Abstract
Mammalian SWI/SNF-related complexes are recruited to the promoters of numerous target genes, and the BRG1 catalytic subunit confers ATPase activity necessary to slide or evict nucleosomes and to regulate transcription. Based on gene-targeting experiments in mice, BRG1 is essential for early embryonic development. However, Brg1 null mutants have provided limited insight into gene-dosage considerations and structure-function relationships. To extend our knowledge of BRG1 function, we describe the genetic and biochemical characteristics of an ENU-induced hypomorphic mutation that encodes a protein with a single amino-acid substitution (E1083G) within the bilobal ATPase/chromatin-remodeling domain. Brg1ENU1/ENU1 mice have ~50% genetic activity and survive embryogenesis but exhibit a postnatal developmental phenotype associated with runting and incompletely penetrant lethality. The E1083G mutant protein is stable, and experiments with recombinant FLAG-tagged BRG1 proteins demonstrated that it retains full ATPase activity. Yet the biochemical activity of the mutant protein is diminished to ~50% of normal in chromatin-remodeling assays. Consistent with these findings, the E1083G substitution is predicted to disrupt a structurally conserved α-helix within the lobe that participates in DNA translocation but does not contain the ATPase catalytic site. We propose that this α-helix participates in the DNA translocation cycle by mechanistically linking DNA interaction surfaces at the DNA entry/anchor point to those within the Helicase C domain of lobe 2 of the bilobal ATPase motor. Taken together, these results demonstrate that BRG1 genetic and biochemical activities are tightly correlated. They also indicate that BRG1 ATPase activity is necessary but not sufficient for chromatin remodeling.
Introduction
SWI/SNF-related complexes are important epigenetic regulators of gene expression in eukaryotic organisms ranging from yeast to humans.Citation1 They are recruited by sequence-specific transcription factors to the promoters of downstream target genes, where they slide or evict nucleosomes in an ATP-dependent manner.Citation2 This alters the number and position of nucleosomes near transcription start sites (TSS) to regulate RNA Polymerase II occupancy and transcriptional initiation. These chromatin-remodeling complexes upregulate or downregulate ~5% of the genes in the yeast genomeCitation3,Citation4 and a similar number in the mammalian genome although there is substantial variation depending on the subunit and cell type that are analyzed.Citation5,Citation6
In mammals, SWI/SNF-related complexes are heterogeneous with different combinatorial assemblies of 9–12 canonical subunits and additional accessory factors.Citation1,Citation7 Each complex utilizes either BRG1 or BRM (also known as SMARCA4 and SMARCA2, respectively) as a catalytic subunit with DNA-dependent ATPase activity. Gene-targeting experiments in mice revealed important functional differences by demonstrating that BRG1 is essential for viability,Citation8 whereas BRM is dispensable.Citation9 The Brg1 null allele has a deletion of exons 15 and 16, which encode the ATP-binding pocket within the active site of the catalytic domain, and it also does not give rise to a stable protein.Citation8 To achieve a better understanding of BRG1 function, we previously performed an N-ethyl-N-nitrosourea (ENU) mutagenesis screen and isolated a novel point mutation in exon 23 that results in a single amino-acid substitution (E1083G) within the ATPase domain.Citation10 Compared with Brg1 null homozygotes, which die at the blastocyst stage of early embryogenesis (E3.5), Brg1null/ENU1 mutants survive until midgestation (E12.5) when they die because of fetal liver hematopoietic defects.Citation10 This phenotype suggests that ENU1 is a hypomorphic mutation, but this has not yet been verified by the analysis of ENU1/ENU1 homozygotes.
BRG1 biochemical function has been investigated using chromatin-remodeling assays. In these experiments, recombinant BRG1 protein is added to 32P-labeled DNA fragments that have a nucleosome positioned at a restriction enzyme recognition site. When BRG1 and ATP are added to this cell-free system, the conformation and position of the nucleosome is altered such that there is increased accessibility of the restriction enzyme recognition site. As a result, the corresponding restriction enzyme cleaves the template with increased efficiency, and this can be detected by performing PAGE followed by autoradiography. Although these assays have been informative,Citation11-Citation13 our mechanistic understanding of the chromatin-remodeling process is still rudimentary. For example, BRG1 ATPase activity is necessary,Citation14,Citation15 but may or may not be sufficient, for remodeling to occur. To interrogate the mechanism in more detail, BRG1 structure-function studies could be performed that compare wild type and mutant ATPase and chromatin-remodeling activities. Mutations that uncouple ATPase remodeling from chromatin remodeling would be particularly informative.
Here, we describe the genetic and biochemical characteristics of the Brg1ENU1 allele and the corresponding E1083G protein. Brg1ENU1/ENU1 mutants survive embryogenesis but are runted and exhibit postnatal lethality with incomplete penetrance. These homozygotes have ~50% genetic activity, which confirms that Brg1ENU1 is a hypomorph. In experiments with recombinant BRG1 proteins, the E1083G protein had normal ATPase activity but a ~50% reduction in chromatin-remodeling activity. These results demonstrate that ATPase activity is necessary but not sufficient for chromatin remodeling. They also demonstrate that BRG1 genetic and biochemical activities are tightly correlated. This work illustrates how genetic and biochemical approaches can be integrated to advance our mechanistic understanding of an important biological process.
