Abstract
Influenza is a prevalent, highly contagious and sometimes fatal respiratory disease. Vaccination provides an effective approach to control the disease, but because of frequent changes in the structure of the major surface proteins, there is great need for a technology that permits rapid preparation of new forms of the vaccine each year in sufficient quantities. Recently, using a safe, simple, time- and cost-effective plant viral vector-based transient expression system, the hemagglutinin antigen of H1N1 influenza A strain (HAC1), an H1N1 influenza vaccine candidate, has been produced in Nicotiana benthamiana plants. As a step toward the generation of a commercially viable subunit influenza vaccine, we developed HAC1 formulations in the presence and absence of an aluminum salt adjuvant (Alhydrogel®), analyzed their properties, and assessed immunogenicity in an animal model.
Biophysical properties of HAC1 were evaluated using several spectroscopic and light scattering techniques as a function of pH and temperature combined with data analysis using an empirical phase diagram approach. Excipients that were potent stabilizers of the recombinant protein were identified using intrinsic fluorescence spectroscopy. The adsorptive capacity and thermal stability of the protein on the surface of Alhydrogel® were then examined in the presence and absence of selected stabilizers using UV absorbance after centrifugation and intrinsic fluorescence spectroscopy, respectively. Immunogenicity studies conducted in mice demonstrated that the highest level of serum immune responses (hemagglutination-inhibiting antibody titers), with a 100% seropositive rates, were induced by HAC1 in the presence of Alhydrogel®, and this response was elicited regardless of the solution conditions of the formulation.
Introduction
Influenza is one of the leading causes of respiratory infections worldwide and is associated with significant morbidity and mortality each year. There are several subtypes of the influenza virus that are currently circulating among humans.Citation1,Citation2 An effective way to combat influenza is through vaccination using a trivalent vaccine produced in embryonated chicken eggs. Almost all commercially available vaccines contain two surface glycoproteins of influenza, hemagglutinin (HA) and neuraminidase (NA), as the key antigens, with HA being the dominant one of the two. Although this system has worked well over the past five decades, it has several well-recognized disadvantages.Citation1 One of the major disadvantages of this costly and time-consuming egg-based technology is that it is difficult to respond to a potential pandemic crisis in a timely manner, since a minimum of 3–6 mo is required to generate an influenza vaccine.Citation2-Citation5 The H1N1/09 virus was responsible for the 2009 swine flu epidemic. Significant morbidity and mortality associated with this disease were due to the lack of an effective vaccine.Citation6 More than 506,060 cases of the H1N1/09 infections have been reported worldwide, with over 6770 deaths.Citation7 These numbers may have been significantly underestimated, since it is no longer required to report all clinically diagnosed cases.
To prevent or effectively diminish the impact of influenza, it is imperative to have a system that can rapidly produce the vaccine when needed. Two relatively new technologies which are being tested to replace the egg-based production system are cell culture-based and recombinant protein (antigen)-based approaches.Citation5,Citation8-Citation11 The cell culture-based technology has its own limitations since the process still requires the production of re-assorted virus similarly to the egg-based technologyCitation12,Citation13 and has a number of other safety-associated concerns.Citation5 In contrast, the recombinant protein-based approach involves the use of purified viral surface antigens (HA and/or NA) as the active component of the vaccine. HA is recognized as the key antigen in the host response induced by influenza vaccination and thus is a logical candidate for a recombinant influenza vaccine. Currently, there are no commercially available recombinant influenza vaccines, although many are in development, including purified HA-based as well as virus-like particle (VLP)-based vaccines.Citation5,Citation8-Citation11
The currently available systems for the commercial production of recombinant protein vaccines include bacteria, yeast, insect and mammalian cell cultures. Although each of these systems has specific advantages, their application and use are limited due to insufficient scalability, safety concerns, high cost and target integrity. A much cheaper, safer and highly scalable alternative for recombinant subunit vaccine production is a plant-based system that can generate proteins in their native form with the desired properties.Citation14 A number of human and animal recombinant subunit vaccines have been produced in plants to date and have been demonstrated to be efficacious and safe in preclinical and clinical studies.Citation14,Citation15
In this report, we have conducted studies to formulate a recombinant HA protein (HAC1) from H1N1 influenza A (A/California/04/09 strain) produced in Nicotiana benthamiana plants as an early developmental step toward the generation of a commercially viable vaccine. In this work, we also test the utility of accelerated stability studies.
Results
Biophysical characterization of HAC1
The CD spectrum of HAC1 at pH 7.0 and 10°C displays a broad negative peak between 205–225 nm with apparent double minima near 208 and 220 nm, indicating significant contributions from α-helices to the secondary structure of the protein (). The CD spectrum at pH 7.0 is representative of what is seen at pH of 3.0–8.0 as well. The broad peak and significant negative ellipiticity at 215 nm, however, indicates the presence of some β-sheet as well. Decreases in the molar ellipiticity at 222 nm provide evidence for the loss of the α-helical secondary structure upon thermal stress (). Only gradual transitions were evident at pH 3.0, 4.0, and 5.0, which initiate at relatively low temperatures (~20–30°C) (). A small transition is seen near 30°C at pH 6.0 (). In contrast, much sharper transitions are observed at pH 7.0 and 8.0 at 45–50°C ().
Figure 2. Circular dichroism thermal melt plots of HAC1 in imidazole buffer. The molar ellipticity at 222 nm was monitored as a function of temperature for six pH conditions—pH 3.0 (A), 4.0 (B), 5.0 (C), 6.0 (D), 7.0 (E) and 8.0 (F).
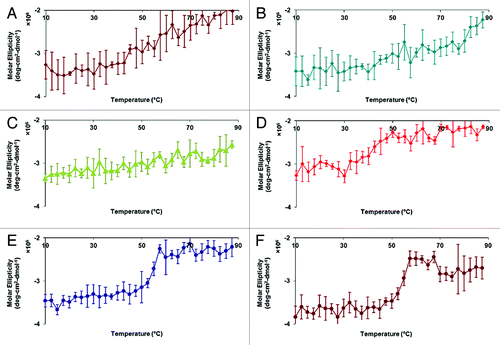
Red shifts in protein Trp peak positions occur as buried Trp residues become exposed to a more polar environment and provide evidence for changes in the tertiary structure. Again, no sharp transitions are seen at pH 3.0, 4.0, and 5.0 (). Instead, a very gradual shift is observed at these three pH values with peak positions at about 344 nm at 10°C (note that the actual peak positions are ~10 nm lower). Sharp transitions are seen at pH 7.0 and 8.0 at about 50°C, with the peak position at about 340 nm at 10°C. At pH 6.0, the protein manifests a lower thermal stability compared with pH 7.0 and 8.0, with the peak position slightly red shifted to about 342 nm at 10°C and transitions initiating above 30°C.
