Abstract
To determine the relationship between synaptic structural changes and cortical function, we recently published a study where we imaged dendritic spines using two-photon in vivo microscopy while monitoring network activity in the visual cortex using intrinsic signal imaging. By manipulating cortical activity levels by dark-rearing mice and re-exposing them to light, we found a close inverse correspondence between dendritic spine structural dynamics and visually-evoked cortical function on a timescale of days. Light exposure following dark-rearing slowly increased visually evoked cortical processing and stabilized dendritic spine structure, an effect partially mimicked by diazepam injections in dark reared mice suggesting that this slow recovery is mediated by inhibitory signaling. Surprisingly, very brief (2 hour) periods of light exposure led to an NMDA-dependent rapid re-organization of cortical networks with an early emergence of visually-evoked cortical activation and enhanced spine dynamics. Here we further explore the relationship between spine morphology and visual function.
Activity-driven remodeling of network function is thought to rely on rapid changes in neuronal function followed by a slower consolidating phase where neuronal morphology is altered.Citation1 Recent evidence, however, suggests that functional and structural changes go hand and in hand and can be equally rapid.Citation2–Citation4 Our recently published resultsCitation5 add to this debate and suggest that structural and functional synaptic changes in vivo are tightly linked on slower timescales. However, their correspondence is perturbed during rapid plastic episodes before the relationship is re-established. Here we further explore the correspondence between visually driven cortical activity and the maturity of dendritic spines assayed structurally. Because spine structure is highly linked to synapse efficacy and maturity,Citation6 we defined an “immaturity index” for dendritic spines which is calculated as the product of spine motility and the percentage of thin spines and filopodia observed for each condition divided by the percentage of “mature” spine types—mushroom and stubby (eg., from and in and ref. Citation5: the motility index of DR spines is 0.029 µm/min, the percentage of thin spines and filopodia is 28.5%, and the percentage of mushroom and stubby spines is 71.5%, yielding an immaturity index of 0.0116). To compare structural and functional changes side by side, we normalized the values of the immaturity index and the optical imaging signal to their respective controls (). Based on this analysis, both the immaturity index and cortical activation are significantly affected by dark rearing. Light exposure for 2 days has no effect on either spine maturity or cortical activation, but after 7 days of light exposure both are significantly altered, although the immaturity index does not return to control levels. A correlation analysis showed that the timescale of changes in the immaturity index and cortical activation are linked and an increase in the intensity of the optical signal correlated with a predictable decrease in the immaturity index (correlation coefficient r2 = 0.951; ). It is important to note that the 2 h time point breaks this trend (immaturity index is 0.0233 and light evoked responses are 0.00299 ΔR/R) and thus was not included in the regression analysis in . Interestingly, blocking NMDA activity with CPP restores the direct correlation between spine motility and cortical activation (correlation coefficient with 2 hrCPP included r2 = 0.91).
Traditionally, the effects of deprivation on synapses have been studied using morphological assays which examine spine density and dendritic spine morphology such as spine length and spine head width.Citation7 We compared spine density and morphology across visual manipulation in our experiment. We found no significant change in spine density with visual manipulation or drug treatment (). While this is surprising given that previous reports have found decreases of spine density with dark rearing,Citation7 this may be due to differences in cell type studied or due to the small sample size that can be obtained in in vivo imaging studies as compared to histological analysis. As spine width is hard to measure in in vivo images taken in different imaging conditions due to the small spine diameter in comparison to the resolution of two-photon microscopy, we limited our morphological analysis to spine length which in general is supraresolution and less prone to artifact. The lengths of individual groups () are significantly different from controls (Mann-Whitney p < 0.05). This suggests that the elongated phenotype typical of immature circuitry is not rescued by 2 or 7 days of light exposure.