Results
Brg1ENU1/ENU1 mice have ~50% genetic activity and exhibit a juvenile fitness phenotype
We previously reported that Brg1null/ENU1 mutant embryos die at midgestation with fetal liver hematopoietic defects,Citation10,Citation16-Citation18 but the Brg1ENU1/ENU1 phenotype has not yet been described. Therefore, we performed +/ENU1 intercrosses and genotyped the progeny from 24 litters. When we genotyped the litters shortly after birth (P1–P3), 23% of the pups were ENU1/ENU1 (), which is close to the expected Mendelian frequency of 25%. However, a significant number of ENU1/ENU1 homozygotes died throughout postnatal development (). As a result, when we re-genotyped the same 24 litters at weaning, only 18% of the progeny were ENU1/ENU1 (). The ENU1/ENU1 homozygotes were consistently runted from the early postnatal stage to weaning (). They remained smaller as adults when compared with +/+ and +/ENU1 littermates (which were indistinguishable from each other, Fig. S1) but were viable and fertile. The ENU1/ENU1 homozygotes are also not susceptible to tumors based on a gross anatomical examination of 50 animals that were necropsied at 1 y of age (data not shown). Based on the Brg1null/ENU1 mutant phenotype, we hypothesized that ENU1/ENU1 homozygotes would be anemic, but this was not the case (). Juvenile fitness phenotypes can arise because of many reasons, and ENU1 is a constitutive mutation rather than a tissue-specific conditional mutation. Therefore, the cause(s) of runting and death is currently unknown. Nevertheless, the postnatal phenotype demonstrates that ENU1 is a hypomorphic allele. We estimate that ENU1/ENU1 homozygotes have ~50% genetic activity based on their phenotypic similarity to +/null heterozygotes, which have BRG1 protein at ~50% of wild-type levels.Citation19 The primary similarity is that the penetrance of postnatal lethality of ENU1/ENU homozygotes and +/null heterozygotes is 22–28% and 15–30%, respectively ().
Figure 1. The Brg1ENU1/ENU1 phenotype. (A) Percentage of ENU1/ENU1 homozygotes at the perinatal stage and weaning arising from intercrosses. Data are based on 195 progeny from 24 litters of intercrosses. Compared with the expected Mendelian ratio of 25%, the results are not statistically significant (n.s.) at perinatal stage but significant (*P < 0.05) at weaning. (B) Kaplan–Meier survival curve of controls (+/+ and +/ENU1) and mutants (ENU1/ENU1). Data are based on the same 195 progeny as presented in panel A. *P < 0.05. (C) Body weights of progeny from intercrosses at weaning. Data are based on 84 weanlings from 12 litters. Squares, males; Circles, females; White, controls (+/+ or ENU1/+); Black, mutants (ENU1/ENU1). Mean weights for each class are indicated at the top with significant differences noted (*P < 0.05). (D) Hematocrits of control and mutant progeny at weaning. Data are from 5 biological replicates of each class and are presented as mean ± SE. Differences are not statistically significant (n.s.).
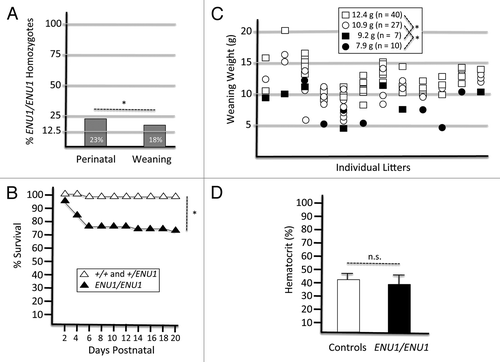
Table 1.Brg1 allelic series confers a range of genetic activities
The mutant BRG1 protein is stable and retains full ATPase activity
To assess whether the ENU1 mutant protein, which has a single amino-acid substitution (E1083G), is stable, we performed western blot analyses. We did not detect any difference in BRG1 protein levels between +/+ and ENU1/ENU1 tissue lysates from a variety of tissues (), which strongly suggests that the mutant protein is completely stable. Our previous work suggested that the mutant protein has normal ATPase activity.Citation10 However, these experiments were performed directly on sepharose beads containing BRG1-immunoprecipitated complexes, and there are caveats associated with this approach. One concern is that β-actin, which is another subunit within the complex that also has ATPase activity,Citation20 may have masked a deficit in the mutant BRG1 protein. Another possibility is that an unrelated ATPase could have been pulled down non-specifically in the immunoprecipitations. To address these concerns, we utilized the baculovirus system to synthesize and purify FLAG-tagged BRG1 recombinant proteins (Fig. S2). We then performed ATPase assays on equal amounts of the wild type and E1083G mutant proteins (). These experiments demonstrated unequivocally that the E1083G mutant protein does, in fact, have full DNA-dependent ATPase activity ().