Figure 3. Intrinsic fluorescence peak position changes as a function of temperature for the pH range 3.0–8.0. Peak positions are determined by the mean spectral center of mass (msm) method, which shifts the actual peak positions by 8–10 nm toward the red.
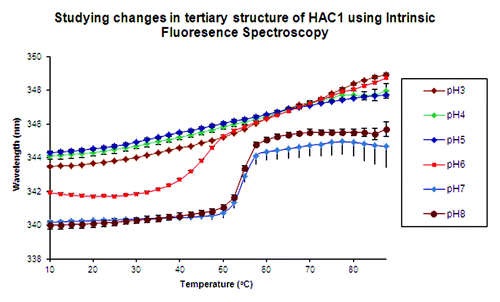
Monitoring static light scattering intensity provides insight into the aggregation behavior of proteins in solution. Despite the absence of visible aggregates even after heating to 90°C, the increasing static light intensity and corresponding transitions observed for HAC1 indicate formation of lower order aggregates with increases in temperature. At pH 3.0, 7.0, and 8.0, the aggregation transitions occur between 40–45°C (see Figure S1). At pH 6.0, the light scattering intensity gradually increases without a sharp transition. The constant and low light scattering intensity throughout the entire temperature scan at pH 4.0 and 5.0 suggests that there is no detectable aggregate formation at these pH values.
At pH 3.0–5.0, ANS shows significant fluorescence intensity at low temperatures suggesting that the tertiary structure of HAC1 is already structurally altered (see Figure S2). A gradual decrease in ANS fluorescence intensity is observed at these pH values indicating release of ANS from the protein as it further unfolds and/or increasing fluorescence quenching at higher temperatures. The transition at pH 6.0 starts at 42°C, while the transitions at pH 7.0 and 8.0 begin at higher temperature (52°C) suggesting an increased conformational stability of HAC1 around neutral pH, similar to that observed by the other techniques. Again, the protein at pH 6.0 exhibits relatively increased stability compared with pH 3.0–5.0, but to a lesser extent than that seen at pH 7.0 and 8.0 as suggested by all of the biophysical studies.
An empirical phase diagram (EPD) was created by combining the data shown above to reflect secondary and tertiary structural changes, aggregation state, and the accessibility of apolar binding sites in the protein as a function of pH and temperature (). The blue region at the lower, right-hand corner of the diagram encompassing pH 7.0 and 8.0 and temperatures below 50°C can be considered the region in which the protein is found to be in its native conformation (region I). Intrinsic fluorescence, ANS fluorescence and light scattering data all confirm this observation. Region II represents an intermediate state of the protein at pH 6.0 and temperatures below 40°C. Regions V and VI, present at pH 6.0, 7.0 and 8.0 at elevated temperatures, represent a physical state in which the protein is severely structurally altered and aggregated. Region III (green), present at pH 3.0–5.0 and below 50–60°C, represents a state of the protein that is partially structurally altered, but remains in a non-aggregated state. As the temperature is increased above 50°C at pH 3.0–5.0, additional behavior (greenish blue) is apparent. At pH 3.0, this reflects a transition observed in the static light scattering plots and represents a severely disrupted and aggregated state of the protein. At pH 4.0 and 5.0, this region represents a severely structurally altered state as indicated by CD and fluorescence data but lacks the presence of aggregates.
Excipient screening
Based on the EPD and thermal melts generated from data acquired during biophysical characterization of HAC1, pH 7.0 was selected for excipient screening based on the optimal conformational stability and aggregation profile of HAC1 at this pH. A series of GRAS compounds from a library previously found to be of wide utility were screened by fluorescence spectroscopy for their ability to enhance HAC1 conformational stability and prevent aggregation. Among those compounds evaluated in the screening were a variety of carbohydrates, nonionic surfactants, amino acids, polyalcohols and cyclodextrins (). Examples of the relative shifts in the thermal unfolding curves of HAC1 in the presence of excipients are shown in . The effect of excipients on the aggregation and conformational stability of HAC1 in imidazole buffer at pH 7.0 is summarized in in the form of Tm values. summarizes the effect of concentration of selected stabilizing excipients on both the aggregation and conformational stability of HAC1. The potential stabilizers identified include dextrose, sorbitol, glycerol, and Tween-80. As seen in , 20% sorbitol, 20% dextrose, and 20% sucrose produced the maximum increase in the conformational stability with a change in Tm of ~4°C, while Tween-80 had the highest inhibitory effect on aggregation with a change of about 8°C. The conformational destabilization caused by Tween-80, however, renders it potentially less useful as a stabilizer.
Table 1. Effect of GRAS compounds on the conformational stability (Tm-Trp) and aggregation (Tm-SLS) of HAC1 as determined by intrinsic fluorescence and static light scattering. The effect of each compound on the aggregation and conformational stability of the protein is represented as a change in Tm. Compounds that inhibit aggregation and enhance stability have increased Tm values, while compounds that induce aggregation and reduce conformational stability produced decreased Tm values
Figure 5. Comparison of thermal unfolding profiles of HAC1 in the presence of selected different stabilizers and destabilizers. Excipients are prepared in imidazole buffer (10 mM imidazole, pH 7.0, I = 0.15 M adjusted with NaCl).
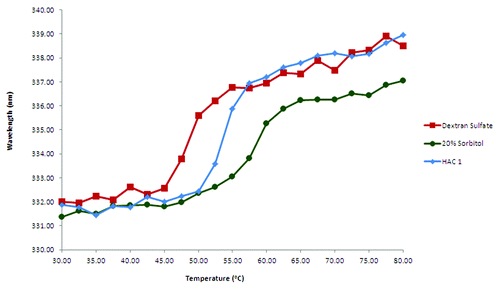
Table 2. Effect of the concentration of selected GRAS compounds on the aggregation (Tm-SLS) and the conformational stability (Tm-Trp) of HAC1 as determined by intrinsic fluorescence and static light scattering
The effects of selected excipients were concentration dependent, except for glycerol and Tween-80 (). The use of these excipients at 20% concentration is beyond recommended limits due to high osmolarity values. Hence, the use of sorbitol, dextrose and sucrose was limited to a concentration of 15%. Tween-80 at concentrations lower than 0.1% was also tested due to its inhibitory effect on aggregation despite its conformation-destabilizing effect.
The effects of combinations of excipients on thermal transitions (both aggregation and conformational stability) are summarized in Table S1A and B. The combinations of selected excipients, however, did not yield significant improvements compared with 15% sorbitol alone with respect to the stabilization of HAC1. Some combinations of excipients such as 15% sucrose and 0.05% Tween-80 showed a stabilizing effect when analyzed by static light scattering. No significant effect, however, was observed using intrinsic fluorescence. This is presumably due to the non-specific interactions of Tween-80 with the protein which inhibits aggregations. In contrast, this does not appear to protect a protein against changes in conformation.
