad transcription factors co-operate to mediate TGFbeta-dependent activation of amyloid-beta precursor protein gene transcription. Biochem J 2004; 383:393 - 399
- Goldgaber D, Harris HW, Hla T, Maciag T, Donnelly RJ, Jacobsen JS, et al. Interleukin 1 regulates synthesis of amyloid beta-protein precursor mRNA in human endothelial cells. Proc Natl Acad Sci USA 1989; 86:7606 - 7610
- Amara FM, Junaid A, Clough RR, Liang B. TGFbeta (1), regulation of alzheimer amyloid precursor protein mRNA expression in a normal human astrocyte cell line: mRNA stabilization. Brain Res Mol Brain Res 1999; 71:42 - 49
- Rogers JT, Leiter LM, McPhee J, Cahill CM, Zhan SS, Potter H, et al. Translation of the alzheimer amyloid precursor protein mRNA is upregulated by interleukin-1 through 5′-untranslated region sequence. J Biol Chem 1999; 274:6421 - 6431
- Lovell MA, Robertson JD, Teesdale WJ, Campbell JL, Markesbery WR. Copper, iron and zinc in Alzheimer's disease senile plaques. J Neurol Sci 1998; 158:47 - 52
- Cho HH, Cahill CM, Vanderburg CR, Scherzer CR, Wang B, Huang X, et al. Selective translational control of the Alzheimer amyloid precursor protein transcript by iron regulatory protein-1. J Biol Chem 2010; 285:31217 - 31232
- Kalaria RN. The role of cerebral ischemia in Alzheimer's disease. Neurobiol Aging 2000; 21:321 - 330
- Pluta R, Kida E, Lossinsky AS, Golabek AA, Mossakowski MJ, Wisniewski HM. Complete cerebral ischemia with short-term survival in rats induced by cardiac arrest. I Extracellular accumulation of Alzheimer's beta-amyloid protein precursor in the brain. Brain Res 1994; 649:323 - 328
- Westmark CJ, Malter JS. FMRP mediates mGluR5-dependent translation of amyloid precursor protein. PLoS Biol 2007; 5:52
- Lee EK, Kim HH, Kuwano Y, Abdelmohsen K, Srikantan S, Subaran SS, et al. hnRNP C promotes APP translation by competing with FMRP for APP mRNA recruitment to P bodies. Nat Struct Mol Biol 2010; 17:732 - 739
- Zalfa F, Achsel T, Bagni C. mRNPs, polysomes or granules: FMRP in neuronal protein synthesis. Curr Opin Neurobiol 2006; 16:265 - 269
- Napoli I, Mercaldo V, Boyl PP, Eleuteri B, Zalfa F, De Rubeis S, et al. The fragile X syndrome protein represses activity-dependent translation through CYFIP1, a new 4E-BP. Cell 2008; 134:1042 - 1054
- Schepens B, Tinton SA, Bruynooghe Y, Parthoens E, Haegman M, Beyaert R, et al. A role for hnRNP C1/C2 and Unr in internal initiation of translation during mitosis. EMBO J 2007; 26:158 - 169
- Rajagopalan LE, Westmark CJ, Jarzembowski JA, Malter JS. hnRNP C increases amyloid precursor protein (APP) production by stabilizing APP mRNA. Nuleic Acid Res 1998; 26:3418 - 3423
- Ceman S, Brown V, Warren ST. Isolation of an FMRP-associated messenger ribonucleoprotein particle and identification of nucleolin and the fragile X-related proteins as components of the complex. Mol Cell Biol 1999; 19:7925 - 7932
- Broytman O, Westmark PR, Gurel Z, Malter JS. Rck/p54 interacts with APP mRNA as part of a multi-protein complex and enhances APP mRNA and protein expression in neuronal cell lines. Neurobiol Aging 2009; 30:1962 - 1974
- de Sauvage F, Kruys V, Marinx O, Huez G, Octave JN. Alternative polyadenylation of the amyloid protein precursor mRNA regulates translation. EMBO J 1992; 11:3099 - 3103
- Mbella EG, Bertrand S, Huez G, Octave JN. A GG nucleotide sequence of the 3′ untranslated region of amyloid precursor protein mRNA plays a key role in the regulation of translation and the binding of proteins. Mol Cell Biol 2000; 20:4572 - 4579
- Hafner M, Landthaler M, Burger L, Khorshid M, Hausser J, Berninger P, et al. Transcriptome-wide identification of RNA-binding protein and microRNA target sites by PAR-CLIP. Cell 2010; 141:129 - 141
- Beaudoin ME, Poirel VJ, Krushel LA. Regulating amyloid precursor protein synthesis through an internal ribosomal entry site. Nucleic Acids Res 2008; 36:6835 - 6847
- Rogers JT. An iron-responsive element type II in the 5′-untranslated region of the Alzheimer's amyloid precursor protein transcript. J Biol Chem 2002; 277:45518 - 45528
- Hentze MW, Caughman SW, Rouault TA, Barriocanal JG, Dancis A, Harford JB, et al. Identification of the iron-responsive element for the translational regulation of human ferritin mRNA. Science 1987; 238:1570 - 1573
- Klausner RD, Rouault TA, Harford JB. Regulating the fate of mRNA: the control of cellular iron metabolism. Cell 1993; 72:19 - 28
- Kim VN, Han J, Siomi MC. Biogenesis of small RNAs in animals. Nat Rev Mol Cell Biol 2009; 10:126 - 139
- Patel N, Hoang D, Miller N, Ansaloni S, Huang Q, Rogers JT, et al. MicroRNAs can regulate human APP levels. Mol. Neurodegener 2008; 3 - 10
- Hébert SS, Horré K, Nicolaï L, Bergmans B, Papadopoulou AS, Delacourte A, et al. MicroRNA regulation of Alzheimer's Amyloid precursor protein expression. Neurobiol Dis 2009; 33:422 - 428
- Vilardo E, Barbato C, Ciotti MT, Cogoni C, Ruberti F. MicroRNA-101 regulates amyloid precursor protein expression in hippocampal neurons. J Biol Chem 2010; 285:18344 - 18351
- Hébert SS, Horré K, Nicolaï L, Papadopoulou AS, Mandemakers W, Silahtaroglu AN, et al. Loss of microRNA cluster miR-29a/b-1 in sporadic Alzheimer's disease correlates with increased BACE1/beta-secretase expression. Proc Natl Acad Sci USA 2008; 105:6415 - 6420
- Nunez-Iglesias J, Liu CC, Morgan TE, Finch CE, Zhou XJ. Joint genome-wide profiling of miRNA and mRNA expression in Alzheimer's disease cortex reveals altered miRNA regulation. PloS One 2010; 5:8898
- Edbauer D, Neilson JR, Foster KA, Wang CF, Seeburg DP, Batterton MN, et al. Regulation of synaptic structure and function by FMRP-associated microRNAs miR-125b and miR-132. Neuron 2010; 65:373 - 384