Figure 2. The BRG1 E1083G protein is stable and has full ATPase activity. (A) Western blot analysis of several organs from +/+ controls and ENU1/ENU1 mutants. Top panel, BRG1 immunoblot; bottom panel, β-actin immunoblot. (B) Representative image of TLC autoradiograph containing ATPase reactions at different time points. 32P-labeled γATP and hydrolyzed inorganic phosphate (Pi) are indicated. (C) Percentage of ATP hydrolyzed by wild type and E1083G mutant BRG1 proteins in the presence or absence of DNA. Each value represents the mean ± SE from 3 independent experiments. Differences between wild type and mutant samples are not significant.
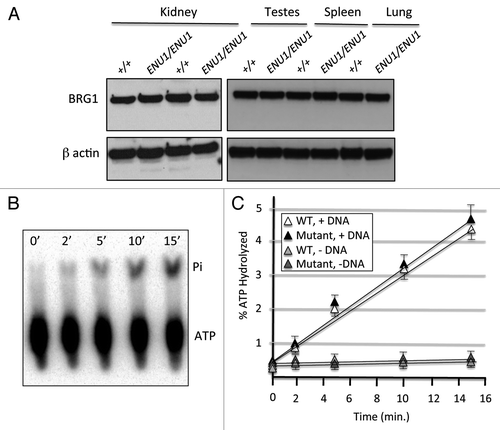
The mutant BRG1 protein has significantly impaired chromatin-remodeling activity
To interrogate further the functional properties of the E1083G mutant protein, we used the recombinant BRG1 proteins to perform chromatin-remodeling assays (). The reactions were dependent on ATP hydrolysis because significant remodeling did not occur when a non-hydrolyzable ATP analog (ATPγS) was used instead of ATP (, first lane). As a positive control, the wild type BRG1 had robust chromatin-remodeling activity based on PstI cutting the mononuclesome template, and the abundance of the cut products increased as a function of time as expected (, top panels). Compared with wild-type BRG1, the E1083G mutant had diminished remodeling activity (, middle panels). This is most evident for the upper cut band on the low exposure panel (left) and the lower cut band on the high exposure (right). As a negative control, we analyzed the K798R BRG1 mutant (, bottom panels), which is “ATPase dead.” As expected, based on previously reports,Citation14,Citation15 K798R completely lacked remodeling activity since the intensity of the cut bands was at background levels comparable to the ATPγS reactions. These results were quantified () and demonstrated that E1083G had 55% of wild-type remodeling activity ().
Figure 3. The BRG1 E1083G protein has a significant reduction in nucleosome-remodeling activity. (A) BRG1-dependent remodeling facilitates Pst1 cleavage of the TPT nucleosomal substrate. (B) Pst1 restriction enzyme accessibility assays were performed with wild type, E1083G, and K798R BRG1 protein in the presence of ATP or non-hydrolyzable ATPγS. The K798R is an “ATPase-dead” that served as a negative control. ATP-containing reactions were quenched at various time points (0.25–60 min), whereas ATPγS containing reactions were incubated for the entire 60 min time course. Shown are images of the same gel from two separate exposures. (C) Band densitometry measurements were used to calculate the fraction of uncut TPT fragments at each time point. (D) Relative remodeling activity of wild-type (set at 1) vs. mutant E1083G BRG1 (0.55) based on fraction of uncut TPT nucleosomal template. Histograms represent mean ± SE based on 3 independent experiments with significant difference noted.
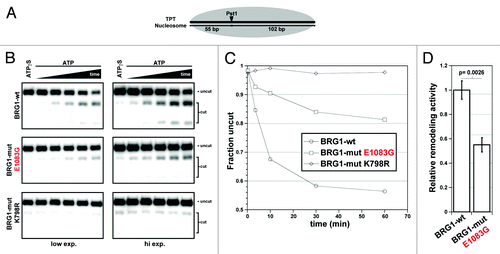
The E1083G substitution is expected to perturb the stability of an α helix within lobe 2 of the chromatin-remodeling domain
Our data indicate that the E1083G substitution uncouples ATPase activity from chromatin remodeling. To further understand the implication of these findings and the effect of the E1083G mutation on BRG1 structure (), we created protein homology predictive models of the human BRG1 ATPase domain using the known structures of 2 related SWI2/SNF2 ATPases, Saccharomyces cerevisiae CHD1 (PDB 3MWY) and zebrafish RAD54L (PDB 1Z3I).Citation21,Citation22 The ATPase domain uses ATP hydrolysis to translocate DNA through a bilobal structure consisting of several helicase-like motifs distributed between each lobe ().Citation23 Lobes 1 of the hBRG1 predictive models are topologically and structurally divergent with respect to each other, which is consistent with this region exhibiting SWI2/SNF2 subfamily-specific features ().Citation22,Citation24 This lobe contains an ATP-binding pocket within helicase-like motif I (), which is perturbed in the K798R ATPase-dead mutation. In contrast, the HD2 and Helicase C domains of lobe 2 are highly superimposable (). The E1083 residue resides within an α-helix (referred to as the HD2-HelC helix) that spans HD2 and Helicase V domains (). Secondary structure predictions for the HD2-HelC helix and neighboring motifs match known structures in scCHD1 and zRAD54L, despite primary sequence divergence (). Given these structural considerations, we modeled the effect of the E1083G mutation on α-helical formation using peptides containing wild-type or mutant HD2-HelC α-helices using algorithms that do not account for protein structure homology. The α-helical forming capacities for the wild-type peptide were similar to protein structure homology predictions for hBRG1 and the known structure of scCHD1 and zRAD54L (). However, the E-to-G mutation reduces the predicted helical forming capacity of this residue by a factor of nine (). This result is compatible with previous evidence that glycine residues are highly disruptive of α-helical formation.Citation25 Altogether, these data suggest that the E1083G mutation perturbs a structurally conserved α-helix that connects the HD2 domain to the Helicase C within lobe 2 of the BRG1 ATPase domain. This likely impairs the DNA translocation ability of the Helicase C domain, leading to significantly diminished chromatin-remodeling activity and dosage-dependent developmental phenotypes ().