Adjuvant binding studies
Adsorption and desorption studies of HAC1 with Alhydrogel®
The time required for the protein to adsorb onto the surface of an aluminum salt adjuvant varies for different proteins. Kinetic studies of HAC1 binding to Alhydrogel® revealed that the protein efficiently binds to Alhydrogel® at a protein concentration of 240 μg/ml and an adjuvant concentration of 0.5 mg/ml. In 10 mM imidazole buffer (pH 7.0, I = 0.15, adjusted with NaCl), all of the protein binds to the adjuvant within the first 2 min of introducing the adjuvant (data not shown). The amount of protein bound to Alhydrogel® does not change significantly during the 20 min period of testing.
The amount of protein that can be adsorbed onto the surface of Alhydrogel®, or the “adsorptive capacity” of Alhydrogel®, was determined by constructing adsorption isotherms. Adsorptive capacity is defined as the maximum amount of protein that can be adsorbed onto the surface of Alhydrogel®. In binding isotherms, 100 μg of Alhydrogel® is able to adsorb up to 160 μg of protein in 10 mM imidazole buffer (pH 7.0, I = 0.15, adjusted with NaCl). In the studies conducted, saturation of protein binding was not attained and experiments with higher protein concentrations were not conducted.
An important study that the FDA requires in the development of a vaccine that involves aluminum salts is desorbing the protein from the surface of the adjuvant. The amount of desorbed protein depends on the desorptive media and the level of interaction between the protein and the adjuvant. Standard desorptive media such as 1 M NaCl and 1 M urea were not able to desorb significant amounts of protein (data not shown). Figure S8 shows the effects of the combination of urea/citrate and citrate alone on the desorption of three different concentrations of the protein adsorbed onto 100 μg of Alhydrogel®. A combination of 1.8 M urea and 0.9 M citrate was required to desorb about 80% of the protein from the surface of the adjuvant.
Effect of selected stabilizers on adsorption of HAC1 on Alhydrogel® surface
The adsorption of HAC1 to the surface of Alhydrogel® may be affected by the presence of the excipients that were identified as stabilizers of the protein in solution. The percent protein adsorbed to the adjuvant did not change significantly in the presence of the stabilizers (see Figure S4). Additional excipients including Tween-80 and phosphate were tested as well (data not shown), and no significant loss in the adsorption of HAC1 to the adjuvant surface was found.
Effect of Alhydrogel adsorption on HAC1 stability
Intrinsic Trp fluorescence spectroscopy was used to analyze changes in the tertiary structure of the protein adsorbed to the surface of Alhydrogel®. At 10°C, the emission peak maximum for HAC1 in solution is at 332 nm, which indicates substantially buried Trp residues (). The emission peak maximum does not change until about 45°C, and slowly transitions to about 337 nm by 55°C. This transition at 45°C represents a surface-induced stability perturbation in the tertiary structure of HAC1, since the Tm of HAC1 in solution is about 54°C. When the protein is adsorbed to Alhydrogel, the steep transition near 54°C observed in solution is replaced by a very broad and gradual transition that actually initiates as low as 25°C (). In the presence of sorbitol, dextrose and sucrose, there is no significant stabilization of HAC1 on the adjuvant. Tween-80 and phosphate were also tested to see if they might help stabilize the adsorbed protein. shows the effect of the presence of 5 mM phosphate, 15% sorbitol and 0.1% Tween-80 as well as 5 mM phosphate alone on the stability of HAC1 adsorbed onto Alhydrogel®. At 10°C, the position of the emission peak maxima is similar to that of HAC1 in solution in both cases. The broad, gradual transitions, however, still exist. HAC1 in the presence of 15% sorbitol, 0.1% Tween-80 and 5 mM phosphate showed a thermal melting profile closest to that seen in solution. In this case, the transition starts at about 35°C with the Tm for the adsorbed protein found to be about 49°C. This represents a 5°C destabilization induced by the adsorption of HAC1 onto the surface of Alhydrogel®, compared with the 10°C destabilization described above.
Figure 6. Intrinsic fluorescence based melting curves of HAC1 in the presence of selected excipients and Alhydrogel®. The excipients shown here were selected by screening in liquid formulations.
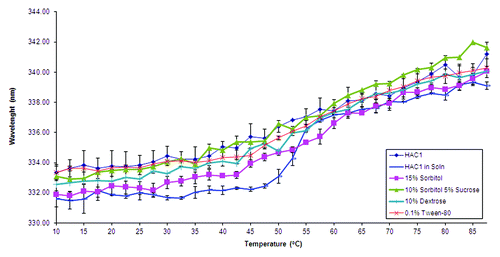
Figure 7. Intrinsic fluorescence based melting curves of HAC1 in the presence of best stabilizers identified for adjuvanted formulation. The error bars reflect the standard deviations based on three different experiments.
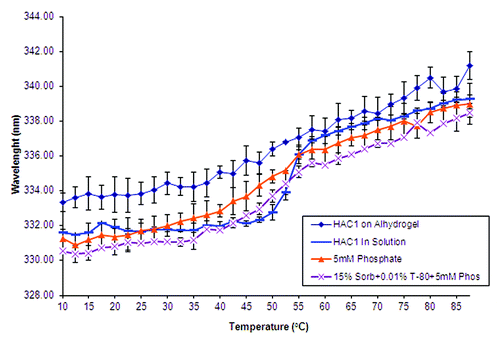
Heat treatment, or “annealing,” of the protein was undertaken for 10 min at 40, 50 or 60°C to see if this could produce a stabilization effect. The position of the emission peak maxima was found to be near 334 nm, which suggests a slight change in structure compared with the non-annealed protein (). When annealed at 40°C, however, the protein retains this conformation until about 40°C, with the position of the emission peak gradually increasing. The Tm for this transition was approximately 54°C, the same as seen for HAC1 in solution in the absence of stabilizers. The extent of this retention of the conformation is not seen when the protein was annealed at 50 or 60°C.
Figure 8. Intrinsic fluorescence based melting curves of annealed HAC1 in the presence of Alhydrogel®.
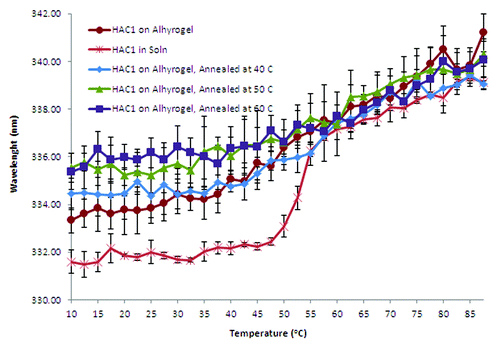
Of the formulations tested in this study, five were selected for further evaluation in animals based on their ability to best delay the transitions seen by intrinsic fluorescence. These formulations included the compounds, sorbitol, sucrose, phosphate and Tween-80. Fifteen percent sorbitol with 5mM phosphate and 0.1% tween-80 was used since it showed a profile closest to that seen in solution. HAC1 in the presence of 5mM phosphate was also used in the animal studies since the phosphate produced slight stabilization of the adsorbed protein. Finally, heat-treated HAC1 at 40°C was also included in animal studies to test if an un-stabilized protein might be more immunogenic.