Taken together, our data show that cortical dendritic spines in visually deprived animals are motile and morphologically immature, as evidenced by the immaturity index (). This suggests the model illustrated in : synapses in DR animals are weak and possibly transient, leading to network connectivity that does not support retinotopic organization and responds poorly to vision. After brief exposure to light, synapses remodel rapidly in an NMDA-dependent manner. Dendritic spines become highly motile and immature morphologically, suggesting large-scale synapse formation and retraction. Light stimulation may be instructive and enhance the formation of synapses that underlie visual processing while eliminating inappropriate synapses formed during dark-rearing. This is supported by our chronic experiments, which show large-scale spine formation 2 h after light re-exposure with significant spine elimination of both new and pre-existing spines at 2 days following re-exposure. This initial outgrowth of new protrusions may lead to rapid emergence of cortical visual responsiveness and retinotopic organization. This phase, however, is not maintained. Dendritic protrusions, initially highly motile and immature, either disappear leading to network re-organization or their function is limited by other mechanisms (such as changes in inhibitory drive which appear to play a role in the slower phase of recovery). This stabilization counteracts the initial functional gains and a slower process is initiated, either during initial light exposure or as a consequence of the first rapid phase of re-organization. This second phase may lead to the slow formation of more stable and mature synapses that underlie cortical visual processing or the stabilization and functional unmasking of previously established connections.
Figures and Tables
Figure 1 Dendritic spine structure and visual cortical function. (A) For each experimental group we reported the Immaturity index (gray) versus optical activation (yellow) both normalized to control values for comparison. Con, control; DR, dark-reared; DR2h, dark-reared then exposed to light for 2 h; DR2d, dark-reared then exposed to light for 2 days; DR7d, dark-reared then exposed to light for 7 days, 2hCPP, dark-reared injected with CPP and exposed to light for 2 h; DRdia, dark-reared injected with diazepam daily for 7 days. (B) Correlation analysis between immaturity index and cortical activation. For light re-exposure on the timescale of days, there is a high correlation (R2 = 0.91) between structural and functional properties, with the exception of DR2h time point (blue dot).
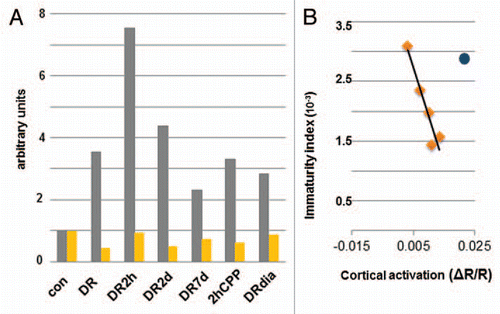
Figure 2 Visual experience does not alter the density of dendritic spines in primary visual cortex. The density of dendritic spines was determined by counting the number of spines per dendrite and dividing by the length of the dendrite in 3D. Density was calculated in the cortex of animals with different visual exposure. Here are reported the individual measurements per animal (asterisks) and the average per group (solid diamond). The density of spines was not different between different groups (Mann-Whitney; p > 0.05). N, number of animals.
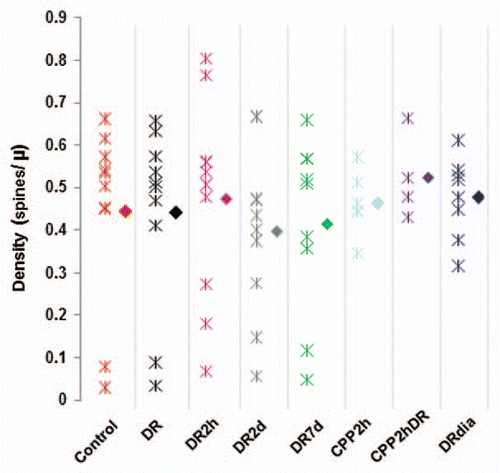
Figure 3 Visual experience controls the length of dendritic spines in primary visual cortex. The length of dendritic spines was determined by measuring each spine in the field of view in each condition. Empty triangles represent the average value per animal while solid triangles show the average value for the group. All deprived animal groups are significantly different from the controls (Mann-Whitney, p < 0.05). N, number of animals.