Figure 4. Structural importance of the E1083 residue. (A) Human (h) BRG1 Uniprot motifs. Roman numerals and dotted lines denote helicase-like motifs. (B) Superimposition of two 3D protein homology models of human BRG1 ATPase chromatin-remodeling domain. (C) Structural annotation lobe 2 domains. Domain features are color-coded to match the stick diagram of BRG1 in panel A. The E1083 residue lies within the HD2-HelC helix (yellow). (D) Multiple sequence alignment of hBRG1, Saccharomyces cerevisiae (sc) CHD1, and zebrafish (z) RAD54L peptides containing the E1083 residue. Protein homology secondary structure predictions of the hBRG1 peptide compared with the known structures of scCHD1 and zRAD54L peptides are annotated below the alignment. (E) Predicted helicity plot of residues contained within the hBRG1 peptide shown in D.
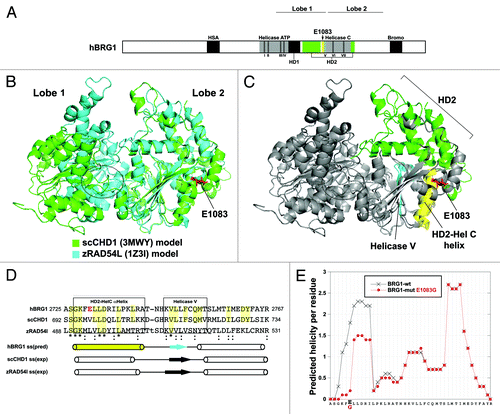
Discussion
The Brg1 allelic series presented in shows that Brg1 gene dosage and function are highly proportional. Decreasing Brg1 genetic activity to 75% does not result in a detectable phenotype, but deleterious effects do occur at a threshold of 50% or below. When genetic activity is ~50%, postnatal lethality occurs with incomplete penetrance. A further decrease to ~25% results in midgestation lethality, while 0% results in peri-implantation lethality. Decreasing Brg1 maternal gene dosage results in an even earlier developmental arrest at the 2-cell stage.Citation26 Although +/null and ENU1/ENU1 mutants both have ~50% genetic activity, they are not equivalent. The former, which express wild-type BRG1 protein at ~50% of normal levels, have an exencephaly phenotype that arises during embryogenesis.Citation8 These null heterozygotes are also susceptible to breast cancer due to haploinsufficiency rather than LOH.Citation19 In contrast, the latter express a mutant BRG1 protein at 100% of normal levels, but the biochemical activity of the mutant protein is diminished to ~50% of normal. These hypomorphic homozygotes exhibit a postnatal runting phenotype but are not tumor prone. The hypomorphic allele is particularly dosage sensitive because haploinsufficiency in null/ENU1 mutants confers completely penetrant midgestation lethality.Citation10 Brg1 dosage sensitivity is compatible with the importance of combined Brg1 and Brm genetic dosage in a subset of Brg1+/null; Brm−/− embryos that die at the peri-implantation stage.Citation27 The idea that Brg1 is dosage sensitive should be carefully considered when interpreting RNAi knockdown data because the extent of BRG1 depletion can vary.
The ENU1 allele encodes a BRG1 protein with a single amino-acid substitution (E1083G) that does not affect ATPase activity but does result in significantly decreased chromatin-remodeling activity. It is noteworthy that the magnitude of the decrease in chromatin remodeling (~55%) is commensurate with the ~50% decrease in ENU1 genetic activity. Therefore, BRG1 genetic and biochemical activity are tightly correlated. Although BRG1 structure-function studies have not been performed previously in mice, mutation of the lysine 2 amino acids proximal to E1083 in S. cerevisiae is a null, whereas mutation of the arginine 4 amino acids downstream has little or no phenotypic effect ().Citation28 Interestingly, the E1083G mutation is right between these 2 residues and is a hypomorph.