Immunogenicity of the selected formulations in mice
An immunogenicity study in mice was conducted to evaluate effects of formulations on HAC1-elicited protective correlates of immunity. In the absence of Alhydrogel®, mice immunized with HAC1 formulations with 15% sorbitol or 10% dextrose (groups 1 and 3) showed higher serum hemagglutination-inhibition (HAI) antibody titers on study day 35 compared with the HAC1 formulation with 10% sorbitol and 5% sucrose (group 2, p < 0.001). In groups 1 and 3, mean HAI titers reached the values of 1:304 (± 138) and 1:154 (± 68), respectively, on study day 35, and 100% and 80% of the immunized animals, respectively, showed HAI titers of ≥ 1:40 (). These titers slightly decreased by study day 70 (). In group 3, the mean HAI titer was 1:26 (± 8) on study day 35 and only 40% of animals reached HAI titers of ≥ 1:40 (). In the presence of Alhydrogel® (groups 4–7), mean HAI titers were significantly higher than those observed in the groups immunized without Alhydrogel®, and were either maintained or slightly increased through study day 35 to 70 with a 100% seropositive rate (). No statistically significant difference was observed among the groups immunized with Alhydrogel®, except that in group 6 the mean HAI titer was lower than in groups 5 and 7 (p < 0.05) on study day 35.
Table 4. Mean HAI antibody titers and seropositive rates
Discussion
HAC1 is an H1N1 influenza vaccine candidate containing the recombinant HA protein from H1N1 influenza virus A/California/04/09 strain produced in a plant-based system. Only the ectodomain of HA is used in HAC1 with the last 77 residues that aid in anchoring the protein to the lipid membrane of the virus removed. In this study, we applied a composite biophysical approach to characterize HAC1 and identify stabilizers. The signals from various spectroscopic and light scattering techniques have been used to monitor changes in the physical structure of HAC1 when exposed to stresses such as pH and temperature. The CD spectra of HAC1 show predominantly α-helical structure, although there is an indication of the presence of some β-sheet character as well. CD secondary structural analysis using K2D2 showed that HAC1 contained 77% of α-helix and about 1% β-sheet.Citation16 This is consistent with the published crystal structure of HA.Citation17 Thermal transitions indicate a gradual loss in the secondary structure with increases in temperature at pH 3.0, 4.0, and 5.0. The CD thermal transition at pH 6.0 manifests a sudden change in the secondary structure at about 30°C, which is raised to about 45–50°C at pH 7.0 and 8.0. The intrinsic Trp fluorescence melts show emission peak maxima for HAC1 at about 344 nm at pH 3.0, 4.0, 5.0 and 6.0, and about 340 nm at pH 7.0 and 8.0, when analyzed using the MSM method, which overestimates the peak position by about 8–12 nm. This confirms that HAC1 is not completely structurally disrupted at any pH tested, but rather exists in a partially perturbed state, with its Trp residues relatively buried under all pH conditions examined. No cooperative transitions were detected at pH 3.0, 4.0 and 5.0. At pH 6.0, a thermal transition was observed at about ~40°C and this transition initiates at ~50°C for pH 7.0 and 8.0. Static light scattering and extrinsic ANS fluorescence also revealed similar trends in the protein’s thermal transitions. ANS was found to bind to HAC1 at low temperature at pH 3.0, 4.0, and 5.0 presumably due to the partially altered structure.
All the data collected for HAC1 was combined in the form of an EPD to better represent the effect of temperature and pH on the protein. The three distinct regions in the EPD presumably reflect the conformational changes that HA undergoes when influenza virus infects a cell.Citation18-Citation20 At physiological pH, the protein is in its native form. HA binds to sialic acid residues on the cell surface, which causes the host cell to internalize the virus into an endosomal compartment. When the pH of the endosome drops below 6.0, the conformation of HA alters, resulting in the exposure of apolar regions. This new form attaches itself to the endosomal membrane. As the pH further drops to about 5.0, HA again changes its structure causing the membrane of the virus to fuse with that of the endosome, propelling viral RNA into the cytoplasm. Thus, the conformational changes that occur at different pH values during natural virus infection appear to be reflected in the EPD.
Since HAC1 was found to be most stable at pH 7.0 and 8.0, pH 7.0 was chosen to screen for stabilizers and further formulation studies. Imidazole buffer was selected for the screening studies. Since phosphate can convert aluminum hydroxide into aluminum phosphate and citrate is known to dissolve the aluminum salt adjuvants, both of which can affect antigen binding properties, citrate phosphate buffer was not used for further studies. Surfactants such as Tween-80, sugars and sugar alcohols such as dextrose and sorbitol showed the highest degree of stabilization with respect to aggregation with an increase in Tm of ~7°C. Although Tween-80 stabilized the protein against aggregation, it did not significantly protect it against thermally induced conformational changes at concentrations of 0.05 - 0.1%. It has been suggested that polyalcohol and sugars delay or inhibit aggregation by enhancing the stability of native-like protein structures. Extensive studies have been reported on this kind of stabilization through nonspecific processes such as preferential hydration.Citation21-Citation23 The potential for stabilization via specific interactions, however, cannot be eliminated due to similarities in the chemical structure between some stabilizers and sialic acid which is a natural ligand for the HA protein. Destabilization and enhanced aggregation of HAC1 in the presence of a polyanion (dextran sulfate) is presumably due to the formation of HA-polyanion complexes. Tween-80 is known to often exert protective effects on protein aggregation through direct interaction with the protein or via its effect on air and water interfaces. In this case, however, the conformational destabilization caused by Tween-80 renders it potentially less useful in formulation development. Sorbitol, dextrose, and sucrose produced the highest increases in Tm values. Concentrations of 15% or below were selected for combination studies to minimize high osmolarity values.
When using aluminum salt adjuvants, the antigen is typically adsorbed onto the surface of the adjuvant. A high level of unbound protein adjuvanted with Alhydrogel® has been associated with hypersensitivity reactions as shown for some malaria antigens in clinical trials.Citation24-Citation26 Several ideas have been proposed over that past 80 y to explain the mechanism of immunopotentiation by aluminum salt adjuvants. The depot theory postulates that the aluminum adjuvant and the adsorbed antigen remain at the site of injection.Citation27 The slow release of antigen from the adjuvant stimulates antibody production. Another theory suggests that the adjuvant causes inflammation at the site of injection, attracting antigen presenting cells (APCs).Citation28 A third idea postulates that particulation of an antigen may occur on the surface of the adjuvant, which results in antigen internalization by macrophages via phagocytosis leading to an enhanced immune response. Recently, it was proposed that upon interaction of dendritic cells (DCs), the key APCs, with an adjuvant, DCs’ lipid membrane undergoes re-assortment which leads to the antigen being delivered in its soluble form into DC followed by processing in the MHC class II compartment, which ultimately shows a high preference for activation of CD4+ T-cells that in turn activate B-cells and increase an antibody response.Citation29 It is possible that some these mechanisms contribute to immunopotentiation by aluminum salt adjuvants. Recent studies have also suggested that in some cases retention of an antigen on an adjuvant is not essential for immunopotentiation.Citation30 Nevertheless, adsorbing the antigen onto the surface of the aluminum salt adjuvant is a common approach to produce an optimal immunogenic effect.