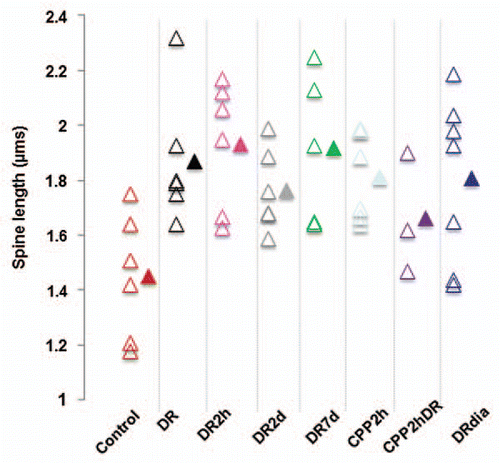
Figure 4 Light exposure induces a rapid remodeling of visual circuitry followed by stabilization. Two different phenomena modulate spine motility: one fast (within 2 h) and one slow (within 7 days). Slow recovery may be dependent on the initial fast response or may proceed through different mechanisms. Rapid changes in structural and functional events are mediated by NMDA receptors, while long-term changes are in part due to GABAergic transmission. Pink presynaptic terminals denote inappropriate connections. Gray terminals denote presynaptic terminal that form networks that serve visual processing. Pink spines denote inappropriate postsynaptic synapses in the processes of retraction.
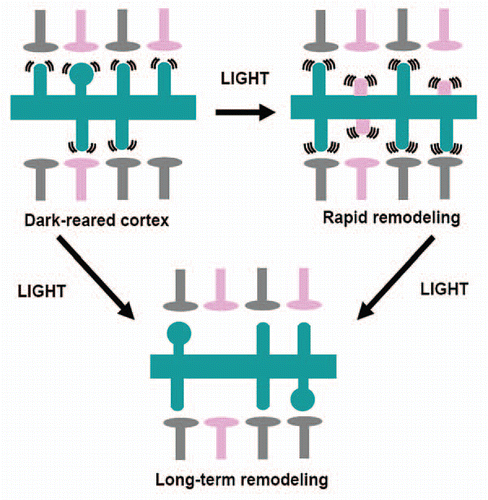
Acknowledgements
This work was funded by grants from the NIH [EY007023 and EY017098 (M.S.); 1F32EY017240 (D.T.); EY019277 (A.K.M.)]. A.K.M. was funded by the Burroughs-Wellcome Career Award in biological sciences, Arthur. P. Sloan Fellowship and a grant from the Whitehall Foundation.
Addendum To:
References
- Lamprecht R, LeDoux J. Structural plasticity and memory. Nat Rev Neurosci 2004; 5:45 - 54; PMID: 14708003
- Matsuzaki M, Honkura N, Ellis-Davies GC, Kasai H. Structural basis of long-term potentiation in single dendritic spines. Nature 2004; 429:761 - 766; PMID: 15190253
- Oray S, Majewska A, Sur M. Dendritic spine dynamics are regulated by monocular deprivation and extracellular matrix degradation. Neuron 2004; 44:1021 - 1030; PMID: 15603744
- Zhou Q, Homma KJ, Poo MM. Shrinkage of dendritic spines associated with long-term depression of hippocampal synapses. Neuron 2004; 44:749 - 757; PMID: 1557210
- Tropea D, Majewska AK, Garcia R, Sur M. Structural dynamics of synapses in vivo correlate with functional changes during experience-dependent plasticity in visual cortex. J Neurosci 2010; 30:11086 - 11095; PMID: 20720116
- Bourne JN, Harris KM. Balancing structure and function at hippocampal dendritic spines. Annu Rev Neurosci 2008; 31:47 - 67; PMID: 18284372
- Wallace W, Bear MF. A morphological correlate of synaptic scaling in visual cortex. J Neurosci 2004; 24:6928 - 6938; PMID: 15295028