It is not surprising that the E1083G mutation does not affect ATPase activity because it is located far away from the ATPase active site. This is the case at both the primary amino-acid sequence level and in terms of the predicted three-dimensional structure (lobe 1 vs. 2 of the catalytic domain) based on X-ray crystal structures of related proteins.Citation21,Citation22 E1083 lies within an α-helix that bridges HD2 to the helicase V motif within the Helicase C domains. E1083G is predicted to prevent this α-helix from forming a stable structure, and glycine residues are known to break α-helical structure.Citation29 A similar function has been proposed for a nearby helicase-like motif V in S. cerevisiae.Citation30 Moreover, it has been suggested that ATP-driven conformational changes in lobe 2, but not lobe 1, drive DNA translocation.Citation24
It is not yet clear how the HD2-HelC helix participates in chromatin remodeling, but we propose that it mechanistically links the DNA binding surfaces within the HD2 domain to those within the Helicase C domain. In support of this idea, the HD2 domain of zRAD54L is predicted to serve as a DNA entry/anchor point.Citation22 DNA loop formation and strand propagation during the translocation cycle is thought to occur when the DNA affinities of the helicase domains alternate on DNA anchored to the HD domains.Citation22,Citation24 This is similar to tracking domain and torsion domain experimental model proposed for the RSC catalytic subunit, STH1.Citation31 Disruption of the HD2-HelC helix (in E1083G) impairs chromatin remodeling in a manner that is consistent with these ideas. Although additional structure-function studies are required to further understand these mechanistic details, the observation that ATPase activity is necessary but not sufficient for chromatin remodeling suggests that the function of the BRG1 catalytic domain is more complicated than previously appreciated. Many missense point mutations have been identified throughout the BRG1 ATPase chromatin-remodeling domain in Coffin–Siris syndrome patients and in human tumors.Citation32 To learn more about the BRG1 chromatin-remodeling mechanism, structure-function analyses could be performed on some of these amino-acid substitutions similar to this study.
Materials and Methods
Mice
The ENU1 mutation arose in an ENU mutagenesis screen on a curly whisker background as previously described.Citation10 Brg1ENU1/ENU1 mice were identified based on their curly whisker phenotype (cw/cw). To rule out a recombination event between cw and Brg1, which are 1 cM apart on proximal chromosome 9, DNA samples from toe or tail biopsies were analyzed by PCR using a microsatellite marker (D9Mit59) immediately distal to Brg1. This analysis also discriminated between +/+ and +/ENU1 mice with normal whiskers. Hematocrits were determined at Antech Diagnostics.
Western blots
Western blots utilized a BRG1 mouse monoclonal antibody that does not cross-react with BRM (Santa Cruz G-7) and a β-actin goat polyclonal antibody (Santa Cruz), and they were detected using secondary antibodies conjugated to horseradish peroxidase.
Recombinant BRG1 proteins
FLAG-tagged BRG1 proteins were expressed using the Bac-to-Bac baculovirus expression system (Invitrogen), and purified using M2 columns (Sigma). Starting with a wild-type BRG1 cDNA clone, a mutant cDNA clone was generated using the QuikChange site-directed mutagenesis kit (Stratagene). DNA sequencing verified the presence of the missense point mutation resulting in E1083G. The wild type and mutant BRG1 cDNAs were subcloned into the pFastBac plasmid (Invitrogen). DNA sequencing verified that the BRG1 coding sequence was in frame with a C-terminal FLAG tag. The constructs were transformed into DH10Bac cells containing bacmid and helper. Recombinant bacmids were then transfected using Cellfectin reagent (Invitrogen) into Sf9 insect cells, and recombinant baculovirus was used to infect insect cells. Nuclei were harvested in the presence of protease inhibitors and dialyzed. The FLAG-tagged BRG1 proteins were purified using M2 columns (Sigma). Fractions eluted with FLAG peptide (Sigma) in BC100 were quantified and analyzed on Coomassie-stained polyacrylamide gels and by western blot analysis. The FLAG-tagged BRG1 protein that is ATPase-dead (K798R) was generated the same way but purchased from Sigma.
ATPase assays
Each 20 mL reaction consisted of 30 nM BRG1, 5 mg of plasmid DNA substrate, and 0.1 mL of g32P-ATP (3000 Ci/mol) in ATP-EMSA buffer [9 mM Tris (pH 7.5), 45 mM NaCl, 4.5 mM MgCl2, 20% glycerol, 0.9 mg/ml BSA, 0.45 mM PMSF, 0.9 mM DTT, 0.45 mM ATP]. Negative control reactions lacked the plasmid DNA template. Reactions were performed at 30°C and were stopped with 5 uL of stop solution (3% SDS, 100 mM EDTA, and 50 mM Tris) Reactions were onto PEI-Cellulose TLC plates which had been prerun in double-distilled water and dried. Inorganic phosphate was separated from non-hydrolyzed ATP by running the TLC plates in 0.5 M LiCl and 1 M formic acid. The ratio of inorganic phosphate to ATP at each time point was quantified using a phosphorimager (Molecular Dynamics).