The PI of HAC1 is around 6.5. This gives the protein a negative charge at pH 7.0. Due to simple electrostatic interactions, HAC1 should adsorb onto positively charged Alhydrogel®. This is in a good agreement with the experimental data. Other interactions such as van der Waals forces may play an important role in the adsorption process as well. Here, almost all of the protein is found to be bound to Alhydrogel® within the first 2 min of introduction. The adsorption isotherm demonstrates a very high adsorption capacity for HAC1 with 100 μg of Alhydrogel® able to adsorb 160 μg of HAC1. The inability of NaCl to desorb the protein from the surface of Alhydrogel® suggests that the binding is not purely electrostatic in nature. The presence of strong desorptive agents such as a combination of urea and citrate resulted in desorption of about 80% of the protein from the surface of Alhydrogel®. The stability of the protein, however, was found to be decreased when adsorbed onto the surface of Alhydrogel®. Several studies have demonstrated that adsorption of a protein onto the surface of aluminum salts generally has a negative effect on the thermal stability of the protein.Citation31,Citation32 When the protein is heated, there is a gradual change in the position of the emission peak maximum, replacing the cooperative unfolding transition that occurs at about 45°C in solution with one 10°C less. This may be due to a time-dependent optimization of interactions between the protein and the surface of the adjuvant. In a few cases, it was possible to partially overcome this effect. This decreased stability may also lead to enhanced chemical degradation. Deamidation is especially a concern. This is due to the high pH in the microenvironment of the protein on the surface of Alhydrogel®. The positively charged surface of the adjuvant repels protons and attracts OH- ions, thereby increasing pH and making deamidation more likely to occur. The high surface pH can be countered by using sodium phosphate. Sodium phosphate can partially convert the positively charged surface of Alhydrogel® to aluminum phosphate lowering the surface pH. As seen is this study, sodium phosphate at a concentration of 5 mM was, in fact, able to stabilize the adsorbed protein to some extent. Actual studies of the chemical stability of HAC1 have not yet been conducted.
The effects of adding excipients and/or stabilizers on the immunogenicity of the HAC1 vaccine were also evaluated. The addition of 15% sorbitol or 10% dextrose resulted in the induction of higher serum HAI antibody titers in mice compared with the addition of 10% sorbitol and 5% sucrose. This suggests that sorbitol or dextrose may have a stabilizing effect, as described in the Results section, and improve the immunogenicity of the HAC1 vaccine. Serum HAI titers, however, gradually decreased from study day 35 through study day 70, while the HAI titers in mice immunized with HAC1 plus Alhydrogel® were maintained at same levels up to study day 70, perhaps due to a depot effect of the aluminum adjuvant. In previous studies,Citation33 we reported the ability of the plant-produced HAC1, at doses of 15 or 5 µg in saline plus Alhydrogel® (1:10 [w:w] of aluminum), to elicit HAI antibody titers ≥ 1:40 in 100% of immunized mice. In addition, immunization with 15 or 5 µg of HAC1 in the absence of Alhydrogel® elicited HAI antibody titers ≥ 1:40 in 83 or 50% of mice, respectively.33According to the FDA’s recommendation,Citation33 however, the amount of aluminum in a dose of a biological product should not exceed 1.14 mg, if determined by calculation on the basis of the amount of aluminum compound added. Therefore, in the current study, we investigated whether vaccine formulations containing a lower, more acceptable amount of aluminum or no aluminum adjuvant at all could still elicit significant antibody responses. The results obtained indicate that immunization with HAC1 plus a lower amount (0.5 mg/ml) of aluminum can still elicit significant HAI antibody responses with 100% of animals generating HAI antibody titers ≥ 1:40. Interestingly, in the absence of Alhydrogel, the addition of 15% sorbitol resulted in the generation of equal or greater HAI responses compared with those induced by HAC1 in saline without Alhydrogel® as reported on previously.Citation33 These results need to be further investigated in a dose-reduction study to determine how the buffer and excipients tested in the current study affected the robustness of the HAI antibody responses, particularly with regard to the lower amount of Alhydrogel® tested. In addition, no statistically significant differences in HAI titers were observed between the group of mice immunized with heat-treated HAC1 plus Alhydrogel® and the other HAC1/Alhydrogel® groups. Similar results were observed when total IgG titers elicited by the different immunizations were analyzed (data not shown). Although our results demonstrate that heat-induced structural changes did not affect the immunogenicity of HAC1 in terms of the magnitude of total IgG and HAI responses induced, comparison of virus-neutralization antibody titers between animals immunized with structurally altered HAC1 vs. non-denatured HAC1 would provide important additional information about the high temperatures have on the immunogenicity of HAC1. In summary, the results of this study demonstrate that the amount of Alhydrogel® in the HAC1 vaccine could be spared down to 0.5 mg/ml, the same amount of aluminum used in GARDASIL, the FDA-approved human papillomavirus vaccine (Merck and Co., Inc., Whitehouse Station, NJ).
Materials and Methods
Plant-derived HA, HAC1, was produced by Fraunhofer CMB Inc. (Newark, DE)Citation34 and supplied as a frozen solution. For initial characterization studies, citrate phosphate (CP) buffers (20 mM, pH 3.0–8.0) with an ionic strength of 0.15 (adjusted with NaCl) were used. Imidazole buffer (10 mM, pH 7.0) containing 150 mM NaCl was used for excipient screening and adjuvant studies. For buffer exchange, protein was dialyzed at 4°C using Slide-A-Lyzer® Dialysis Cassettes, 10 kDa MWCO (Pierce, Rockford, IL). Alhydrogel® (2%) was obtained from Brenntag Biosector, Frederickssund, Denmark. All other reagents were of analytical grade. Protein concentration was determined by UV absorbance at 280 nm using an extinction coefficient of 1.36 ml/mg-cm for HAC1. Experiments in the presence of the adjuvant were conducted by adding Alhydrogel® to the protein (or the protein-excipient mixture).