Chromatin-remodeling assays
Chromatin remodeling assays were performed as previously described.Citation12,Citation13 A mononuclesome positioning DNA fragment from the pTPT plasmidCitation14 was internally labeled with dCTP [α32P] by PCR using the following primers: (F) ACGCGTCGGT GTTAGAGC and (R) GAATTCTCTA GACAGTGTC CCA. Mononucleosomes were assembled according to the “salt-jump” methodCitation33,Citation34 in the presence of core histones from HeLa cells (Active Motif) and sonicated calf-thymus carrier DNA. Crude mononucleosome assemblies were gel-fractionated by 5%-Tris-EDTA [pH 7.6] acrylamide electrophoresis at 4 °C, and then gel purified by diffusion in 10 mM Tris-Cl [pH 7.6], 0.2 mM EDTA, 40 mM NaCl, 0.01% Igepal-CA380, 0.5 mM phenylmethylsulfonyl fluoride at 4 °C for 12–18 h. Mononucleosome assemblies were concentrated by centrifugation, and then stored at 4°C in gel elution buffer supplemented with 15% glycerol, 0.3 mg/mL bovine serum albumin (BSA), 0.1% Igepal-CA380, and 1 mM dithiothreitol. Mononucleosomes were incubated at 37 °C for 1–2 h prior to use. Gel-purified mononucleosomes (<1 nM) were incubated with either 2 mM ATP or ATPγS and saturating amounts (100 ng) of wild-type or mutant BRG1 proteins in 20 µL reactions containing 12 mM HEPES-KOH [pH 7.9], 2 mM Tris-Cl [pH 7.6], 60 mM KCl, 8 mM NaCl, 4 mM MgCl2, 0.2 mM dithiothreitol, 0.06 mg/mL bovine serum albumin (BSA), 0.02% Igepal-CA380, 3% glycerol, and 0.5 Units/µL PstI enzyme (NEB) for 0.25, 3.5, 10, 30 or 60 min. at 30 °C. Reactions were quenched by the addition of 1.6 volumes of 20 mM Tris-Cl [pH 7.6], 70 mM EDTA, 2% SDS, 0.2 mg/mL xylene cynanole, 0.2 mg/mL bromophenol blue, 0.2 mg/mL proteinase K, and 10% glycerol, followed by a 1 h incubation at 37 °C. Samples were resolved by 6%-Tris-Borate-EDTA acrylamide electrophoresis. Gels were dried then exposed to film. Band densitometry measurements from independent reactions were averaged and the standard deviations of the triplicate values measured were plotted. Statistical differences were detected using a 2-tailed Student t-test.
Protein structure modeling
Three-dimensional (3D) structural homology modeling of human BRG1 was performed using the protein homology/analogy recognition engine (Phyre).Citation35 Helical content of BRG1 peptides was calculated using the AGADIR algorithm.Citation36 3D protein structure visualization and annotation of Protein Data Bank (PDB) files were performed using the PyMOL Molecular Graphics System (pymol.org). Multiple protein sequence alignments were performed using COBALT.Citation37
Additional material
Download Zip (2.6 MB)Disclosure of Potential Conflicts of Interest
No potential conflicts of interest were disclosed.
Acknowledgments
We thank Narlikar G and members of her laboratory at UCSF, particularly Racki L, for a FLAG-tagged BRG1 cDNA clone in a baculovirus expression vector, protocols and advice on the production of recombinant protein, and help with ATPase and chromatin-remodeling assays at an early stage of this project. This work was supported, in part, by an American Cancer Society Postdoctoral Fellowship (PF-09-116-01-CCG) and an Ann Schreiber Mentored Investigator Award (258831) from the Ovarian Cancer Research Fund to Chandler RC, NIH grants to Magnuson T (R01HD036655) and Bultman SJ (RO1CA125237), and the American Institute of Cancer Research (to Bultman SJ).