Characterization of the physical stability of HAC1
Sample preparation
For each of the spectroscopic techniques, duplicate samples were prepared at different pH values (pH 3.0 to 8.0, at one pH unit intervals) by dilution of the stock material with 20 mM CP buffer of the appropriate pH. The ionic strength of each sample was kept constant at a value of 0.15 using NaCl. Protein solutions were studied at a concentration of 0.2 mg/ml for CD studies and 0.1 mg/ml for fluorescence studies.
Far-UV circular dichroism (CD) spectroscopy
CD spectra were acquired using a Jasco J-810 spectropolarimeter equipped with a 6-position peltier temperature-controlled sample cell holder (Jasco Inc., Easton, MD). The CD spectra were recorded from 260–190 nm with a scanning speed of 50 nm/min, a response time of 2 sec and an accumulation of 3.To study thermal transitions (melting curves) of the proteins (in sealed cuvettes of 0.1 cm pathlength), the CD signal at 222 nm was monitored every 2.5°C with a 3 min equilibration over a 10 to 87.5°C temperature range employing a temperature ramp of 15°C/hr. A buffer baseline was subtracted from each spectrum.
Intrinsic tryptophan (Trp) fluorescence spectroscopy
Fluorescence spectra were acquired using a Photon Technology International (PTI) spectrofluorometer (Lawrenceville, NJ) equipped with a turreted 4-position peltier-controlled cell holder. An excitation wavelength of 295 nm was used to excite Trp residues and the emission spectra (> 95% Trp emission) were collected from 310 to 400 nm. Light scattering was monitored using a second detector located at 180° to the emission detector, and was collected at 295 nm. Emission spectra were collected every 2.5°C with a 3 min equilibration period at each temperature over the temperature range of 10 to 87.5°C in a 1 cm pathlength quartz cuvette. A buffer baseline was subtracted from each raw emission spectrum. Peak positions and intensities of the emission spectra were obtained using a mean spectral center of mass (MSM) method which increases the actual peak positions by about 8–10 nm, but yields a better signal to noise ratio and increased reproducibility.
ANS fluorescence spectroscopy
The accessibility of apolar sites on protein surfaces was monitored by fluorescence emission of the extrinsic probe 8-anilino-1-naphthalene sulfonate (ANS). Each sample contained an optimized 15-fold molar excess of ANS to protein. The ANS was excited at 375 nm and emission spectra were collected from 400–600 nm. Emission spectra were collected every 2.5°C with a 3 min equilibration period over a temperature range of 10 to 87.5°C. The ANS-buffer baseline at each corresponding pH was subtracted from raw emission spectra. Peak intensities of the emission spectra were obtained using the MSM method.
Empirical phase diagrams (EPDs)
EPDs were constructed as previously described.Citation35 CD molar ellipticity at 222 nm, intrinsic Trp fluorescence peak intensity and peak shift, light scattering intensity and ANS fluorescence peak intensity data sets were used for construction of the empirical phase diagram. All calculations were performed using Matlab software (The MathWorks, Natick, MA). Briefly, the experimental data sets were represented as n-dimensional vectors in n x n density matrices, where n is the number of variables (e.g., CD, fluorescence, light scattering, etc.) at each pH-temperature combination. The density matrices were used to derive n sets of eigenvalues and eigenvectors. The three eigenvectors which corresponded to the highest eigenvalues were employed to re-expand the data into three dimensions, and each vector was assigned a color (red, green or blue). The resulting multi-colored plot represents contributions of each vector at a given pH/temperature value. Thus, regions of the plot with similar color indicate similar physical states of the protein. A detailed explanation of the calculations involved in construction of EPDs is presented elsewhere.Citation36,Citation37
Excipient screening
Prior to excipient screening, an accelerated stability assay based on intrinsic fluorescence and light scattering (using thermal transitions) was employed to select an optimal buffer system at pH 7.0. After observing insignificant differences in protein stability in the buffers tested including PBS, imidazole and citrate-phosphate, imidazole was chosen as the buffer for further experiments. For excipient screening, duplicate samples were prepared at pH 7.0 in 10 mM imidazole buffer. The ionic strength of all samples was kept constant at a value of 0.15 using NaCl. Protein solutions were studied at a concentration of 0.1 mg/ml for these studies. The intrinsic fluorescence assay was further used to screen for stabilizing compounds. Thermal transition plots of the protein in the presence of various GRAS (Generally Regarded as Safe) excipients were acquired using the PTI spectrofluorometer as described above. The excipients that shift the thermal transitions to higher temperatures are defined as stabilizers, while those that shift the curves toward lower temperatures are considered destabilizers. Thus, the extent of stabilization and delay in aggregation of protein by the excipients were evaluated by their ability to shift the midpoint of the thermal transition temperature (Tm) of the protein to higher temperatures. The Tm values were obtained by normalizing the melting curve between 0 and 100 percent, i.e., the part of the curve before the transition initiates was set to 0, and the part of the curve after the transition is completed was set to 100. The temperature at which the melting curve crosses the 50% mark is then defined as the Tm.
Following identification of potential stabilizers, the concentration of the stabilizers was optimized. Once suitable concentrations of stabilizing compounds were obtained, the stabilizers were used in combinations to determine if any additive or synergistic effects could be obtained for stabilization of HAC1.
Adjuvant binding and stability studies
The adsorptive capacity of HAC1 in the presence of Alhydrogel® at various concentrations (0.04 – 0.36 mg/ml at 0.04 unit intervals) was determined by constructing a binding isotherm. The protein solutions in the presence of 0.2 mg/ml Alhydrogel® were tumbled in an end-over-end tube rotator at 4°C for 20 min in imidazole buffer at pH 7.0. The samples were centrifuged using an IEC Micromax centrifuge at 2,500 x g for 1 min to pellet the adjuvant. The amount of protein remaining in the supernatants (measured by UV absorbance spectroscopy) was used for the construction of binding curves. The ability of the protein to bind to Alhydrogel® in the presence of excipients was determined by the same procedure. In this case, Alhydrogel® was added to the protein-excipient solution.
Desorption of the protein from Alhydrogel® was monitored in the presence of several potential desorption agents including NaCl, guanidine hydrochloride, urea and sodium citrate. The protein-Alhydrogel® pellets were prepared as described above. The pellets were washed with imidazole buffer (pH 7.0) to remove any protein present in the supernatant prior to addition of the desorption solution. The Alhydrogel® solutions were then tumbled in an end-over-end tube rotator at 4°C for 20 min. The samples were centrifuged at 2,500 x g for 2 min to pellet the adjuvant. The amount of protein in the supernatant was used to measure the protein desorbed from the surface of the adjuvant.
The thermal stability of the protein bound to Alhydrogel® was monitored by intrinsic Trp fluorescence spectroscopy. Protein (180 μg/ml) was added to Alhydrogel® (0.5 mg/ml) by the procedure described above, which ensured > 90% bound protein. The solution was transferred into a 1 mm cuvette and the adjuvant was allowed to settle for 4 h. The use of a small pathlength cuvette enables us to obtain the fluorescence spectra of the protein while adsorbed to the adjuvant.Citation32 The pellet was then analyzed between 10 and 87.5°C. The Tm values were obtained from the melting curves by intrinsic fluorescence spectroscopy. The effect of excipients on the stability on the adjuvant-bound protein was also tested. When tested with excipients, the adjuvant was always added last to the protein-excipient mixture.
A heat treatment (“annealing”) of the protein was undertaken to see if this procedure offered any additional stability. Samples of the protein in the presence of the adjuvant were heated at 40, 50, or 60°C for 10 min or 2 h in a water bath. After the specified amount of time, they were allowed to cool to room temperature and then refrigerated at 4°C until further experiments. The stability of the bound protein was tested as described above using intrinsic fluorescence spectroscopy. The stability of the annealed protein was studied in the presence of selected excipients as well.
Immunogenicity of selected formulations of HAC1 in mice
Immunogenicity of selected formulations of HAC1 was evaluated in mice using HAI antibody response as a parameter. Groups of 6-week–old Balb/c mice, 5 mice per group, were immunized with 18 µg/dose of HAC1 intramuscularly (i.m.) at 3-week intervals on study days 0 and 21. The HAC1 formulations tested were prepared in the buffer containing 10 mM imidazole and 150 mM NaCl, pH 7, and included three formulations without Alhydrogel®, two formulations with Alhydrogel® (0.5 mg/ml of aluminum), and heat-treated (“annealed”) HAC1 with Alhydrogel® (to evaluate the effect of the heat treatment on the immunogenicity), as shown in . Animals in group 7 received HAC1 in saline with Alhydrogel® (1.8 mg/ml of aluminum), which has been previously shown to elicit robust HAI antibody responses in mice,Citation34 and group 8 received saline alone with 1.8 mg/ml of Alhydrogel® (a negative control group). Serum samples were collected prior to each immunization and at 2, 3 and 7 weeks after the second dose. Serum samples collected from immunized animals were first treated with a receptor-destroying enzyme (RDE; Denka Seiken Co. Ltd., Tokyo, Japan) and then tested in the HAI assay. The HAI assay was performed using 0.75% turkey erythrocytes as described previously,Citation38 with an initial serum dilution of 1:20 against the A/California/07/09 x 179A virus (CDC # 2009713114). Immunized animals with HAI antibody titers of ≥ 1:40 were defined as seropositive.
Table 3. Formulations tested in mouse immunogenicity study
Statistical analysis of data was performed using a two-tailed F-test with equal variance and significance was considered at a p value < 0.05. Mouse serum samples without detectable HAI were assigned a value of 10.
Summary
The empirical phase diagram approach was successfully used to characterize the plant-derived flu hemagglutinin as a function of pH and temperature. Intrinsic fluorescence spectroscopy was used to identify several excipients that stabilized the protein in solution and in the presence of Alhydrogel. Adsorption of the protein to Alhydrogel destabilized the protein conformationally, and additional excipients were identified to stabilize the adsorbed protein. Immunogenicity studies in mice revealed higher antibody titers against the protein in the presence of the adjuvant.
Additional material
Download Zip (582.4 KB)Disclosure of Potential Conflicts of Interest
No potential conflicts of interest were disclosed.
References
- Plotkin SA. O.W., Vaccines. 4 ed. 2004: Saunders.
- Gerdil C. The annual production cycle for influenza vaccine. Vaccine 2003; 21:1776 - 9; http://dx.doi.org/10.1016/S0264-410X(03)00071-9; PMID: 12686093
- Sheridan C. Next generation flu vaccine boosted by Chiron debacle. Nat Biotechnol 2004; 22:1487 - 8; http://dx.doi.org/10.1038/nbt1204-1487; PMID: 15583640
- Stephenson I, Nicholson KG, Wood JM, Zambon MC, Katz JM. Confronting the avian influenza threat: vaccine development for a potential pandemic. Lancet Infect Dis 2004; 4:499 - 509; http://dx.doi.org/10.1016/S1473-3099(04)01105-3; PMID: 15288823
- Wang K, Holtz KM, Anderson K, Chubet R, Mahmoud W, Cox MM. Expression and purification of an influenza hemagglutinin--one step closer to a recombinant protein-based influenza vaccine. Vaccine 2006; 24:2176 - 85; http://dx.doi.org/10.1016/j.vaccine.2005.11.005; PMID: 16310896
- Delaney JW, Fowler RA. 2009 influenza A (H1N1): a clinical review. Hosp Pract (Minneap) 2010; 38:74 - 81; PMID: 20469616
- Maritz J, Maree L, Preiser W. Pandemic influenza A (H1N1) 2009: the experience of the first six months. Clin Chem Lab Med 2010; 48:11 - 21; http://dx.doi.org/10.1515/CCLM.2010.023; PMID: 20025556
- Cox MM, Hollister JR. FluBlok, a next generation influenza vaccine manufactured in insect cells. Biologicals 2009; 37:182 - 9; http://dx.doi.org/10.1016/j.biologicals.2009.02.014; PMID: 19297194
- Cox MM, Patriarca PA, Treanor J. FluBlok, a recombinant hemagglutinin influenza vaccine. Influenza Other Respi Viruses 2008; 2:211 - 9; http://dx.doi.org/10.1111/j.1750-2659.2008.00053.x; PMID: 19453397
- Kissmann J, Joshi SB, Haynes JR, Dokken L, Richardson C, Middaugh CR. H1N1 influenza virus-like particles: physical degradation pathways and identification of stabilizers. J Pharm Sci 2011; 100:634 - 45; http://dx.doi.org/10.1002/jps.22304; PMID: 20669328
- McPherson CE. Development of a novel recombinant influenza vaccine in insect cells. Biologicals 2008; 36:350 - 3; http://dx.doi.org/10.1016/j.biologicals.2008.08.001; PMID: 18804387