References
- Ho L, Crabtree GR. Chromatin remodelling during development. Nature 2010; 463:474 - 84; http://dx.doi.org/10.1038/nature08911; PMID: 20110991
- Liu N, Balliano A, Hayes JJ. Mechanism(s) of SWI/SNF-induced nucleosome mobilization. Chembiochem 2011; 12:196 - 204; http://dx.doi.org/10.1002/cbic.201000455; PMID: 21243709
- Sudarsanam P, Iyer VR, Brown PO, Winston F. Whole-genome expression analysis of snf/swi mutants of Saccharomyces cerevisiae. Proc Natl Acad Sci U S A 2000; 97:3364 - 9; http://dx.doi.org/10.1073/pnas.97.7.3364; PMID: 10725359
- Holstege FC, Jennings EG, Wyrick JJ, Lee TI, Hengartner CJ, Green MR, Golub TR, Lander ES, Young RA. Dissecting the regulatory circuitry of a eukaryotic genome. Cell 1998; 95:717 - 28; http://dx.doi.org/10.1016/S0092-8674(00)81641-4; PMID: 9845373
- Hargreaves DC, Crabtree GR. ATP-dependent chromatin remodeling: genetics, genomics and mechanisms. Cell Res 2011; 21:396 - 420; http://dx.doi.org/10.1038/cr.2011.32; PMID: 21358755
- Euskirchen G, Auerbach RK, Snyder M. SWI/SNF chromatin-remodeling factors: multiscale analyses and diverse functions. J Biol Chem 2012; 287:30897 - 905; http://dx.doi.org/10.1074/jbc.R111.309302; PMID: 22952240
- Kadoch C, Hargreaves DC, Hodges C, Elias L, Ho L, Ranish J, Crabtree GR. Proteomic and bioinformatic analysis of mammalian SWI/SNF complexes identifies extensive roles in human malignancy. Nat Genet 2013; 45:592 - 601; http://dx.doi.org/10.1038/ng.2628; PMID: 23644491
- Bultman S, Gebuhr T, Yee D, La Mantia C, Nicholson J, Gilliam A, Randazzo F, Metzger D, Chambon P, Crabtree G, et al. A Brg1 null mutation in the mouse reveals functional differences among mammalian SWI/SNF complexes. Mol Cell 2000; 6:1287 - 95; http://dx.doi.org/10.1016/S1097-2765(00)00127-1; PMID: 11163203
- Reyes JC, Barra J, Muchardt C, Camus A, Babinet C, Yaniv M. Altered control of cellular proliferation in the absence of mammalian brahma (SNF2alpha). EMBO J 1998; 17:6979 - 91; http://dx.doi.org/10.1093/emboj/17.23.6979; PMID: 9843504
- Bultman SJ, Gebuhr TC, Magnuson TA. A Brg1 mutation that uncouples ATPase activity from chromatin remodeling reveals an essential role for SWI/SNF-related complexes in beta-globin expression and erythroid development. Genes Dev 2005; 19:2849 - 61; http://dx.doi.org/10.1101/gad.1364105; PMID: 16287714
- Phelan ML, Sif S, Narlikar GJ, Kingston RE. Reconstitution of a core chromatin remodeling complex from SWI/SNF subunits. Mol Cell 1999; 3:247 - 53; http://dx.doi.org/10.1016/S1097-2765(00)80315-9; PMID: 10078207
- Narlikar GJ, Phelan ML, Kingston RE. Generation and interconversion of multiple distinct nucleosomal states as a mechanism for catalyzing chromatin fluidity. Mol Cell 2001; 8:1219 - 30; http://dx.doi.org/10.1016/S1097-2765(01)00412-9; PMID: 11779498
- Fan HY, He X, Kingston RE, Narlikar GJ. Distinct strategies to make nucleosomal DNA accessible. Mol Cell 2003; 11:1311 - 22; http://dx.doi.org/10.1016/S1097-2765(03)00192-8; PMID: 12769854
- Sif S, Saurin AJ, Imbalzano AN, Kingston RE. Purification and characterization of mSin3A-containing Brg1 and hBrm chromatin remodeling complexes. Genes Dev 2001; 15:603 - 18; http://dx.doi.org/10.1101/gad.872801; PMID: 11238380
- Trotter KW, Fan HY, Ivey ML, Kingston RE, Archer TK. The HSA domain of BRG1 mediates critical interactions required for glucocorticoid receptor-dependent transcriptional activation in vivo. Mol Cell Biol 2008; 28:1413 - 26; http://dx.doi.org/10.1128/MCB.01301-07; PMID: 18086889
- Cohen SM, Chastain PD 2nd, Rosson GB, Groh BS, Weissman BE, Kaufman DG, Bultman SJ. BRG1 co-localizes with DNA replication factors and is required for efficient replication fork progression. Nucleic Acids Res 2010; 38:6906 - 19; http://dx.doi.org/10.1093/nar/gkq559; PMID: 20571081
- Kim SI, Bresnick EH, Bultman SJ. BRG1 directly regulates nucleosome structure and chromatin looping of the alpha globin locus to activate transcription. Nucleic Acids Res 2009; 37:6019 - 27; http://dx.doi.org/10.1093/nar/gkp677; PMID: 19696073
- Kim SI, Bultman SJ, Kiefer CM, Dean A, Bresnick EH. BRG1 requirement for long-range interaction of a locus control region with a downstream promoter. Proc Natl Acad Sci U S A 2009; 106:2259 - 64; http://dx.doi.org/10.1073/pnas.0806420106; PMID: 19171905