- Robertson JS, Naeve CW, Webster RG, Bootman JS, Newman R, Schild GC. Alterations in the hemagglutinin associated with adaptation of influenza B virus to growth in eggs. Virology 1985; 143:166 - 74; http://dx.doi.org/10.1016/0042-6822(85)90105-9; PMID: 4060580
- Schild GC, Oxford JS, de Jong JC, Webster RG. Evidence for host-cell selection of influenza virus antigenic variants. Nature 1983; 303:706 - 9; http://dx.doi.org/10.1038/303706a0; PMID: 6190093
- Yusibov V, Streatfield SJ, Kushnir N. Clinical development of plant-produced recombinant pharmaceuticals: vaccines, antibodies and beyond. Hum Vaccin 2011; 7:313 - 21; http://dx.doi.org/10.4161/hv.7.3.14207; PMID: 21346417
- Musiychuk K, Stephenson N, Bi H, Farrance CE, Orozovic G, Brodelius M, et al. A launch vector for the production of vaccine antigens in plants. Influenza Other Respi Viruses 2007; 1:19 - 25; http://dx.doi.org/10.1111/j.1750-2659.2006.00005.x; PMID: 19453476
- Perez-Iratxeta C, Andrade-Navarro MA. K2D2: estimation of protein secondary structure from circular dichroism spectra. BMC Struct Biol 2008; 8:25; http://dx.doi.org/10.1186/1472-6807-8-25; PMID: 18477405
- Lin T, Wang G, Li A, Zhang Q, Wu C, Zhang R, et al. The hemagglutinin structure of an avian H1N1 influenza A virus. Virology 2009; 392:73 - 81; http://dx.doi.org/10.1016/j.virol.2009.06.028; PMID: 19628241
- Cross KJ, Langley WA, Russell RJ, Skehel JJ, Steinhauer DA. Composition and functions of the influenza fusion peptide. Protein Pept Lett 2009; 16:766 - 78; http://dx.doi.org/10.2174/092986609788681715; PMID: 19601906
- Harrison SC. Mechanism of membrane fusion by viral envelope proteins. Adv Virus Res 2005; 64:231 - 61; http://dx.doi.org/10.1016/S0065-3527(05)64007-9; PMID: 16139596
- Harrison SC. Viral membrane fusion. Nat Struct Mol Biol 2008; 15:690 - 8; http://dx.doi.org/10.1038/nsmb.1456; PMID: 18596815
- Shimizu S, Smith DJ. Preferential hydration and the exclusion of cosolvents from protein surfaces. J Chem Phys 2004; 121:1148 - 54; http://dx.doi.org/10.1063/1.1759615; PMID: 15260652
- Uedaira H, Uedaira H. Role of hydration of polyhydroxy compounds in biological systems. Cell Mol Biol (Noisy-le-grand) 2001; 47:823 - 9; PMID: 11728096
- Lee JC, Timasheff SN. The stabilization of proteins by sucrose. J Biol Chem 1981; 256:7193 - 201; PMID: 7251592
- Edelman R, Wasserman SS, Kublin JG, Bodison SA, Nardin EH, Oliveira GA, et al. Immediate-type hypersensitivity and other clinical reactions in volunteers immunized with a synthetic multi-antigen peptide vaccine (PfCS-MAP1NYU) against Plasmodium falciparum sporozoites. Vaccine 2002; 21:269 - 80; http://dx.doi.org/10.1016/S0264-410X(02)00468-1; PMID: 12450702
- Keitel WA, Kester KE, Atmar RL, White AC, Bond NH, Holland CA, et al. Phase I trial of two recombinant vaccines containing the 19kd carboxy terminal fragment of Plasmodium falciparum merozoite surface protein 1 (msp-1(19)) and T helper epitopes of tetanus toxoid. Vaccine 1999; 18:531 - 9; http://dx.doi.org/10.1016/S0264-410X(99)00221-2; PMID: 10519944
- Kaslow DC. Transmission-blocking vaccines. Chem Immunol 2002; 80:287 - 307; http://dx.doi.org/10.1159/000058850; PMID: 12058646
- Glenny AT, Buttle GAH, Stevens MF. Rate of disappearance of diphtheria toxoid injected into rabbits and guinea - pigs: Toxoid precipitated with alum. J Pathol Bacteriol 1931; 34:267 - 75; http://dx.doi.org/10.1002/path.1700340214
- McKee AS, Munks MW, MacLeod MK, Fleenor CJ, Van Rooijen N, Kappler JW, et al. Alum induces innate immune responses through macrophage and mast cell sensors, but these sensors are not required for alum to act as an adjuvant for specific immunity. J Immunol 2009; 183:4403 - 14; http://dx.doi.org/10.4049/jimmunol.0900164; PMID: 19734227
- Flach TL, Ng G, Hari A, Desrosiers MD, Zhang P, Ward SM, et al. Alum interaction with dendritic cell membrane lipids is essential for its adjuvanticity. Nat Med 2011; 17:479 - 87; http://dx.doi.org/10.1038/nm.2306; PMID: 21399646
- Noe SM, Green MA, HogenEsch H, Hem SL. Mechanism of immunopotentiation by aluminum-containing adjuvants elucidated by the relationship between antigen retention at the inoculation site and the immune response. Vaccine 2010; 28:3588 - 94; http://dx.doi.org/10.1016/j.vaccine.2010.02.085; PMID: 20211692
- Jones LS, Peek LJ, Power J, Markham A, Yazzie B, Middaugh CR. Effects of adsorption to aluminum salt adjuvants on the structure and stability of model protein antigens. J Biol Chem 2005; 280:13406 - 14; http://dx.doi.org/10.1074/jbc.M500687200; PMID: 15684430
- Peek LJ, Martin TT, Elk Nation C, Pegram SA, Middaugh CR. Effects of stabilizers on the destabilization of proteins upon adsorption to aluminum salt adjuvants. J Pharm Sci 2007; 96:547 - 57; http://dx.doi.org/10.1002/jps.20762; PMID: 17080408
- FDA. U.S. CFR - Code of Federal Regulations Title 21. [cited 2011 December 9]; Available from: http://www.accessdata.fda.gov/scripts/cdrh/cfdocs/cfcfr/CFRSearch.cfm?fr=610.15.
- Shoji Y, Chichester JA, Jones M, Manceva SD, Damon E, Mett V, et al. Plant-based rapid production of recombinant subunit hemagglutinin vaccines targeting H1N1 and H5N1 influenza. Hum Vaccin 2011; 7:Suppl 41 - 50; http://dx.doi.org/10.4161/hv.7.0.14561; PMID: 21266846
- Fan H, Ralston J, Dibiase M, Faulkner E, Middaugh CR. Solution behavior of IFN-beta-1a: an empirical phase diagram based approach. J Pharm Sci 2005; 94:1893 - 911; http://dx.doi.org/10.1002/jps.20410; PMID: 16052555
- Kueltzo LA, Ersoy B, Ralston JP, Middaugh CR. Derivative absorbance spectroscopy and protein phase diagrams as tools for comprehensive protein characterization: a bGCSF case study. J Pharm Sci 2003; 92:1805 - 20; http://dx.doi.org/10.1002/jps.10439; PMID: 12949999
- Maddux NR, Joshi SB, Volkin DB, Ralston JP, Middaugh CR. Multidimensional methods for the formulation of biopharmaceuticals and vaccines. J Pharm Sci 2011; http://dx.doi.org/10.1002/jps.22618; PMID: 21647886
- Shoji Y, Chichester JA, Bi H, Musiychuk K, de la Rosa P, Goldschmidt L, et al. Plant-expressed HA as a seasonal influenza vaccine candidate. Vaccine 2008; 26:2930 - 4; http://dx.doi.org/10.1016/j.vaccine.2008.03.045; PMID: 18440103