- Bultman SJ, Herschkowitz JI, Godfrey V, Gebuhr TC, Yaniv M, Perou CM, Magnuson T. Characterization of mammary tumors from Brg1 heterozygous mice. Oncogene 2008; 27:460 - 8; http://dx.doi.org/10.1038/sj.onc.1210664; PMID: 17637742
- Zhao K, Wang W, Rando OJ, Xue Y, Swiderek K, Kuo A, Crabtree GR. Rapid and phosphoinositol-dependent binding of the SWI/SNF-like BAF complex to chromatin after T lymphocyte receptor signaling. Cell 1998; 95:625 - 36; http://dx.doi.org/10.1016/S0092-8674(00)81633-5; PMID: 9845365
- Hauk G, McKnight JN, Nodelman IM, Bowman GD. The chromodomains of the Chd1 chromatin remodeler regulate DNA access to the ATPase motor. Mol Cell 2010; 39:711 - 23; http://dx.doi.org/10.1016/j.molcel.2010.08.012; PMID: 20832723
- Thomä NH, Czyzewski BK, Alexeev AA, Mazin AV, Kowalczykowski SC, Pavletich NP. Structure of the SWI2/SNF2 chromatin-remodeling domain of eukaryotic Rad54. Nat Struct Mol Biol 2005; 12:350 - 6; http://dx.doi.org/10.1038/nsmb919; PMID: 15806108
- Singleton MR, Wigley DB. Modularity and specialization in superfamily 1 and 2 helicases. J Bacteriol 2002; 184:1819 - 26; http://dx.doi.org/10.1128/JB.184.7.1819-1826.2002; PMID: 11889086
- Dürr H, Körner C, Müller M, Hickmann V, Hopfner KP. X-ray structures of the Sulfolobus solfataricus SWI2/SNF2 ATPase core and its complex with DNA. Cell 2005; 121:363 - 73; http://dx.doi.org/10.1016/j.cell.2005.03.026; PMID: 15882619
- Pace CN, Scholtz JM. A helix propensity scale based on experimental studies of peptides and proteins. Biophys J 1998; 75:422 - 7; http://dx.doi.org/10.1016/S0006-3495(98)77529-0; PMID: 9649402
- Bultman SJ, Gebuhr TC, Pan H, Svoboda P, Schultz RM, Magnuson T. Maternal BRG1 regulates zygotic genome activation in the mouse. Genes Dev 2006; 20:1744 - 54; http://dx.doi.org/10.1101/gad.1435106; PMID: 16818606
- Smith-Roe SL, Bultman SJ. Combined gene dosage requirement for SWI/SNF catalytic subunits during early mammalian development. Mamm Genome 2013; 24:21 - 9; http://dx.doi.org/10.1007/s00335-012-9433-z; PMID: 23076393
- Richmond E, Peterson CL. Functional analysis of the DNA-stimulated ATPase domain of yeast SWI2/SNF2. Nucleic Acids Res 1996; 24:3685 - 92; http://dx.doi.org/10.1093/nar/24.19.3685; PMID: 8871545
- O’Neil KT, DeGrado WF. A thermodynamic scale for the helix-forming tendencies of the commonly occurring amino acids. Science 1990; 250:646 - 51; http://dx.doi.org/10.1126/science.2237415; PMID: 2237415
- Smith CL, Peterson CL. A conserved Swi2/Snf2 ATPase motif couples ATP hydrolysis to chromatin remodeling. Mol Cell Biol 2005; 25:5880 - 92; http://dx.doi.org/10.1128/MCB.25.14.5880-5892.2005; PMID: 15988005
- Saha A, Wittmeyer J, Cairns BR. Chromatin remodeling through directional DNA translocation from an internal nucleosomal site. Nat Struct Mol Biol 2005; 12:747 - 55; http://dx.doi.org/10.1038/nsmb973; PMID: 16086025
- Ronan JL, Wu W, Crabtree GR. From neural development to cognition: unexpected roles for chromatin. Nat Rev Genet 2013; 14:347 - 59; http://dx.doi.org/10.1038/nrg3413; PMID: 23568486
- Stein A, Whitlock JP Jr., Bina M. Acidic polypeptides can assemble both histones and chromatin in vitro at physiological ionic strength. Proc Natl Acad Sci U S A 1979; 76:5000 - 4; http://dx.doi.org/10.1073/pnas.76.10.5000; PMID: 291918
- Duband-Goulet I, Ouararhni K, Hamiche A. Methods for chromatin assembly and remodeling. Methods 2004; 33:12 - 7; http://dx.doi.org/10.1016/j.ymeth.2003.10.015; PMID: 15039082
- Kelley LA, Sternberg MJ. Protein structure prediction on the Web: a case study using the Phyre server. Nat Protoc 2009; 4:363 - 71; http://dx.doi.org/10.1038/nprot.2009.2; PMID: 19247286
- Muñoz V, Serrano L. Elucidating the folding problem of helical peptides using empirical parameters. Nat Struct Biol 1994; 1:399 - 409; http://dx.doi.org/10.1038/nsb0694-399; PMID: 7664054
- Papadopoulos JS, Agarwala R. COBALT: constraint-based alignment tool for multiple protein sequences. Bioinformatics 2007; 23:1073 - 9; http://dx.doi.org/10.1093/bioinformatics/btm076; PMID: 17332019